Exploring nanofibrous self-assembling peptide hydrogels using mouse myoblast cells for three-dimensional bioprinting and tissue engineering applications
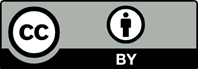
Injured skeletal muscles which lose more than 20% of their volume, known as volumetric muscle loss, can no longer regenerate cells through self-healing. The traditional solution for recovery is through regenerative therapy. As the technology of three-dimensional (3D) bioprinting continues to advance, a new approach for tissue transplantation is using biocompatible materials arranged in 3D scaffolds for muscle repair. Ultrashort self-assembling peptide hydrogels compete as a potential biomaterial for muscle tissue formation due to their biocompatibility. In this study, two sequences of ultrashort peptides were analyzed with muscle myoblast cells (C2C12) for cell viability, cell proliferation, and differentiation in 3D cell culture. The peptides were then extruded through a custom-designed robotic 3D bioprinter to create cell-laden 3D structures. These constructs were also analyzed for cell viability through live/dead assay. Results showed that 3D bioprinted structures of peptide hydrogels could be used as tissue platforms for myotube formation – a process necessary for muscle repair.
1. Choi YJ, Jun YJ, Kim DY, et al., 2019, A 3D Cell Printed Muscle Construct with Tissue-derived Bioink for the Treatment of Volumetric Muscle Loss. Biomaterials, 206:160-9. DOI 10.1016/j.biomaterials.2019.03.036.
2. Kim JH, Seol YJ, Ko IK, et al., 2018, 3D Bioprinted Human Skeletal Muscle Constructs for Muscle Function Restoration. Scientific Reports, 8:12307. DOI 10.1038/s41598-018- 29968-5.
3. VanDusen KW, Syverud BC, Williams ML, et al., 2014, Engineered Skeletal Muscle Units for Repair of Volumetric Muscle Loss in the Tibialis Anterior Muscle of a Rat. Tissue Engineering Part A, 20(21-22):2920-30. DOI 10.1089/ten.tea.2014.0060.
4. Chua CK, Yeong WY, 2014, Bioprinting: Principles and Applications. Vol. 1. Singapore: World Scientific Publishing Co, Inc.
5. Kwee BJ, Mooney DJ, 2017, Biomaterials for Skeletal Muscle Tissue Engineering. Current Opinion in Biotechnology, 47:16-22. DOI 10.1016/j.copbio.2017.05.003.
6. Frontera WR, Ochala J, 2015, Skeletal muscle: A Brief Review of Structure and Function. Calcified Tissue International, 96(3):183-95. DOI 10.1007/s00223-014-9915-y.
7. Beldjilali-Labro M, Garcia AG, Farhat F, et al., 2018, Biomaterials in Tendon and Skeletal Muscle Tissue Engineering: Current Trends and Challenges. Materials, 11(7):1116. DOI 10.3390/ma11071116.
8. Relaix F, Zammit PS, 2012, Satellite Cells are Essential for Skeletal Muscle Regeneration: The Cell on the Edge Returns Centre Stage. Development, 139(16):2845-56. DOI 10.1242/dev.069088.
9. Brack AS, Rando TA, 2012, Tissue-specific Stem Cells: Lessons from the Skeletal Muscle Satellite Cell. Cell Stem Cell, 10(5):504-14. DOI 10.1016/j.stem.2012.04.001.
10. Grogan BF, Hsu JR, Consortium STR, 2011, Volumetric Muscle Loss. JAAOS Journal of the American Academy of Orthopaedic Surgeons, 19:S35-7. DOI 10.5435/00124635- 201102001-00007.
11. Turner NJ, Badylak SF, Regeneration of Skeletal Muscle. Cell and Tissue Research, 347(3):759-74. DOI 10.1007/ s00441-011-1185-7.
12. Lynch GS, Schertzer JD, Ryall JG, 2008, Anabolic Agents for Improving Muscle Regeneration and Function After Injury. Clinical and Experimental Pharmacology and Physiology, 35(7):852-58. DOI 10.1111/j.1440-1681.2008.04955.x.
13. Järvinen TA, Järvinen TL, Kääriäinen M, et al., 2007, Muscle Injuries: Optimising Recovery. Best Practice and Research Clinical Rheumatology, 21(2):317-31. DOI 10.1016/j. berh.2006.12.004.
14. Järvinen TA, Järvinen TL, Kääriäinen M, et al., 2005, Muscle Injuries: Biology and Treatment. The American Journal of Sports Medicine, 33(5):745-64. DOI 10.1177/0363546505274714.
15. Järvinen TA, Kääriäinen M, Järvinen M, Kalimo H, 2000, Muscle Strain Injuries. Current Opinion in Rheumatology, 12(2):155-61. DOI 10.1097/00002281-200003000-00010.
16. Corona BT, Wu X, Ward CL, et al., 2013, The Promotion of a Functional Fibrosis in Skeletal Muscle with Volumetric Muscle Loss Injury following the Transplantation of muscle- ECM. Biomaterials, 34(13):3324-35. DOI 10.1016/j. biomaterials.2013.01.061.
17. Manring H, Abreu E, Brotto L, et al., 2014, Novel Excitation-contraction Coupling Related Genes Reveal Aspects of Muscle Weakness Beyond Atrophy new Hopes for Treatment of Musculoskeletal Diseases. Frontiers in Physiology, 5:37. DOI 10.3389/fphys.2014.00037.
18. Grasman JM, Zayas MJ, Page RL, et al., 2015, Biomimetic Scaffolds for Regeneration of Volumetric Muscle Loss in Skeletal Muscle Injuries. Acta Biomaterialia, 25:2-15. DOI 10.1016/j.actbio.2015.07.038.
19. Mase VJ, Hsu JR, Wolf SE, et al., 2010, Clinical Application of an Acellular Biologic Scaffold for Surgical Repair of a Large, Traumatic Quadriceps Femoris Muscle Defect. Orthopedics, 33(7):511. DOI 10.3928/01477447-20100526-24.
20. Zorlutuna P, Annabi N, Camci-Unal G, et al., 2012, Microfabricated Biomaterials for Engineering 3D Tissues. Advanced Materials, 24(14):1782-804. DOI 10.1002/ adma.201104631.
21. Hauser CA, Zhang S, 2010, Designer Self-assembling Peptide Nanofiber Biological Materials. Chemical Society Reviews, 39(8):2780-90. DOI 10.1039/b921448h.
22. Loo Y, Zhang S, Hauser CA, 2012, From Short Peptides to Nanofibers to Macromolecular Assemblies in Biomedicine. Biotechnology Advances, 30(3):593-603. DOI 10.1016/j. biotechadv.2011.10.004.
23. Wu EC, Zhang S, Hauser CA, 2012, Self-assembling Peptides as Cell-interactive Scaffolds. Advanced Functional Materials, 22(3):456-68. DOI 10.1002/adfm.201101905.
24. Hauser CA, Deng R, Mishra A, et al., 2011, Natural Tri-to Hexapeptides Self-assemble in Water to Amyloid β-type Fiber Aggregates by Unexpected α-helical Intermediate Structures. Proceedings of the National Academy of Sciences, 108(4):1361-6. DOI 10.1073/pnas.1014796108.
25. Mishra A, Loo Y, Deng R, et al., 2011, Ultrasmall Natural Peptides Self-assemble to Strong Temperature-resistant Helical Fibers in Scaffolds Suitable for Tissue Engineering. Nano Today, 6(3):232-9. DOI 10.1016/j.nantod.2011.06.010.
26. Pollot BE, Rathbone CR, Wenke JC, et al., 2018, Natural Polymeric Hydrogel Evaluation for Skeletal Muscle Tissue Engineering. Journal of Biomedical Materials Research Part B: Applied Biomaterials, 106(2):672-9. DOI 10.1002/jbm.b.33859.
27. Kahin K, Khan Z, Albagami M, et al., 2019, Development of a Robotic 3D Bioprinting and Microfluidic Pumping System for Tissue and Organ Engineering. Microfluidics, Biomems, and Medical Microsystems, Doi 10.1117/12.2507237.
28. Khan Z, Kahin K, Rauf S, et al., 2018, Optimization of a 3D Bioprinting Process Using Ultrashort Peptide Bioinks. International Journal of Bioprinting, 5(1):1-3. DOI 10.18063/ ijb.v5i1.173.
29. Brenneisen P, Blaudschun R, Gille J, et al., 2003, Essential role of an Activator Protein-2 (AP-2)/Specificity Protein 1 (Sp1) Cluster in the UVB-mediated Induction of the Human Vascular Endothelial Growth Factor in HaCaT Keratinocytes. Biochemical Journal, 369(2):341-9. DOI 10.1042/bj20021032.
30. Hendriks J, Riesle J, van Blitterswijk CA, 2007, Co-culture in Cartilage Tissue Engineering. Journal of Tissue Engineering and Regenerative Medicine, 1(3):170-8. DOI 10.1002/term.19.
31. Loo Y, Lakshmanan A, Ni M, et al., 2015, Peptide Bioink: Self-assembling Nanofibrous Scaffolds for Three-dimensional Organotypic Cultures. Nano Letters, 15(10):6919-25. DOI 10.1021/acs.nanolett.5b02859.
32. Jayawarna V, Ali M, Jowitt TA, et al., 2006, Nanostructured Hydrogels for Three-dimensional Cell Culture Through Self-assembly of Fluorenylmethoxycarbonyl Dipeptides. Advanced Materials, 18(5):611-4. DOI 10.1002/adma.200501522.
33. Arab W, Rauf S, Al-Harbi O, et al., 2018, Novel Ultrashort Self-Assembling Peptide Bioinks for 3D Culture of Muscle Myoblast Cells. International Journal of Bioprinting, 4(2):129. DOI 10.18063/ijb.v4i1.129.
34. Lakshmanan A, Cheong DW, Accardo A, et al., 2013, Aliphatic Peptides Show Similar Self-assembly to Amyloid Core Sequences, Challenging the Importance of Aromatic Interactions in Amyloidosis. Proceedings of the National Academy of Sciences, 110(2):519-24. DOI 10.1073/ pnas.1217742110.
35. Chen H, Zhong J, Wang J, et al., 2019, Enhanced Growth and Differentiation of Myoblast Cells Grown on E-jet 3D Printed Platforms. International Journal of Nanomedicine, 14:937-50. DOI 10.2147/ijn.s193624.