Zebrafish as a Model for Obesity and Diabetes
- 1Department of Molecular Physiology and Biophysics, Vanderbilt University School of Medicine, Nashville, TN, United States
- 2Graduate School of Regional Innovation Studies, Mie University, Tsu, Japan
- 3Center for Reproductive Biology, Washington State University, Pullman, WA, United States
Obesity and diabetes now considered global epidemics. The prevalence rates of diabetes are increasing in parallel with the rates of obesity and the strong connection between these two diseases has been coined as “diabesity.” The health risks of overweight or obesity include Type 2 diabetes mellitus (T2DM), coronary heart disease and cancer of numerous organs. Both obesity and diabetes are complex diseases that involve the interaction of genetics and environmental factors. The underlying pathogenesis of obesity and diabetes are not well understood and further research is needed for pharmacological and surgical management. Consequently, the use of animal models of obesity and/or diabetes is important for both improving the understanding of these diseases and to identify and develop effective treatments. Zebrafish is an attractive model system for studying metabolic diseases because of the functional conservation in lipid metabolism, adipose biology, pancreas structure, and glucose homeostasis. It is also suited for identification of novel targets associated with the risk and treatment of obesity and diabetes in humans. In this review, we highlight studies using zebrafish to model metabolic diseases, and discuss the advantages and disadvantages of studying pathologies associated with obesity and diabetes in zebrafish.
Introduction
The prevalence of overweight and obesity has steadily increased worldwide in the past several decades. In 2016, more than 1.9 billion adults were overweight, and of these over 650 million were obese (WHO, 2017). This is primarily due to excess food consumption (Vandevijvere et al., 2015). Overweight and obesity are major risk factors for numerous chronic diseases, including cardiovascular diseases, diabetes, and certain types of cancer (Haslam and James, 2005). In the United States, class III obese individuals (BMI ≥ 40 kg/m2) have a six-fold increase in diabetes risk over normal-weight individuals (Leung et al., 2017) and more than 90% of people with type 2 diabetes mellitus (T2DM) are overweight or obese. The global increase of overweight and obesity largely explains the incidence and prevalence of type 2 diabetes over the past 20 years. Obesity and T2DM can substantially decrease life expectancy, diminish quality of life, and impose a large economic burden to society (Leung et al., 2017).
Both obesity and T2DM have high heritability (Poulsen et al., 2001; Willemsen et al., 2015). Recent genome wide association studies and whole exome sequencing studies have identified a large number of genetic variants that are associated with overweight/obesity and/or T2DM (Lawlor et al., 2017; Loos, 2018). In most cases, however, the causative genes for these linked variants are uncertain and the mechanism by which these variants contribute to the disease phenotypes is unclear (Loos, 2018). Furthermore, the aggregate effect of all the variants only account for a small fraction of the heritability of these conditions (Fuchsberger et al., 2016). It is likely that more alleles are yet to be discovered to play a role in obesity and T2DM susceptibility.
The wealth of human genetic and epidemiological data on obesity and T2DM provides ample opportunity for mechanistic investigations in animal models. Zebrafish is a well-established model system for developmental biology, human genetics, and human diseases (Dooley and Zon, 2000; Gibert et al., 2013; Freifeld et al., 2017). Several features have propelled zebrafish to its current prominence in developmental biology and disease modeling. It is a vertebrate, having high degree of genetic, anatomical, and physiological similarities to humans. It is fecund, easy to maintain in large number and has a relative short generation time, allowing facile genetic, and chemical genetic screens (Kimmel et al., 1995; MacRae and Peterson, 2015). Its external development affords easy accessibility to embryonic and genetic manipulations (Kimura et al., 2014; Hoshijima et al., 2016; Yin et al., 2016). The optical transparency of its embryos permits time lapse live imaging (Hall et al., 2009; Herrgen et al., 2009; Feierstein et al., 2015). Although traditionally used for developmental biology, zebrafish has recently been used to investigate metabolic diseases. Here, we will review some of the recent studies using zebrafish to model human metabolic diseases, with an emphasis on obesity, and diabetes. We will discuss the advantages and disadvantages of studying pathologies associated with obesity and diabetes in zebrafish.
Zebrafish Obesity Models
Lipid Metabolism and Adipose Biology in Zebrafish
Obesity is a consequence of positive energy balance. Regulation of energy intake and expenditure involves many organ systems including the brain, intestines, skeletal muscle, and adipose tissue (Cai, 2013; Dailey, 2014; Periasamy et al., 2017). Therefore, whole animal models are essential for better understanding of the development and progression of metabolic dysfunction. Zebrafish is an excellent model in which to study metabolic dysfunction because they have the key organs that are important for regulation of energy homeostasis and metabolism in mammals, including digestive organs, adipose tissues, and skeletal muscle (Lieschke and Currie, 2007; Schlegel and Stainier, 2007). The key functions such as appetite regulation, insulin regulation and lipid storage are also well conserved (Elo et al., 2007; Flynn et al., 2009; Nishio et al., 2012). Similar to mammals, excess nutrients in zebrafish cause increased plasma triglyceride levels and hepatic steatosis (Oka et al., 2010). Obese zebrafish also exhibit dysregulation of pathways that control lipid metabolism, including SREBF1, PPARs, NR1H3, and LEP (Oka et al., 2010). The conservation of these metabolic pathways that play key roles in adipocyte differentiation, energy homeostasis (Den Broeder et al., 2015), and cholesterol metabolism (Schlombs et al., 2003) demonstrates zebrafish as a suitable model for human lipid metabolism. However, zebrafish is an ectotherm species and its metabolic rate is not regulated by environmental temperature. Consistent with this, zebrafish does not have brown adipocyte tissues (BAT).
A primary characteristic of obesity is adipose hypertrophy and hyperplasia. Zebrafish have multiple adipose tissue depots and their development has been characterized (Flynn et al., 2009). Neutral lipid droplets first appear in visceral adipocytes and accumulate as zebrafish grow. Similar to mammalian white adipose tissue (WAT), early-stage zebrafish adipocytes contain multiple small lipid droplets while mature zebrafish adipocytes have a single large lipid droplet. As occurs in mammals, the adipocyte lineage expresses pparg, and fabp4 (Flynn et al., 2009). Visceral adiposity is a critical risk factor for T2DM and other metabolic diseases (Ahima and Lazar, 2013). In zebrafish, like in mammals, lipids are stored in visceral, intramuscular and subcutaneous adipocyte depots (Song and Cone, 2007), providing the opportunity to understand the regulation of body fat distribution. The high degree of conservation in distribution and formation of adipose tissue in the zebrafish compared to mammals makes it an appropriate model to study obesity.
Methods to Quantitate Adiposity in Zebrafish
Quantitative measures of adiposity are important to assess the degree of obesity-related metabolic derangements. Body mass index (BMI) and quantitative computed tomography (CT) are widely used measurements of adiposity in humans but are more difficult to apply in zebrafish. Commonly used lipophilic dyes for visualizing lipids in histological sections and cultured cells, including Nile red, Oil red O, and Sudan black B, have been utilized to detect lipids in adult zebrafish sections and fixed zebrafish larvae (Marza et al., 2005; Schlegel and Stainier, 2006). With the optical transparency of zebrafish larvae, live-imaging, and fluorescence based screens have been developed for the study of digestive physiology or lipid metabolism. In particular, Nile red has been used for live imaging and quantification of intracellular neutral lipid droplets (Greenspan et al., 1985) as well as for purification of adipocyte tissues (Jones et al., 2008; Flynn et al., 2009; Oka et al., 2010). In addition, a variety of fluorescent lipid analogs and tracers are available, including BODIPY Fatty Acid Analogs, BODIPY-cholesterol analogs and fluorescence reports like PED6, for tracking the metabolism and distribution of exogenous lipids in live zebrafish (Hölttä-Vuori et al., 2010; Anderson et al., 2011). 3D micro-CT is also available for this small animal and allows volume measurement of total adipocyte tissue as well as different fat depots (Hasumura et al., 2012; Figure 1). Recently, Landgraf et al. compared the methodology of quantify zebrafish body fat mass using MR images (MRI) and EchoMRI 4in1 (EchoMRI™; Landgraf et al., 2017). The body fat mass of 8 adult male zebrafish was measured using the two methods and the two techniques showed high correlation. Overall, these methods provide accurate measurements of zebrafish adiposity and provide means for longitudinal monitoring.
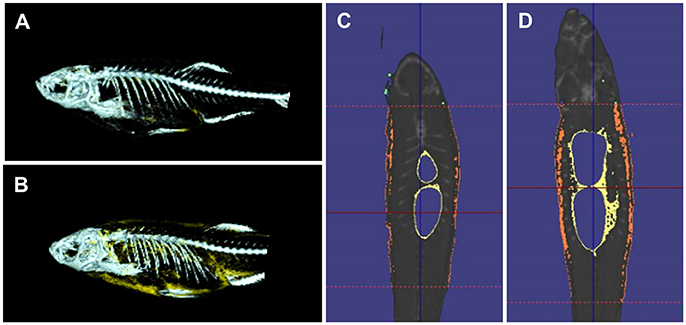
Figure 1. 3D micro-CT analysis in normal fed and diet-included obese zebrafish. (A) 3D-images of normal fed zebrafish. Gray color indicates skeleton and yellow color means adipocyte tissue. (B) 3D-images obese zebrafish. (C) Cross-sectional images of normal fed zebrafish. Yelloe color indicates visceral adipose tissue and orange color indicates subcutaneous adipocyte tissue. (D) Cross-sectional images of obese zebrafish.
A number of obesity models have been developed in zebrafish using diet and genetic manipulations (Table 1). The next section describes the details of these models.
High-Fat Diet/Over-Nutrition Induced Obesity
A common approach to induce obesity is excess fat intake. Obese zebrafish can be conveniently produced by overfeeding starting from the onset of feeding at 5 dpf (day post fertilization). This is advantageous over rodent models since diet can only be manipulated after weaning, which is at least 3 weeks after birth. Although early larvae have no WAT (Imrie and Sadler, 2010), lipid droplets accumulate in the blood stream and other measures such as whole-larval triacylglycerol level may be used as an indicator for quantifying obesity progression (Schlegel and Stainier, 2006; Tingaud-Sequeira et al., 2011). Although heavy cream has been used (Schlegel and Stainier, 2006), chicken egg yolk solution is the most used high-fat diet for zebrafish larvae and juveniles (Tingaud-Sequeira et al., 2011; Zhou et al., 2015; Kopp et al., 2016; den Broeder et al., 2017). The high fat diet rapidly increases zebrafish adiposity.
Adult zebrafish have also been used as an obesity model. The first diet-induced obese (DIO) zebrafish model was reported in 2010 (Oka et al., 2010) where adult fish (3.5 months of age) were fed with 60 mg or 5 mg of freshly hatched Artemia per day for 8 weeks (150 calories vs. 20 calories). The overfed zebrafish exhibited increased BMI, hypertriglyceridemia and hepatosteatosis compared to the normal fed zebrafish. Male and female zebrafish showed similar responses to over-nutrition treatment. Furthermore, comparative transcriptome analysis of visceral adipose tissue among zebrafish, mouse, rat and human revealed that lipid metabolism networks of zebrafish are similar to those of mammals.
Besides overfeeding with Artemia, several other methods have been used to generate obese zebrafish. Meguro et al. developed custom high-fat zebrafish diets containing 20% corn oil or lard. They demonstrated that these high-fat diets make zebrafish obese (Meguro et al., 2015). Similarly, overfeeding the combination of a commercial tropical fish flakes (Tetramin) and 20% crude vegetable oil to zebrafish for 256 days triggers cardiovascular overload (Vargas and Vásquez, 2017).
It is interesting to note that obesity induced by overfeeding normal fat diet differs from that induced by high fat diet. Landgraf et al. compared the metabolic phenotype of obesity induced by overfeeding of a normal fat diet (NFD; Artemia cysts, 22% fat) to that by high fat diet (HFD; egg yolk powder, 59% fat). Although both increase adiposity, fish with NFD-induced obesity are metabolically healthy. In contrast, fish with HFD-induced obesity are metabolically unhealthy, with glucose intolerance, fatty liver, and preferential increase of visceral fat (Landgraf et al., 2017). This is consistent with the “obesity paradox” observed in humans. Overweight and obesity are not always associated with insulin resistance, the major driver and precursor of T2DM. In fact, overweight has also been paradoxically associated with lower mortality (Kokkinos et al., 2009; Flegal et al., 2013). This is thought to be due to the difference in the fat distribution pattern. In general, the visceral fat mass is a better predictor for insulin resistance and T2DM than BMI (Lebovitz and Banerji, 2005; InterAct et al., 2012). Therefore, the pathophysiological consequences of different fat depots are also conserved in zebrafish.
Genetic Models of Obesity
Obesity is a complex disease that results of an interaction between genetic and environmental factors. Genetically-modified animal models that reflect human obesity pathology are needed for understanding the physiological and genetic basis of obesity and for the development of pharmaceuticals to treat obesity. Genetic models of obesity have been characterized in zebrafish including transgenic lines expressing obesogenic genes or mutants from targeted mutagenesis and genetic screens.
Underscoring the conservation of metabolic regulation, genetic manipulation of pathways that control body weight in mammalian systems also causes obesity in zebrafish. Transgenic zebrafish obesity models are often generated by mimicking existing mammalian models. The central melanocortin system (CMS), including peptides derived from proopiomelanocortin (POMC), their receptors (MC3R and MC4R), and Agouti-related peptide (AgRP), regulates energy homeostasis in zebrafish as it does in mammals (Ringholm et al., 2002; Hansen et al., 2003). Suppression of central melanocortin receptors by ectopic expression of the hair follicle restricted Agouti due to chromosomal translocations underlies one of the classical obese mouse models, Agouti Yellow (Bultman et al., 1992; Lu et al., 1994). This led to the identification of the endogenous melanocortin antagonist AgRP, whose transgenic overexpression in brain also causes obesity (Ollmann et al., 1997). In the zebrafish a genetic model of obesity has been developed by overexpressing AgRP [Tg(b-actin:AgRP)] (Song and Cone, 2007). These transgenic zebrafish exhibit an increase in body weight, linear growth, visceral adipose accumulation, and total triglycerides in all stages. The increased adiposity results from both hypertrophic and hyperplastic growth of adipocytes (Song and Cone, 2007). This transgenic zebrafish model demonstrates that central regulation of metabolism is conserved. The microRNA miR-27b has been suggested to be a regulatory hub for lipid metabolism by inhibiting the translation of a number of key lipid-metabolism genes (Vickers et al., 2013). Although several lines of evidence from cell culture support a role of miR-27b in lipid metabolism (Karbiener et al., 2009; Kang et al., 2013), there was a lack of in vivo supporting evidence. Recently, Hsu et al. generated transgenic zebrafish lines to deplete miR-27b by expressing a miR-27b sponge (C27bSP) driven by the ubiquitous beta-actin promoter (bC27bSP) or the hepatocyte-specific fabp10 promoter (hC27bSP). They demonstrated that the transgenic fish display hyperlipidemia, hepatic steatosis and increased white adipose tissue mass (Hsu et al., 2017), supporting a role of miR-27b in lipid metabolism in vivo. Another obesogenic model, Tg(krt4Hsa.myrAkt1)cy18, was initially generated to study skin cancer. However, the transgenic adults were found to be obese, with increased BMI, adipocyte hyperplasia, abnormal fat deposition, and glucose intolerance. These phenotypes likely result from ectopic expression of the constitutively active human AKT1 in several mesenchymal derived tissues (Chu et al., 2012). Tg(krt4Hsa.myrAkt1)cy18 fish differ from Tg(b-actin:AgRP) in two aspects. First, Tg(krt4Hsa.myrAkt1)cy18 fish do not display adipocyte hypertrophy. Second, Tg(krt4Hsa.myrAkt1)cy18 fish exhibit ectopic lipoma-like adipose tissue in dorsal muscle, gill arches, and tail bone tissues, whereas Tg(b-actin:AgRP) fish show a normal distribution of adipocyte tissues.
Multiple mutant lines have been identified that reveal genes and pathways contributing to lipid metabolism and adipose tissue regulation. These mutants are identified often because they have fatty liver at larval stages. Still other mutants are identified as adults due to increased adiposity. Although these mutants may share a common phenotype, they are due to the disruption of a diverse number of processes. Many mutants have larval hepatic steatosis, or fatty liver due to ER stress. In a “shelf screen” for liver size, Foie gras, and cdipthi559Tg/+ were identified because they displayed fatty liver by 5 days of age (Sadler et al., 2005; Thakur et al., 2011). The affected gene product in cdipthi559Tg/+, Cdipt, is necessary for phosphatidylinositol synthesis and lack of phosphatidylinositol causes ER stress and lipid accumulation in hepatocytes (Thakur et al., 2011). The Foie gras mutant results from mutation in transport protein particle 11 (trappc 11) that encodes a protein critical for ER to Golgi vesicular transport. As a result, the mutation causes pathogenic ER stress in hepatocytes, leading to fatty liver (DeRossi et al., 2016).
Beyond ER stress, other pathways leading to fatty liver have been identified using staining of never-fed mutant larvae with lipophilic dyes (Schlegel and Stainier, 2006; Kim et al., 2015; Hugo and Schlegel, 2017). One of these mutants, harvest moon (hmn), results from a mutation in glutamine-fructose-6-phosphate transamidase (gfpt1) gene (Hugo and Schlegel, 2017), while another mutant, 7466mu110, is caused by a mutation in vacuole membrane protein 1 (vmp1; Kim et al., 2015). These mutants feature lipid accumulation in hepatocytes and increased whole body adiposity and may provide clues to pathogenesis of fatty liver. Another mutant with fatty liver, ducttrip (dtp), was identified in a screen for mutations affecting the development of exocrine pancreas. The dtp mutant stems from a mutation in the gene encoding S-adenosylhomocysteine hydrolase and larvae exhibit mitochondrial dysfunction and liver degeneration in addition to hepatic steatosis and disrupted exocrine pancreas (Yee et al., 2005). While most fatty liver mutants do not survive to adulthood there are exceptions such as red moon (rmn) where both larvae and adults exhibit increased liver neutral lipids. The mutant is due to a loss-of-function of β-hydroxybutyrate transporter (slc16a6a; Hugo et al., 2012) and fatty liver results from the diversion of entrapped ketogenic precursor into lipids. Furthermore, the mutants are less tolerant of starvation. This mutant thus reveals a role of ketone body export in fasting energy homeostasis (Hugo et al., 2012). This diverse group of mutants highlights the complex regulation of lipid metabolism and how disruption at one node can lead to a phenotype of hepatic steatosis.
Other zebrafish mutants have alterations in adipose tissue. The zebrafish vizzini mutant exhibits decreased somatic growth and increased subcutaneous and visceral adipose tissues relative to body size. In vizzini, the subcutaneous adipose tissue (SAT) lipid droplets are extremely large although the number of lipid droplets in adipocytes is unchanged (McMenamin et al., 2013). This is due to a mutation in growth hormone 1 gene (gh1) leading to a premature stop codon. The phenotype is consistent with GH-deficient mice and humans that develop enlarged volume of SAT (Li et al., 1990; Wabitsch et al., 1995). Mutations in cyp2r1 gene (Peng et al., 2017) also results in growth retardation and increased adiposity. These cyp2r1 mutants are deficient in 1α,25(OH)2D3, the principal active form of vitamin D3, and 25(OH)D3 treatment rescues the growth and adiposity defects. In mammals, genetic and epidemiological data suggest a role of vitamin D deficiency in obesity, but vitamin D supplement fails to reduce the risk of obesity and associated pathologies (Rosen et al., 2012). These zebrafish mutants support a role of vitamin D in lipid metabolism and distribution. Mechanisms underlying distribution of adipose are critical as visceral fat is a better predictor than BMI of risk for cardiovascular diseases, insulin resistance and T2DM (Lebovitz and Banerji, 2005; InterAct et al., 2012). The loss of plexin d1 function in zebrafish specifically impacts visceral fat (Minchin et al., 2015). PLEXIN D1 is one of the 67 genes identified in GWAS analyses in humans to be associated with visceral fat mass (Shungin et al., 2015), but the function of PLEXIN D1 in the distribution of fat mass was unknown. In zebrafish plxnd1 mutants, visceral fat is reduced due to a decrease of lipid droplet size and adipocyte hyperplasia. Consequently, with high fat diet, the mutants preferentially store lipid in subcutaneous adipose tissue and are protected from developing insulin resistance (Minchin et al., 2015).
Taken together, these transgenic and mutant zebrafish lines further demonstrate conserved regulation of lipid metabolism. They also provide models in which to address mechanistic understanding of the underlying phenotypes.
Utilities of Zebrafish Obesity Models
One advantage of zebrafish models is the amenability for quick identification of chemical and genetic modifiers of the phenotype. The diet-induced obesity models have been used to test the effects of some dietary supplements on body fat accumulation. Green tea extract inhibited lipid accumulation (Tainaka et al., 2011; Meguro et al., 2015; Meguro and Hasumura, 2018), by decreasing the visceral adipose tissue volume and altering the expression of lipid catabolism genes (Hasumura et al., 2012). Eriocitrin, an antioxidative flavonoid in lemon, showed lipid-lowering effects in DIO zebrafish similar to that reported in a high-fat diet in rats (Hiramitsu et al., 2014). Oral administration of Yuzu (Citrus junos Siebold ex Tanaka) peel to DIO zebrafish exhibited anti-obesity effects by activating hepatic PPARα and adipocyte PPARγ pathways (Zang et al., 2014). Rhamnan sulfate, a sulphated polysaccharide from a marine green alga (Monostroma nitidum), also attenuated hepatic steatosis by suppressing lipogenesis (Zang et al., 2015a). Recently, a natural polyphenol, resveratrol, was reported to have anti-obesity effects via regulating lipid metabolism (Ran et al., 2017). Overall, DIO zebrafish is an attractive model system to evaluate the effects of functional foods and compounds on obesity development and treatment.
The diet-induced obesity models have also been used in drug testing. Tingaud-Sequeira et al. assessed the effect of small molecules on the whole-body adiposity after 24-h fasting in larvae previously overfed with egg yolk power (Tingaud-Sequeira et al., 2011). They found that two PPARγ agonists, rosiglitazone and TBT, a biocide found in antifouling paints, increases adiposity by inducing adipocyte hypertrophy and are thus obesogenic. In contrast, a PPARγ antagonist and an α1-adrenergic receptor agonist, known to promote lipolysis, are anti-obesogenic. Zhou et al. also performed proof of principle drug testing experiments using a similar model and found that all the 5 human hypolipidemic drugs exhibit significant hypolipidemic effect in zebrafish as they do in humans (Zhou et al., 2015). These results demonstrate the value of zebrafish obesity model on drug screening.
Genetic zebrafish models of obesity also provide mechanistic insights into the underlying causes. For instance, studies in the cyp2r1 mutants that show increased adiposity and growth retardation identified pgc1a as a direct target for vitamin D receptor. As Pgc1a is a known master regulator of mitochondrial biogenesis, the study further showed that the increased adiposity results from impaired mitochondrial function (Peng et al., 2017). Similarly, using the plxnd1 mutants, Minchin et al. investigated transcriptional changes in extracellular matrix genes (Minchin et al., 2015). They found that the mRNA and protein product of col5a1 was increased and the visceral fat in the plxnd1 mutants had more pronounced fibrillogenesis. Knocking down col5a1 normalized the defects in visceral fat.
One cautionary note is that not all of the lipid metabolism genes are highly conserved in sequence and function in zebrafish. For example, the leptin protein of zebrafish is only 19% identical to the human protein. In mice and humans, leptin is an adipostatic hormone that regulates adipose mass, and failure of leptin signaling results in hyperphagia and obesity (Myers et al., 2010). Unlike mammals, leptin, and leptin receptor are not expressed in adipose tissue in zebrafish. Leptin receptor-deficient zebrafish primarily have disrupted glucose homeostasis (Michel et al., 2016), which is different from phenotypes observed in mouse models such as severe hyperphagia, hyperlipidemia and morbid obesity (Yen et al., 1976).
These examples illustrate the utility of zebrafish models for mechanistic investigations, drug testing and drug discovery in obesity and lipid metabolism. Thus far, the power these models remain largely untapped. It is anticipated that more mechanistic discoveries will be made from these and other zebrafish obesity models.
Zebrafish Diabetes Models
Pancreas Development and Glucose Homeostasis in Zebrafish
The morphogenesis and basic cellular architecture of zebrafish pancreas is similar to mammalian pancreas (Tehrani and Lin, 2011) with both exocrine and endocrine compartments. The endocrine compartment consists of glucagon-secreting α-cells, insulin-producing β-cells, somatostatin-producing δ-cells, ghrelin-producing ε-cells and pancreatic polypeptide producing PP-cells. These cells are arranged in a manner similar to mouse islets (Argenton et al., 1999; Biemar et al., 2001). The signaling pathways and mechanisms of zebrafish endocrine pancreas development are highly homologous to those of mammals (Kinkel and Prince, 2009). In addition to the pancreas, development and function of other organ systems involved in glucose homeostasis, including brain, liver, adipocyte tissue and skeletal muscle, are also conserved (Maddison and Chen, 2017). The conservation of the pancreas structure and glucose homeostasis system make zebrafish useful to identify novel targets in pancreas related diseases such as diabetes.
Tools to Studies Glucose Homeostasis in Zebrafish
Numerous transgenic zebrafish strains with a fluorescent protein expression have been widely used to study pancreas development and glucose homeostasis in a whole living vertebrate (Kinkel and Prince, 2009; Tiso et al., 2009; Prince et al., 2017). For example, Tg(-1.2ins:EGFP) transgenic lines, where GFP expression is driven by the zebrafish preproinsulin promoter, provide a convenient fluorescent marker of β-cells (Xu et al., 2010) and insulin-expressing cells of the pancreatic islets can be visualized under a fluorescent microscope. Additionally, a transgenic line, Tg(gcga:GFP), where GFP is driven by zebrafish preproglucagon promotor, marks pancreatic α-cell (Zecchin et al., 2007). Using these cell-specific transgenic lines, the β-cell and α-cell area and total numbers are easily measured to evaluate alterations in cell mass and number, which is a predictor for glucose clearance (Li et al., 2015; Maddison et al., 2015).
Methods for zebrafish pancreas function have been established, including fasting and postprandial glucose measurement, and intraperitoneal glucose tolerance tests as well as techniques for pancreas dissection and islet cell culture (Eames et al., 2010; Eames Nalle et al., 2017). In larvae, blood collection for glucose measurement is not a viable methodology but free glucose in whole larvae can be measured by a coupled-enzyme fluorescent assay (Jurczyk et al., 2011). For adult zebrafish, the small size (3–4 cm) makes blood collection challenging. Nevertheless, several protocols for blood collection have been developed, such as lateral incision in the region of the dorsal aorta, decapitation and tail ablation although these methods require sacrifice of the animal (Jagadeeswaran et al., 1999; Eames et al., 2010; Velasco-Santamaría et al., 2011). However, a method for repeated blood collection in the same individual adult zebrafish has been developed (Zang et al., 2013, 2015b). Blood glucose can be measured by hand-held glucose-meters (Eames et al., 2010; Zang et al., 2015b). Protocols for glucose tolerance test (GTT) have also been developed in zebrafish (Kinkel et al., 2010; Matsuda et al., 2017; Zang et al., 2017), which is the most used approach to diagnose diabetes mellitus or glucose intolerance in humans.
Measuring insulin and insulin function has presented more of a challenge. As surrogate indicators, insulin mRNA levels can be determined directly by qPCR (Michel et al., 2016) and insulin promoter activity may be determined indirectly by measuring EGFP signal intensity in Tg(−1.0ins:EGFP)sc1 zebrafish (Zang et al., 2017). An insulin antibody for immunostaining both in whole larvae or adult zebrafish histologic sections is also available (Kimmel et al., 2015). Semi-quantitative dot-blot has been used to compare insulin levels in different fish simultaneously (Olsen et al., 2012). But insulin release has yet to be reliably measured in zebrafish. GFP has been used to replace the C-peptide of proinsulin in a transgenic line as one method to measure insulin release (Eames et al., 2013). Phosphorylation of Akt has been used to assess insulin function as a method to investigate early stage insulin resistance (Maddison et al., 2015; Landgraf et al., 2017). Insulin sensitivity can also be assessed by intraperitoneal injection of insulin in hyperglycemic zebrafish (Capiotti et al., 2014; Maddison et al., 2015).
Much of the biology in glucose homeostasis, from genes to organs, is conserved from zebrafish to humans. The application of powerful live imaging in zebrafish, coupled with genetic, and chemical genetic manipulations, will likely yield insights to many outstanding questions in diabetes.
Zebrafish Diabetes Models
Table 2 summaries zebrafish diabetes models developed by diet and genetic manipulations.
Type 1 Diabetes Mellitus Models
Type 1 diabetes mellitus (T1DM) is primarily an autoimmune disease caused by destruction of insulin producing pancreatic β-cells. Although an autoimmune derived T1DM model is lacking in zebrafish, several models have been developed using targeted β-cell damage. Three methods of β-cell destruction have been applied: surgical removal, chemical-dependent ablation, and genetic ablation. Pancreatectomy is feasible under the microscope in transgenic zebrafish with islet specific expression of GFP (Moss et al., 2009; Delaspre et al., 2015). However, this method is technically difficult and is not commonly used in zebrafish. Chemical-induced diabetes is widely used in rodents and also in zebrafish. Intraperitoneal injection of streptozotocin (STZ) is effective at β-cell ablation in adult zebrafish and eventually causes elevated fasting blood glucose and reduced insulin levels (Moss et al., 2009; Olsen et al., 2010; Intine et al., 2013). A total of 6 administrations of STZ within 4 weeks induces stable hyperglycemia and diabetic complications including retinopathy, nephropathy, and impaired fin regeneration. Alloxan can also selectively kill β-cells in zebrafish larvae (Nam et al., 2015; Castañeda et al., 2017). However, these compounds also exhibit other toxicity. Multiple genetic model of T1DM have been reported. Although stable expression of cell-lethal diphtheria toxin A chain (DTA) can eliminate all β-cells, these fish have growth retardation and fail to thrive (Ninov et al., 2013). Therefore, inducible β-cell ablation has been the preferred method for modeling T1DM. Two approaches of inducible β-cell ablation have been reported. In one approach, transgenic zebrafish lines with β-cell specific expression of the bacterial nitroreductase (NTR) enzyme, are exposed to the prodrug metronidazole (MTZ), the NTR substrate, which is converted into a cytotoxic compound that rapidly induces β-cell apoptosis (Curado et al., 2007, 2008; Pisharath et al., 2007; Ye et al., 2015). This NTR/MTZ ablation system is used for β-cell regeneration research as the elimination of β-cell occurs in 18–24 h after MTZ administration and recovers within 3–4 days after MTZ washout. A different approach has been to use a combinatorial, inducible transgene where the insulin promoter drives the expression of a doxycycline/ecdysone-dependent transcription factor and the TetOR-based promoter to express activated human Bid that triggers apoptosis (Li et al., 2014). Ablation models all face the same hurdle in that zebrafish have a remarkable regenerative capacity and β-cell mass is restored once the ablation mechanism is removed.
Type 2 Diabetes Mellitus Models
T2DM is characterized by insulin resistance and β-cell dysfunction. β-cell death may also occur in long standing T2DM. Both nutritional and genetic approaches have been used to generate T2DM models in zebrafish. Immersion of zebrafish in glucose solution is a widely-used method because of its convenience. Immersing adult zebrafish into alternating concentrations of 0 and 2% glucose every other day for 28–30 days, or chronic exposure to 2% glucose solution for 14 days, induces diabetic phenotypes, including elevated blood glucose levels and impaired response to exogenous insulin (Gleeson et al., 2007; Alvarez et al., 2010; Capiotti et al., 2014), similar to mice following 6 weeks of high-galactose diet (Joussen et al., 2009). Young zebrafish (4–11 months) acclimate to glucose exposure better than older zebrafish (1–3 years), but persistent hyperglycemia, can be achieved even in young zebrafish by gradually increasing the glucose concentration (Connaughton et al., 2016).
Obesity is the major risk factor for T2DM. High-fat diet causes both obesity and T2DM in rodent models (Winzell and Ahrén, 2004). In zebrafish, overfeeding with a commercial food quickly caused insulin resistance, elevated fasting blood glucose, and impaired glucose tolerance (Zang et al., 2017). Calorie restriction and anti-diabetic drugs (metformin and glimepiride) ameliorated the hyperglycemia in the overfed zebrafish. These drugs are both frequently prescribed treatments for T2DM and their effectiveness in the zebrafish model demonstrates conservation in glucose homeostasis pathways.
Insulin resistance is a major driver of T2DM. Our lab has developed two transgenic models of insulin resistance. In one model, skeletal muscle insulin resistance is achieved by transgenic expression of a dominant-negative IGF-I receptor (IGF-IR) in skeletal muscle. The transgenic fish showed impaired Akt phosphorylation postprandially or after insulin administration (Maddison et al., 2015). These fish had significantly increased fasting blood glucose as early as 3-month old compared to wild-type fish and is exacerbated by overfeeding (unpublished data). In the other model, insulin resistance is achieved through liver specific knockdown of the insulin receptors using CRISPR/Cas9 (Yin et al., 2015). Similar to mice and humans, liver insulin resistance causes fasting hypoglycemia and postprandial hyperglycemia. Since muscle and liver insulin resistance are thought to be the major drivers of T2DM, these models will be useful to dissect the progression of T2DM.
Another type of diabetes, MODY (maturity-onset diabetes of the young), is a rare, autosomal dominant, noninsulin-dependent and monogenic form of diabetes resulting from pancreatic β-cell dysfunction with an onset before 25 years of age. Since this disease is caused by mutation in a single gene, with different genes leading to different forms, MODY models can be developed by targeted gene ablation. However, as in mice, the mode of inheritance in MODY gene mutations is usually recessive, not autosomal dominant. MODY5 stems from mutations in hepatocyte nuclear factor 1β (HNF1β). A zebrafish hnf1ba mutant line (hnf1bas430) was identified from a zebrafish ENU mutagenesis screen (Lancman et al., 2013). The homozygous mutants exhibit pancreas hypoplasia and reduced β-cell numbers similar to MODY5. MODY6 results from mutations in NEUROD1 (Malecki et al., 1999). In mice, disruption of NeuroD1 leads to diabetes and premature death (Naya et al., 1997). In zebrafish neurod1 deficiency led to failed endocrine cell differentiation and increased free glucose levels in larvae (Dalgin and Prince, 2015). MODY4 is a result of PDX1 mutation (Stoffers et al., 1997) and a pdx1 mutant line exhibited reduced β-cell numbers, disrupted glucose homeostasis, sensitivity to over-nutrition and is responsive to anti-diabetic drug treatment (Kimmel et al., 2015). The adult pdx1 mutant zebrafish have small body size and decreased viability. MODY10 results from mutations in INS gene (Meur et al., 2010). A transgenic line expressing a mutated preproinsulin protein (C43G) has been developed (Eames et al., 2013). Interestingly, glucose homeostasis and β-cell mass were not altered in these fish, even though excess proinsulin accumulates in endoplasmic reticulum (ER). This could be due to the regenerative capacity of the zebrafish leading to turnover of the dysfunctional β-cells. However, this provides an opportunity to investigate misfolded proinsulin and ER stress in a non-diabetic in vivo system. Together, these MODY models develop phenotypes observed in patients, further supporting the utility of zebrafish as a diabetes model.
Although an appropriate model is still lacking for studying the long-term effect of diabetes, there have been approaches to study diabetic complications. For example, long immersion of larval or adult zebrafish in glucose solution has been used to model chronic hyperglycemia (Capiotti et al., 2014; Connaughton et al., 2016). This approach has been used to study diabetic retinopathy (Gleeson et al., 2007; Jung et al., 2016) as well as changes in bone metabolism (Carnovali et al., 2016). Inducing hyperglycemia through repeated STZ treatment in adult fish can impair wound healing (Olsen et al., 2010) and can cause heritable epigenetic changes after normalization of glycemia (Olsen et al., 2012). These studies underscore the lasting consequences of disrupting glucose control in zebrafish.
Overall, zebrafish offers particular advantages to the study of metabolic diseases. Models for studying obesity, pancreas regeneration, hyperglycemia, and diabetic complications have been established and will promote the understanding of the disease mechanisms, and provide new targets for disease therapy.
Author Contributions
All authors listed have made a substantial, direct and intellectual contribution to the work, and approved it for publication.
Funding
Supported by ADA 1-13-BS-27 and a Vanderbilt DRTC Pilot and Feasibility Grant (WC), R01 DK109407 (WC), and by JSPS KAKENHI Grant Number 15KK0305 (LZ).
Conflict of Interest Statement
The authors declare that the research was conducted in the absence of any commercial or financial relationships that could be construed as a potential conflict of interest.
References
Ahima, R. S., and Lazar, M. A. (2013). Physiology. The health risk of obesity–better metrics imperative. Science 341, 856–858. doi: 10.1126/science.1241244
Alvarez, Y., Chen, K., Reynolds, A. L., Waghorne, N., O'Connor, J. J., and Kennedy, B. N. (2010). Predominant cone photoreceptor dysfunction in a hyperglycaemic model of non-proliferative diabetic retinopathy. Dis. Model. Mech. 3, 236–245. doi: 10.1242/dmm.003772
Anderson, J. L., Carten, J. D., and Farber, S. A. (2011). Zebrafish lipid metabolism: from mediating early patterning to the metabolism of dietary fat and cholesterol. Methods Cell Biol. 101, 111–141. doi: 10.1016/B978-0-12-387036-0.00005-0
Argenton, F., Zecchin, E., and Bortolussi, M. (1999). Early appearance of pancreatic hormone-expressing cells in the zebrafish embryo. Mech. Dev. 87, 217–221. doi: 10.1016/S0925-4773(99)00151-3
Biemar, F., Argenton, F., Schmidtke, R., Epperlein, S., Peers, B., and Driever, W. (2001). Pancreas development in zebrafish: early dispersed appearance of endocrine hormone expressing cells and their convergence to form the definitive islet. Dev. Biol. 230, 189–203. doi: 10.1006/dbio.2000.0103
Bultman, S. J., Michaud, E. J., and Woychik, R. P. (1992). Molecular characterization of the mouse agouti locus. Cell 71, 1195–1204. doi: 10.1016/S0092-8674(05)80067-4
Cai, D. (2013). Neuroinflammation and neurodegeneration in overnutrition-induced diseases. Trends Endocrin. Met. 24, 40–47. doi: 10.1016/j.tem.2012.11.003
Capiotti, K. M., Antonioli, R. Jr., Kist, L. W., Bogo, M. R., Bonan, C. D., and Da Silva, R. S. (2014). Persistent impaired glucose metabolism in a zebrafish hyperglycemia model. Comp. Biochem. Physiol. B Biochem. Mol. Biol. 171, 58–65. doi: 10.1016/j.cbpb.2014.03.005
Carnovali, M., Luzi, L., Banfi, G., and Mariotti, M. (2016). Chronic hyperglycemia affects bone metabolism in adult zebrafish scale model. Endocrine 54, 808–817. doi: 10.1007/s12020-016-1106-3
Castañeda, R., Rodriguez, I., Nam, Y. H., Hong, B. N., and Kang, T. H. (2017). Trigonelline promotes auditory function through nerve growth factor signaling on diabetic animal models. Phytomedicine 36, 128–136. doi: 10.1016/j.phymed.2017.09.023
Chu, C. Y., Chen, C. F., Rajendran, R. S., Shen, C. N., Chen, T. H., Yen, C. C., et al. (2012). Overexpression of Akt1 enhances adipogenesis and leads to lipoma formation in zebrafish. PLoS ONE 7:e36474. doi: 10.1371/journal.pone.0036474
Connaughton, V. P., Baker, C., Fonde, L., Gerardi, E., and Slack, C. (2016). Alternate immersion in an external glucose solution differentially affects blood sugar values in older versus younger zebrafish adults. Zebrafish 13, 87–94. doi: 10.1089/zeb.2015.1155
Curado, S., Anderson, R. M., Jungblut, B., Mumm, J., Schroeter, E., and Stainier, D. Y. (2007). Conditional targeted cell ablation in zebrafish: a new tool for regeneration studies. Dev. Dyn. 236, 1025–1035. doi: 10.1002/dvdy.21100
Curado, S., Stainier, D. Y., and Anderson, R. M. (2008). Nitroreductase-mediated cell/tissue ablation in zebrafish: a spatially and temporally controlled ablation method with applications in developmental and regeneration studies. Nat. Protoc. 3, 948–954. doi: 10.1038/nprot.2008.58
Dailey, M. J. (2014). Nutrient-induced intestinal adaption and its effect in obesity. Physiol. Behav. 136, 74–78. doi: 10.1016/j.physbeh.2014.03.026
Dalgin, G., and Prince, V. E. (2015). Differential levels of Neurod establish zebrafish endocrine pancreas cell fates. Dev. Biol. 402, 81–97. doi: 10.1016/j.ydbio.2015.03.007
Delaspre, F., Beer, R. L., Rovira, M., Huang, W., Wang, G., Gee, S., et al. (2015). Centroacinar cells are progenitors that contribute to endocrine pancreas regeneration. Diabetes 64, 3499–3509. doi: 10.2337/db15-0153
Den Broeder, M. J., Kopylova, V. A., Kamminga, L. M., and Legler, J. (2015). Zebrafish as a model to study the role of peroxisome proliferating-activated receptors in adipogenesis and obesity. PPAR Res. 2015:358029. doi: 10.1155/2015/358029
den Broeder, M. J., Moester, M. J. B., Kamstra, J. H., Cenijn, P. H., Davidoiu, V., Kamminga, L. M., et al. (2017). Altered adipogenesis in zebrafish larvae following high fat diet and chemical exposure is visualised by stimulated raman scattering microscopy. Int. J. Mol. Sci. 18:E894. doi: 10.3390/ijms18040894
DeRossi, C., Vacaru, A., Rafiq, R., Cinaroglu, A., Imrie, D., Nayar, S., et al. (2016). Trappc11 is required for protein glycosylation in zebrafish and humans. Mol. Biol. Cell 27, 1220–1234. doi: 10.1091/mbc.e15-08-0557
Dooley, K., and Zon, L. I. (2000). Zebrafish: a model system for the study of human disease. Curr. Opin. Genet. Dev. 10, 252–256. doi: 10.1016/S0959-437X(00)00074-5
Eames Nalle, S. C., Franse, K. F., and Kinkel, M. D. (2017). Analysis of pancreatic disease in zebrafish. Method Cell Biol. 138, 271–295. doi: 10.1016/bs.mcb.2016.08.005
Eames, S. C., Kinkel, M. D., Rajan, S., Prince, V. E., and Philipson, L. H. (2013). Transgenic zebrafish model of the C43G human insulin gene mutation. J. Diabetes Investig. 4, 157–167. doi: 10.1111/jdi.12015
Eames, S. C., Philipson, L. H., Prince, V. E., and Kinkel, M. D. (2010). Blood sugar measurement in zebrafish reveals dynamics of glucose homeostasis. Zebrafish 7, 205–213. doi: 10.1089/zeb.2009.0640
Elo, B., Villano, C. M., Govorko, D., and White, L. A. (2007). Larval zebrafish as a model for glucose metabolism: expression of phosphoenolpyruvate carboxykinase as a marker for exposure to anti-diabetic compounds. J. Mol. Endocrinol. 38, 433–440. doi: 10.1677/JME-06-0037
Feierstein, C. E., Portugues, R., and Orger, M. B. (2015). Seeing the whole picture: a comprehensive imaging approach to functional mapping of circuits in behaving zebrafish. Neuroscience 296, 26–38. doi: 10.1016/j.neuroscience.2014.11.046
Flegal, K. M., Kit, B. K., Orpana, H., and Graubard, B. I. (2013). Association of all-cause mortality with overweight and obesity using standard body mass index categories: a systematic review and meta-analysis. JAMA 309, 71–82. doi: 10.1001/jama.2012.113905
Flynn, E. J. III., Trent, C. M., and Rawls, J. F. (2009). Ontogeny and nutritional control of adipogenesis in zebrafish (Danio rerio). J. Lipid Res. 50, 1641–1652. doi: 10.1194/jlr.M800590-JLR200
Freifeld, L., Odstrcil, I., Förster, D., Ramirez, A., Gagnon, J. A., Randlett, O., et al. (2017). Expansion microscopy of zebrafish for neuroscience and developmental biology studies. Proc. Natl. Acad. Sci. U.S.A. 114, E10799–E10808. doi: 10.1073/pnas.1706281114
Fuchsberger, C., Flannick, J., Teslovich, T. M., Mahajan, A., Agarwala, V., Gaulton, K. J., et al. (2016). The genetic architecture of type 2 diabetes. Nature 536, 41–47. doi: 10.1038/nature18642
Gibert, Y., Trengove, M. C., and Ward, A. C. (2013). Zebrafish as a genetic model in pre-clinical drug testing and screening. Curr. Med. Chem. 20, 2458–2466. doi: 10.2174/0929867311320190005
Gleeson, M., Connaughton, V., and Arneson, L. S. (2007). Induction of hyperglycaemia in zebrafish (Danio rerio) leads to morphological changes in the retina. Acta Diabetol. 44, 157–163. doi: 10.1007/s00592-007-0257-3
Greenspan, P., Mayer, E. P., and Fowler, S. D. (1985). Nile red: a selective fluorescent stain for intracellular lipid droplets. J. Cell Biol. 100, 965–973. doi: 10.1083/jcb.100.3.965
Hall, C., Flores, M. V., Crosier, K., and Crosier, P. (2009). Live cell imaging of zebrafish leukocytes. Methods Mol. Biol. 546, 255–271. doi: 10.1007/978-1-60327-977-2_16
Hansen, I. A., To, T. T., Wortmann, S., Burmester, T., Winkler, C., Meyer, S. R., et al. (2003). The pro-opiomelanocortin gene of the zebrafish (Danio rerio). Biochem. Biophys. Res. Commun. 303, 1121–1128. doi: 10.1016/S0006-291X(03)00475-3
Haslam, D. W., and James, W. P. (2005). Obesity. Lancet 366, 1197–1209. doi: 10.1016/S0140-6736(05)67483-1
Hasumura, T., Shimada, Y., Kuroyanagi, J., Nishimura, Y., Meguro, S., Takema, Y., et al. (2012). Green tea extract suppresses adiposity and affects the expression of lipid metabolism genes in diet-induced obese zebrafish. Nutr. Metab. 9:73. doi: 10.1186/1743-7075-9-73
Herrgen, L., Schröter, C., Bajard, L., and Oates, A. C. (2009). Multiple embryo time-lapse imaging of zebrafish development. Methods Mol. Biol. 546, 243–254. doi: 10.1007/978-1-60327-977-2_15
Hiramitsu, M., Shimada, Y., Kuroyanagi, J., Inoue, T., Katagiri, T., Zang, L., et al. (2014). Eriocitrin ameliorates diet-induced hepatic steatosis with activation of mitochondrial biogenesis. Sci. Rep. 4:3708. doi: 10.1038/srep03708
Hölttä-Vuori, M., Salo, V. T., Nyberg, L., Brackmann, C., Enejder, A., Panula, P., et al. (2010). Zebrafish: gaining popularity in lipid research. Biochem. J. 429, 235–242. doi: 10.1042/BJ20100293
Hoshijima, K., Jurynec, M. J., and Grunwald, D. J. (2016). Precise editing of the zebrafish genome made simple and efficient. Dev. Cell 36, 654–667. doi: 10.1016/j.devcel.2016.02.015
Hsu, C. C., Lai, C. Y., Lin, C. Y., Yeh, K. Y., and Her, G. M. (2017). MicroRNA-27b Depletion Enhances Endotrophic and Intravascular Lipid Accumulation and Induces Adipocyte Hyperplasia in Zebrafish. Int. J. Mol. Sci. 19:E93. doi: 10.3390/ijms19010093
Hugo, S. E., Cruz-Garcia, L., Karanth, S., Anderson, R. M., Stainier, D. Y., and Schlegel, A. (2012). A monocarboxylate transporter required for hepatocyte secretion of ketone bodies during fasting. Genes Dev. 26, 282–293. doi: 10.1101/gad.180968.111
Hugo, S. E., and Schlegel, A. (2017). A genetic screen for zebrafish mutants with hepatic steatosis identifies a locus required for larval growth. J. Anat. 230, 407–413. doi: 10.1111/joa.12570
Imrie, D., and Sadler, K. C. (2010). White adipose tissue development in zebrafish is regulated by both developmental time and fish size. Dev. Dyn. 239, 3013–3023. doi: 10.1002/dvdy.22443
InterAct, C., Langenberg, C., Sharp, S. J., Schulze, M. B., Rolandsson, O., Overvad, K., et al. (2012). Long-term risk of incident type 2 diabetes and measures of overall and regional obesity: the EPIC-InterAct case-cohort study. PLoS Med. 9:e1001230. doi: 10.1371/journal.pmed.1001230
Intine, R. V., Olsen, A. S., and Sarras, M. P. Jr. (2013). A zebrafish model of diabetes mellitus and metabolic memory. J. Vis. Exp. 72:e50232. doi: 10.3791/50232
Jagadeeswaran, P., Sheehan, J. P., Craig, F. E., and Troyer, D. (1999). Identification and characterization of zebrafish thrombocytes. Br. J. Haematol. 107, 731–738. doi: 10.1046/j.1365-2141.1999.01763.x
Jones, K. S., Alimov, A. P., Rilo, H. L., Jandacek, R. J., Woollett, L. A., and Penberthy, W. T. (2008). A high throughput live transparent animal bioassay to identify non-toxic small molecules or genes that regulate vertebrate fat metabolism for obesity drug development. Nutr. Metab. 5:23. doi: 10.1186/1743-7075-5-23
Joussen, A. M., Doehmen, S., Le, M. L., Koizumi, K., Radetzky, S., Krohne, T. U., et al. (2009). TNF-alpha mediated apoptosis plays an important role in the development of early diabetic retinopathy and long-term histopathological alterations. Mol. Vis. 15, 1418–1428.
Jung, S. H., Kim, Y. S., Lee, Y. R., and Kim, J. S. (2016). High glucose-induced changes in hyaloid-retinal vessels during early ocular development of zebrafish: a short-term animal model of diabetic retinopathy. Br. J. Pharmacol. 173, 15–26. doi: 10.1111/bph.13279
Jurczyk, A., Roy, N., Bajwa, R., Gut, P., Lipson, K., Yang, C., et al. (2011). Dynamic glucoregulation and mammalian-like responses to metabolic and developmental disruption in zebrafish. Gen. Comp. Endocrinol. 170, 334–345. doi: 10.1016/j.ygcen.2010.10.010
Kang, T., Lu, W., Xu, W., Anderson, L., Bacanamwo, M., Thompson, W., et al. (2013). MicroRNA-27 (miR-27) targets prohibitin and impairs adipocyte differentiation and mitochondrial function in human adipose-derived stem cells. J. Biol. Chem. 288, 34394–34402. doi: 10.1074/jbc.M113.514372
Karbiener, M., Fischer, C., Nowitsch, S., Opriessnig, P., Papak, C., Ailhaud, G., et al. (2009). microRNA miR-27b impairs human adipocyte differentiation and targets PPARgamma. Biochem. Biophys. Res. Commun. 390, 247–251. doi: 10.1016/j.bbrc.2009.09.098
Kim, S. H., Wu, S. Y., Baek, J. I., Choi, S. Y., Su, Y., Flynn, C. R., et al. (2015). A post-developmental genetic screen for zebrafish models of inherited liver disease. PLoS ONE 10:e0125980. doi: 10.1371/journal.pone.0125980
Kimmel, C. B., Ballard, W. W., Kimmel, S. R., Ullmann, B., and Schilling, T. F. (1995). Stages of embryonic development of the zebrafish. Dev. Dyn. 203, 253–310. doi: 10.1002/aja.1002030302
Kimmel, R. A., Dobler, S., Schmitner, N., Walsen, T., Freudenblum, J., and Meyer, D. (2015). Diabetic pdx1-mutant zebrafish show conserved responses to nutrient overload and anti-glycemic treatment. Sci. Rep. 5:14241. doi: 10.1038/srep14241
Kimura, Y., Hisano, Y., Kawahara, A., and Higashijima, S. (2014). Efficient generation of knock-in transgenic zebrafish carrying reporter/driver genes by CRISPR/Cas9-mediated genome engineering. Sci. Rep. 4:6545. doi: 10.1038/srep06545
Kinkel, M. D., Eames, S. C., Philipson, L. H., and Prince, V. E. (2010). Intraperitoneal injection into adult zebrafish. J. Vis. Exp. 42:2126. doi: 10.3791/2126
Kinkel, M. D., and Prince, V. E. (2009). On the diabetic menu: zebrafish as a model for pancreas development and function. Bioessays 31, 139–152. doi: 10.1002/bies.200800123
Kokkinos, P., Myers, J., Nylen, E., Panagiotakos, D. B., Manolis, A., Pittaras, A., et al. (2009). Exercise capacity and all-cause mortality in African American and Caucasian men with type 2 diabetes. Diabetes Care 32, 623–628. doi: 10.2337/dc08-1876
Kopp, R., Billecke, N., Legradi, J., den Broeder, M., Parekh, S. H., and Legler, J. (2016). Bringing obesity to light: rev-erbalpha, a central player in light-induced adipogenesis in the zebrafish? Int. J. Obes. 40, 824–832. doi: 10.1038/ijo.2015.240
Lancman, J. J., Zvenigorodsky, N., Gates, K. P., Zhang, D., Solomon, K., Humphrey, R. K., et al. (2013). Specification of hepatopancreas progenitors in zebrafish by hnf1ba and wnt2bb. Development 140, 2669–2679. doi: 10.1242/dev.090993
Landgraf, K., Schuster, S., Meusel, A., Garten, A., Riemer, T., Schleinitz, D., et al. (2017). Short-term overfeeding of zebrafish with normal or high-fat diet as a model for the development of metabolically healthy versus unhealthy obesity. BMC Physiol. 17, 4. doi: 10.1186/s12899-017-0031-x
Lawlor, N., Khetan, S., Ucar, D., and Stitzel, M. L. (2017). Genomics of Islet (Dys)function and Type 2 Diabetes. Trends Genet. 33, 244–255. doi: 10.1016/j.tig.2017.01.010
Lebovitz, H. E., and Banerji, M. A. (2005). Point: visceral adiposity is causally related to insulin resistance. Diabetes Care 28, 2322–2325. doi: 10.2337/diacare.28.9.2322
Leung, M. Y., Carlsson, N. P., Colditz, G. A., and Chang, S. H. (2017). The burden of obesity on diabetes in the united states: medical expenditure panel survey, 2008 to 2012. Value Health 20, 77–84. doi: 10.1016/j.jval.2016.08.735
Li, M., Dean, E. D., Zhao, L., Nicholson, W. E., Powers, A. C., and Chen, W. (2015). Glucagon receptor inactivation leads to alpha-cell hyperplasia in zebrafish. J. Endocrinol. 227, 93–103. doi: 10.1530/JOE-15-0284
Li, M., Maddison, L. A., Page-McCaw, P., and Chen, W. (2014). Overnutrition induces beta-cell differentiation through prolonged activation of beta-cells in zebrafish larvae. Am. J. Physiol. Endocrinol. Metab. 306, E799–E807. doi: 10.1152/ajpendo.00686.2013
Li, S., Crenshaw, E. B. III., Rawson, E. J., Simmons, D. M., Swanson, L. W., and Rosenfeld, M. G. (1990). Dwarf locus mutants lacking three pituitary cell types result from mutations in the POU-domain gene pit-1. Nature 347, 528–533. doi: 10.1038/347528a0
Lieschke, G. J., and Currie, P. D. (2007). Animal models of human disease: zebrafish swim into view. Nat. Rev. Genet. 8, 353–367. doi: 10.1038/nrg2091
Loos, R. J. (2018). The genetics of adiposity. Curr. Opin. Genet. Dev. 50, 86–95. doi: 10.1016/j.gde.2018.02.009
Lu, D., Willard, D., Patel, I. R., Kadwell, S., Overton, L., Kost, T., et al. (1994). Agouti protein is an antagonist of the melanocyte-stimulating-hormone receptor. Nature 371, 799–802. doi: 10.1038/371799a0
MacRae, C. A., and Peterson, R. T. (2015). Zebrafish as tools for drug discovery. Nat. Rev. Drug Discov. 14, 721–731. doi: 10.1038/nrd4627
Maddison, L. A., and Chen, W. B. (2017). Modeling pancreatic endocrine cell adaptation and diabetes in the zebrafish. Front Endocrinol. 8:9. doi: 10.3389/fendo.2017.00009
Maddison, L. A., Joest, K. E., Kammeyer, R. M., and Chen, W. (2015). Skeletal muscle insulin resistance in zebrafish induces alterations in beta-cell number and glucose tolerance in an age- and diet-dependent manner. Am. J. Physiol. Endocrinol. Metab. 308, E662–E669. doi: 10.1152/ajpendo.00441.2014
Malecki, M. T., Jhala, U. S., Antonellis, A., Fields, L., Doria, A., Orban, T., et al. (1999). Mutations in NEUROD1 are associated with the development of type 2 diabetes mellitus. Nat. Genet. 23, 323–328. doi: 10.1038/15500
Marza, E., Barthe, C., André, M., Villeneuve, L., Hélou, C., and Babin, P. J. (2005). Developmental expression and nutritional regulation of a zebrafish gene homologous to mammalian microsomal triglyceride transfer protein large subunit. Dev. Dyn. 232, 506–518. doi: 10.1002/dvdy.20251
Matsuda, H., Mullapudi, S. T., Zhang, Y. X., Hesselson, D., and Stainier, D. Y. R. (2017). Thyroid hormone coordinates pancreatic islet maturation during the zebrafish larval-to-juvenile transition to maintain glucose homeostasis. Diabetes 66, 2623–2635. doi: 10.2337/db16-1476
McMenamin, S. K., Minchin, J. E., Gordon, T. N., Rawls, J. F., and Parichy, D. M. (2013). Dwarfism and increased adiposity in the gh1 mutant zebrafish vizzini. Endocrinology 154, 1476–1487. doi: 10.1210/en.2012-1734
Meguro, S., and Hasumura, T. (2018). Fish oil suppresses body fat accumulation in zebrafish. Zebrafish 15, 27–32. doi: 10.1089/zeb.2017.1475
Meguro, S., Hasumura, T., and Hase, T. (2015). Body fat accumulation in zebrafish is induced by a diet rich in fat and reduced by supplementation with green tea extract. PLoS ONE 10:e0120142. doi: 10.1371/journal.pone.0120142
Meur, G., Simon, A., Harun, N., Virally, M., Dechaume, A., Bonnefond, A., et al. (2010). Insulin gene mutations resulting in early-onset diabetes: marked differences in clinical presentation, metabolic status, and pathogenic effect through endoplasmic reticulum retention. Diabetes 59, 653–661. doi: 10.2337/db09-1091
Michel, M., Page-McCaw, P. S., Chen, W., and Cone, R. D. (2016). Leptin signaling regulates glucose homeostasis, but not adipostasis, in the zebrafish. Proc. Natl. Acad. Sci. U.S.A. 113, 3084–3089. doi: 10.1073/pnas.1513212113
Minchin, J. E., Dahlman, I., Harvey, C. J., Mejhert, N., Singh, M. K., Epstein, J. A., et al. (2015). Plexin D1 determines body fat distribution by regulating the type V collagen microenvironment in visceral adipose tissue. Proc. Natl. Acad. Sci. U.S.A. 112, 4363–4368. doi: 10.1073/pnas.1416412112
Moss, J. B., Koustubhan, P., Greenman, M., Parsons, M. J., Walter, I., and Moss, L. G. (2009). Regeneration of the pancreas in adult zebrafish. Diabetes 58, 1844–1851. doi: 10.2337/db08-0628
Myers, M. G. Jr., Leibel, R. L., Seeley, R. J., and Schwartz, M. W. (2010). Obesity and leptin resistance: distinguishing cause from effect. Trends Endocrinol. Metab. 21, 643–651. doi: 10.1016/j.tem.2010.08.002
Nam, Y. H., Hong, B. N., Rodriguez, I., Ji, M. G., Kim, K., Kim, U. J., et al. (2015). Synergistic potentials of coffee on injured pancreatic islets and insulin action via KATP channel blocking in zebrafish. J. Agric. Food Chem. 63, 5612–5621. doi: 10.1021/acs.jafc.5b00027
Naya, F. J., Huang, H. P., Qiu, Y. H., Mutoh, H., DeMayo, F. J., Leiter, A. B., et al. (1997). Diabetes, defective pancreatic morphogenesis, and abnormal enteroendocrine differentiation in BETA2/NeuroD-deficient mice. Genes Dev. 11, 2323–2334. doi: 10.1101/gad.11.18.2323
Ninov, N., Hesselson, D., Gut, P., Zhou, A., Fidelin, K., and Stainier, D. Y. (2013). Metabolic regulation of cellular plasticity in the pancreas. Curr. Biol. 23, 1242–1250. doi: 10.1016/j.cub.2013.05.037
Nishio, S., Gibert, Y., Berekelya, L., Bernard, L., Brunet, F., Guillot, E., et al. (2012). Fasting induces CART down-regulation in the zebrafish nervous system in a cannabinoid receptor 1-dependent manner. Mol. Endocrinol. 26, 1316–1326. doi: 10.1210/me.2011-1180
Oka, T., Nishimura, Y., Zang, L., Hirano, M., Shimada, Y., Wang, Z., et al. (2010). Diet-induced obesity in zebrafish shares common pathophysiological pathways with mammalian obesity. BMC Physiol. 10:21. doi: 10.1186/1472-6793-10-21
Ollmann, M. M., Wilson, B. D., Yang, Y. K., Kerns, J. A., Chen, Y., Gantz, I., et al. (1997). Antagonism of central melanocortin receptors in vitro and in vivo by agouti-related protein. Science 278, 135–138. doi: 10.1126/science.278.5335.135
Olsen, A. S., Sarras, M. P. Jr., and Intine, R. V. (2010). Limb regeneration is impaired in an adult zebrafish model of diabetes mellitus. Wound Repair Regener. 18, 532–542. doi: 10.1111/j.1524-475X.2010.00613.x
Olsen, A. S., Sarras, M. P. Jr., Leontovich, A., and Intine, R. V. (2012). Heritable transmission of diabetic metabolic memory in zebrafish correlates with DNA hypomethylation and aberrant gene expression. Diabetes 61, 485–491. doi: 10.2337/db11-0588
Peng, X., Shang, G., Wang, W., Chen, X., Lou, Q., Zhai, G., et al. (2017). Fatty acid oxidation in zebrafish adipose tissue is promoted by 1alpha,25(OH)2D3. Cell Rep. 19, 1444–1455. doi: 10.1016/j.celrep.2017.04.066
Periasamy, M., Herrera, J. L., and Reis, F. C. G. (2017). Skeletal muscle thermogenesis and its role in whole body energy metabolism. Diabetes Metab. J. 41, 327–336. doi: 10.4093/dmj.2017.41.5.327
Pisharath, H., Rhee, J. M., Swanson, M. A., Leach, S. D., and Parsons, M. J. (2007). Targeted ablation of beta cells in the embryonic zebrafish pancreas using E. coli nitroreductase. Mech. Dev. 124, 218–229. doi: 10.1016/j.mod.2006.11.005
Poulsen, P., Vaag, A., Kyvik, K., and Beck-Nielsen, H. (2001). Genetic versus environmental aetiology of the metabolic syndrome among male and female twins. Diabetologia 44, 537–543. doi: 10.1007/s001250051659
Prince, V. E., Anderson, R. M., and Dalgin, G. (2017). Zebrafish pancreas development and regeneration: fishing for diabetes therapies. Curr. Top. Dev. Biol. 124, 235–276. doi: 10.1016/bs.ctdb.2016.10.005
Ran, G., Ying, L., Li, L., Yan, Q., Yi, W., Ying, C., et al. (2017). Resveratrol ameliorates diet-induced dysregulation of lipid metabolism in zebrafish (Danio rerio). PLoS ONE 12:e0180865. doi: 10.1371/journal.pone.0180865
Ringholm, A., Fredriksson, R., Poliakova, N., Yan, Y. L., Postlethwait, J. H., Larhammar, D., et al. (2002). One melanocortin 4 and two melanocortin 5 receptors from zebrafish show remarkable conservation in structure and pharmacology. J. Neurochem. 82, 6–18. doi: 10.1046/j.1471-4159.2002.00934.x
Rosen, C. J., Adams, J. S., Bikle, D. D., Black, D. M., Demay, M. B., Manson, J. E., et al. (2012). The nonskeletal effects of vitamin D: an endocrine society scientific statement. Endocr. Rev. 33, 456–492. doi: 10.1210/er.2012-1000
Sadler, K. C., Amsterdam, A., Soroka, C., Boyer, J., and Hopkins, N. (2005). A genetic screen in zebrafish identifies the mutants vps18, nf2 and foie gras as models of liver disease. Development 132, 3561–3572. doi: 10.1242/dev.01918
Schlegel, A., and Stainier, D. Y. (2006). Microsomal triglyceride transfer protein is required for yolk lipid utilization and absorption of dietary lipids in zebrafish larvae. Biochemistry 45, 15179–15187. doi: 10.1021/bi0619268
Schlegel, A., and Stainier, D. Y. (2007). Lessons from “lower” organisms: what worms, flies, and zebrafish can teach us about human energy metabolism. PLoS Genet. 3:e199. doi: 10.1371/journal.pgen.0030199
Schlombs, K., Wagner, T., and Scheel, J. (2003). Site-1 protease is required for cartilage development in zebrafish. Proc. Natl. Acad. Sci. U.S.A. 100, 14024–14029. doi: 10.1073/pnas.2331794100
Shungin, D., Winkler, T. W., Croteau-Chonka, D. C., Ferreira, T., Locke, A. E., Mägi, R., et al. (2015). New genetic loci link adipose and insulin biology to body fat distribution. Nature 518, 187–196. doi: 10.1038/nature14132
Song, Y., and Cone, R. D. (2007). Creation of a genetic model of obesity in a teleost. FASEB J. 21, 2042–2049. doi: 10.1096/fj.06-7503com
Stoffers, D. A., Ferrer, J., Clarke, W. L., and Habener, J. F. (1997). Early-onset type-II diabetes mellitus (MODY4) linked to IPF1. Nat. Genet. 17, 138–139. doi: 10.1038/ng1097-138
Tainaka, T., Shimada, Y., Kuroyanagi, J., Zang, L., Oka, T., Nishimura, Y., et al. (2011). Transcriptome analysis of anti-fatty liver action by Campari tomato using a zebrafish diet-induced obesity model. Nutr. Metab. 8:88. doi: 10.1186/1743-7075-8-88
Tehrani, Z., and Lin, S. (2011). Endocrine pancreas development in zebrafish. Cell Cycle 10, 3466–3472. doi: 10.4161/cc.10.20.17764
Thakur, P. C., Stuckenholz, C., Rivera, M. R., Davison, J. M., Yao, J. K., Amsterdam, A., et al. (2011). Lack of de novo phosphatidylinositol synthesis leads to endoplasmic reticulum stress and hepatic steatosis in cdipt-deficient zebrafish. Hepatology 54, 452–462. doi: 10.1002/hep.24349
Tingaud-Sequeira, A., Ouadah, N., and Babin, P. J. (2011). Zebrafish obesogenic test: a tool for screening molecules that target adiposity. J. Lipid Res. 52, 1765–1772. doi: 10.1194/jlr.D017012
Tiso, N., Moro, E., and Argenton, F. (2009). Zebrafish pancreas development. Mol. Cell. Endocrinol. 312, 24–30. doi: 10.1016/j.mce.2009.04.018
Vandevijvere, S., Chow, C. C., Hall, K. D., Umali, E., and Swinburn, B. A. (2015). Increased food energy supply as a major driver of the obesity epidemic: a global analysis. Bull. World Health Organ. 93, 446–456. doi: 10.2471/BLT.14.150565
Vargas, R., and Vásquez, I. C. (2017). Effects of overfeeding and high-fat diet on cardiosomatic parameters and cardiac structures in young and adult zebrafish. Fish. Physiol. Biochem. 43, 1761–1773. doi: 10.1007/s10695-017-0407-7
Velasco-Santamaría, Y. M., Korsgaard, B., Madsen, S. S., and Bjerregaard, P. (2011). Bezafibrate, a lipid-lowering pharmaceutical, as a potential endocrine disruptor in male zebrafish (Danio rerio). Aquat. Toxicol. 105, 107–118. doi: 10.1016/j.aquatox.2011.05.018
Vickers, K. C., Shoucri, B. M., Levin, M. G., Wu, H., Pearson, D. S., Osei-Hwedieh, D., et al. (2013). MicroRNA-27b is a regulatory hub in lipid metabolism and is altered in dyslipidemia. Hepatology 57, 533–542. doi: 10.1002/hep.25846
Wabitsch, M., Hauner, H., Heinze, E., and Teller, W. M. (1995). The role of growth hormone/insulin-like growth factors in adipocyte differentiation. Metab. Clin. Exp. 44, 45–49. doi: 10.1016/0026-0495(95)90220-1
WHO. (2017). Obesity and Overweight. Available online at: http://www.who.int/news-room/fact-sheets/detail/obesity-and-overweight
Willemsen, G., Ward, K. J., Bell, C. G., Christensen, K., Bowden, J., Dalgård, C., et al. (2015). The concordance and heritability of type 2 diabetes in 34,166 twin pairs from international twin registers: the discordant twin (DISCOTWIN) consortium. Twin Res. Hum. Genet. 18, 762–771. doi: 10.1017/thg.2015.83
Winzell, M. S., and Ahrén, B. (2004). The high-fat diet-fed mouse: a model for studying mechanisms and treatment of impaired glucose tolerance and type 2 diabetes. Diabetes 53 (Suppl. 3), S215–S219. doi: 10.2337/diabetes.53.suppl_3.S215
Xu, P. F., Zhu, K. Y., Jin, Y., Chen, Y., Sun, X. J., Deng, M., et al. (2010). Setdb2 restricts dorsal organizer territory and regulates left-right asymmetry through suppressing fgf8 activity. Proc. Natl. Acad. Sci. U.S.A. 107, 2521–2526. doi: 10.1073/pnas.0914396107
Ye, L., Robertson, M. A., Hesselson, D., Stainier, D. Y. R., and Anderson, R. M. (2015). glucagon is essential for alpha cell transdifferentiation and beta cell neogenesis. Development 142, 1407–1417. doi: 10.1242/dev.117911
Yee, N. S., Lorent, K., and Pack, M. (2005). Exocrine pancreas development in zebrafish. Dev. Biol. 284, 84–101. doi: 10.1016/j.ydbio.2005.04.035
Yen, T. T., Allan, J. A., Yu, P. L., Acton, M. A., and Pearson, D. V. (1976). Triacylglycerol contents and in vivo lipogenesis of ob/ob, db/db and Avy/a mice. Biochim. Biophys. Acta 441, 213–220. doi: 10.1016/0005-2760(76)90164-8
Yin, L., Maddison, L. A., and Chen, W. (2016). Multiplex conditional mutagenesis in zebrafish using the CRISPR/Cas system. Methods Cell Biol. 135, 3–17. doi: 10.1016/bs.mcb.2016.04.018
Yin, L., Maddison, L. A., Li, M., Kara, N., LaFave, M. C., Varshney, G. K., et al. (2015). Multiplex conditional mutagenesis using transgenic expression of Cas9 and sgRNAs. Genetics 200, 431–441. doi: 10.1534/genetics.115.176917
Zang, L., Shimada, Y., Kawajiri, J., Tanaka, T., and Nishimura, N. (2014). Effects of Yuzu (Citrus junos Siebold ex Tanaka) peel on the diet-induced obesity in a zebrafish model. J. Funct. Foods 10, 499–510. doi: 10.1016/j.jff.2014.08.002
Zang, L., Shimada, Y., and Nishimura, N. (2017). Development of a novel zebrafish model for type 2 diabetes mellitus. Sci. Rep. 7:1461 doi: 10.1038/s41598-017-01432-w
Zang, L., Shimada, Y., Nishimura, Y., Tanaka, T., and Nishimura, N. (2013). A novel, reliable method for repeated blood collection from aquarium fish. Zebrafish 10, 425–432. doi: 10.1089/zeb.2012.0862
Zang, L., Shimada, Y., Nishimura, Y., Tanaka, T., and Nishimura, N. (2015b). Repeated blood collection for blood tests in adult zebrafish. Jove J. Vis. Exp. 102:e53272. doi: 10.3791/53272
Zang, L., Shimada, Y., Tanaka, T., and Nishimura, N. (2015a). Rhamnan sulphate from Monostroma nitidum attenuates hepatic steatosis by suppressing lipogenesis in a diet-induced obesity zebrafish model. J. Funct. Foods 17, 364–370. doi: 10.1016/j.jff.2015.05.041
Zecchin, E., Filippi, A., Biemar, F., Tiso, N., Pauls, S., Ellertsdottir, E., et al. (2007). Distinct delta and jagged genes control sequential segregation of pancreatic cell types from precursor pools in zebrafish. Dev. Biol. 301, 192–204. doi: 10.1016/j.ydbio.2006.09.041
Keywords: zebrafish, obesity, diabetes, transgenic models, disease models, animal
Citation: Zang L, Maddison LA and Chen W (2018) Zebrafish as a Model for Obesity and Diabetes. Front. Cell Dev. Biol. 6:91. doi: 10.3389/fcell.2018.00091
Received: 02 April 2018; Accepted: 25 July 2018;
Published: 20 August 2018.
Edited by:
Ryan M. Anderson, Indiana University, Purdue University Indianapolis, United StatesReviewed by:
Marta Letizia Hribal, Università Degli Studi Magna Græcia di Catanzaro, ItalyAnne-Francoise Burnol, Institut National de la Santé et de la Recherche Médicale (INSERM), France
Copyright © 2018 Zang, Maddison and Chen. This is an open-access article distributed under the terms of the Creative Commons Attribution License (CC BY). The use, distribution or reproduction in other forums is permitted, provided the original author(s) and the copyright owner(s) are credited and that the original publication in this journal is cited, in accordance with accepted academic practice. No use, distribution or reproduction is permitted which does not comply with these terms.
*Correspondence: Wenbiao Chen, wenbiao.chen@Vanderbilt.Edu