Glycosaminoglycans: From Vascular Physiology to Tissue Engineering Applications
- 1Department of Biomedical Sciences, University of Sassari, Sassari, Italy
- 2Complex Tissue Regeneration Department, MERLN Institute for Technology Inspired Regenerative Medicine, Maastricht, Netherlands
Cardiovascular diseases represent the number one cause of death globally, with atherosclerosis a major contributor. Despite the clinical need for functional arterial substitutes, success has been limited to arterial replacements of large-caliber vessels (diameter > 6 mm), leaving the bulk of demand unmet. In this respect, one of the most challenging goals in tissue engineering is to design a “bioactive” resorbable scaffold, analogous to the natural extracellular matrix (ECM), able to guide the process of vascular tissue regeneration. Besides adequate mechanical properties to sustain the hemodynamic flow forces, scaffold’s properties should include biocompatibility, controlled biodegradability with non-toxic products, low inflammatory/thrombotic potential, porosity, and a specific combination of molecular signals allowing vascular cells to attach, proliferate and synthesize their own ECM. Different fabrication methods, such as phase separation, self-assembly and electrospinning are currently used to obtain nanofibrous scaffolds with a well-organized architecture and mechanical properties suitable for vascular tissue regeneration. However, several studies have shown that naked scaffolds, although fabricated with biocompatible polymers, represent a poor substrate to be populated by vascular cells. In this respect, surface functionalization with bioactive natural molecules, such as collagen, elastin, fibrinogen, silk fibroin, alginate, chitosan, dextran, glycosaminoglycans (GAGs), and growth factors has proven to be effective. GAGs are complex anionic unbranched heteropolysaccharides that represent major structural and functional ECM components of connective tissues. GAGs are very heterogeneous in terms of type of repeating disaccharide unit, relative molecular mass, charge density, degree and pattern of sulfation, degree of epimerization and physicochemical properties. These molecules participate in a number of vascular events such as the regulation of vascular permeability, lipid metabolism, hemostasis, and thrombosis, but also interact with vascular cells, growth factors, and cytokines to modulate cell adhesion, migration, and proliferation. The primary goal of this review is to perform a critical analysis of the last twenty-years of literature in which GAGs have been used as molecular cues, able to guide the processes leading to correct endothelialization and neo-artery formation, as well as to provide readers with an overall picture of their potential as functional molecules for small-diameter vascular regeneration.
Introduction
Cardiovascular diseases are a group of disorders affecting heart and blood vessels that represent a significant global health problem, being the leading cause of morbidity and mortality in the world. In 2019 acute clinical events, such as heart attack and stroke, mainly caused by the atherosclerosis of coronaries, carotid, and cerebral arteries, have been the cause of 16.2% and 11.6% of the global deaths, respectively (Data from the Global Burden of disease, https://vizhub.healthdata.org/gbd-compare/). Atherosclerosis is a chronic systemic inflammatory condition with a very complex etiology in which both genetic (e.g. hypertension, diabetes, obesity, high blood cholesterol and other lipids) and environmental (e.g. lifestyles including unhealthy diet, physical inactivity, smoking, alcohol abuse) risk factors play a role (Benjamin et al., 2017). Atherosclerosis is characterized by the accumulation of lipids and fibrous elements into the subendothelial space of arteries leading to plaque formation, which, ultimately, could evolve into an acute clinical event due to plaque rupture and thrombosis (Libby et al., 2019). There are many evidences demonstrating that high levels of plasma cholesterol-rich lipoproteins (LDLs) have a causal role in the early events leading to lesion formation (atherogenesis) (Ference et al., 2017). In this respect, it is known that some vascular extracellular matrix (ECM) components mediate their entrapment into the subendothelial space, thus leading to lipids accumulation within the arterial intima.
A major commitment of vascular tissue engineering is to design biomimetic scaffolds that combine the mechanical properties of natural blood vessels with biocompatibility, controlled biodegradability, and a specific combination of molecular signals allowing for vascular regeneration. Despite the clinical need for functional arterial substitutes, success has been limited to arterial replacement of large- and medium-caliber vessels with Dacron (polyethylene terephthalate) and Goretex (expanded polytetrafluoroethylene) synthetic grafts, leaving the bulk of demand unmet. In fact, for the replacement of small-diameter vessels (< 6 mm), such synthetic grafts are often rejected in a few months due to restenosis; hence, autologous veins (e.g. the great saphenous vein) or arteries (e.g. the internal mammary artery, and the radial artery) remain the best choice, despite their limited availability. To overcome these limitations, in the last decades, different tissue engineering approaches have been applied to develop vascular substitutes using natural, synthetic or hybrid materials, some of them reaching the preclinical/clinical stages (Liu et al., 2018).
Several attempts were performed to create completely biological living substitutes, such as collagen- or fibrin-based scaffolds in combination with vascular cells, sometimes with promising results (Peck et al., 2012). The approach of tissue engineering by self-assembly (TESA), allowing for the construction of an engineered blood vessel starting from autologous fibroblasts, obtained from skin biopsy, has been proven to be useful as arterial bypass grafts in long-term animal models (L'Heureux et al., 2006) as well as arteriovenous shunt in hemodialysis patients (L'Heureux et al., 2007). However, besides the high costs of fabrication, the long manufacturing time (i.e. few months) represents a limitation in patients who need rapid intervention. Interesting results were recently obtained by using acellular tissue engineered vessels (A-TEV) (Koobatian et al., 2016; Smith et al., 2019; Smith et al., 2020). This technology relies on decellularized natural matrices functionalized with bioactive molecules, thus providing host cells with a physiological environment. Issues remain about the total removal of xenogenic material and decellularizing agents that could elicit an immune response.
Several synthetic vascular grafts have been developed with the aim to guide vascular cells to attach, proliferate, and synthesize their own ECM, in which the inflammatory/thrombotic nature of the scaffolds could be overcome by coating them with bioactive natural molecules (Pashneh-Tala et al., 2016). Fabrication methods, such as phase separation, self-assembly and electrospinning, have been used to obtain biocompatible nanofibrous scaffolds with a well-organized architecture and mechanical properties suitable for vascular tissue regeneration (Yao et al., 2020). However, several evidences have shown that naked scaffolds for small-diameter blood vessel replacement suffer from low patency rates, are pro-thrombotic, susceptible to infection and do not have growth potential for the pediatric population (Song et al., 2018). Furthermore, they often represent a poor substrate to be populated by vascular cells (Awad et al., 2018). To overcome these issues, variously functionalized bioresorbable scaffolds have been developed as temporary guides for neo-artery formation. In this respect, surface functionalization with drugs or bioactive natural molecules, including ECM components such as collagen, elastin, or glycosaminoglycans (GAGs), and growth factors, has been proven to be effective in reducing thrombotic events, enhancing endothelialization, and further promoting cell proliferation.
Here, the composition of normal blood vessel and some pathophysiological roles of vascular proteoglycans (PGs) and GAGs have been discussed. Besides, the potential of GAGs as functional molecules for vascular tissue regeneration is the main topic of this review. In this respect, we performed an in-depth literature search, using MEDLINE (PubMed), with the aim of providing readers with the main findings of both in vitro and in vivo studies on the different small-diameter vascular devices, functionalized with GAGs, developed in the last 20 years.
Significance of Proteoglycans and Glycosaminoglycans in Vascular Biology
Blood Vessel Wall Structure
Blood vessels share some common features as they all consist of three concentric layers called tunica Intima, facing the vessel lumen, tunica Media and tunica Adventitia. The Intima is composed by a continuous monolayer of endothelial cells anchored to the basement membrane, which is involved in critical events such as blood coagulation, exchange of oxygen and nutrients, vascular tone regulation through mechanosensing (induction of endothelial nitric oxide synthesis by shear stress), inflammation, and immune response (Kruger-Genge et al., 2019). Most of the Intima’s functions are modulated by the endothelial glycocalyx, a dynamic and complex gel-like network on the luminal surface of endothelial cells, consisting of PGs (syndecans, glypicans), glycoproteins, GAGs (hyaluronan, heparan sulfate and chondroitin sulfate) and soluble proteins from both plasma and endothelium. The thickness (approximately 0.5–5.0 μm) and structure of the glycocalyx vary in relation to the vascular bed, blood flow rate, pathophysiological conditions, and they result from a dynamic balance between constant biosynthesis of new glycans and shear-dependent alterations, with a considerable rate of turnover of its components (Machin et al., 2019). Derangement of the endothelial glycocalyx structure plays major roles in several pathological conditions, including cardiovascular disease (Machin et al., 2019), diabetes (Dogne et al., 2018), kidney disease (Jourde-Chiche et al., 2019), sepsis (Uchimido et al., 2019), and trauma (Tuma et al., 2016). Underneath the tunica Intima there is the Media, a thick contractile multilayer of smooth muscle cells that provide support to the vessel as well as participate in regulating both blood flow and pressure. The Adventitia, with its collagenous ECM, is primarily responsible for the tensile strength of blood vessels. The three layers are separated from each other by an internal elastic lamina (between Intima and Media) and an external elastic lamina (between Media and Adventitia), consisting of fibers that provide elasticity to the vessel wall. In this scenario, the ECM in which vascular cells are immersed, mainly composed of collagens, elastin, fibronectin, laminins, glycoproteins, and PGs, not only provides the vascular wall with its mechanical properties, but also plays crucial roles in vascular cells homeostasis and pathogenesis. Therefore, the vascular wall is a very complex multilayer structure, in which different resident and circulating cell types interact with each other and with the ECM. Designing a substitute able to mimic the mechanical properties as well as the physiological functions of the vascular wall represents a challenge for tissue engineering.
Proteoglycans and Glycosaminoglycans of the Vascular Wall
PGs are hydrophilic molecules, which provide the vasculature with viscoelasticity and turgor. These molecules participate in a number of vascular events such as regulation of vascular permeability, lipid metabolism, hemostasis, and thrombosis, as well as interact with vascular cells, growth factors, and cytokines, in order to modify vascular cell adhesion, migration, and proliferation. Some PGs exert specific functions in their soluble forms following cleavage by proteolytic enzymes (Lepedda et al., 2021). The amount of PGs in the artery wall is physiologically low, but increases deeply during the early phases of atherosclerotic lesion formation. Many studies have shown that PGs are involved in retention of cholesterol-rich lipoproteins and other serum components, ECM metabolism, and crosstalk with inflammatory cells that extravasate to the subendothelial space. Readers are referred to the excellent review by Wight TN (Wight, 2018) for a more detailed discussion on this topic.
PGs have common structural features, as they all consist of a core protein covalently linked to one or more GAG chains, primarily responsible for their biological properties (Raman et al., 2005). GAGs are a family of anionic heteropolysaccharides that differ in terms of type of repeating disaccharide units, chains length, charge density, degree of sulfation, and hexuronic acid epimerization, found in connective tissues as well as in biological fluids such as plasma and urine (Vynios et al., 2002). The repeating disaccharide units are composed by a N-acetylated hexosamine (N-acetylgalactosamine or N-acetylglucosamine) and a hexuronic acid (glucuronic acid or its carbon-5 epimer iduronic acid) or galactose (in keratan sulfate). GAGs are key structural and functional components of the ECM of connective tissues, playing numerous biological roles, including embryonic development, ECM assembly and regulation of cell signaling in various physiological and pathological conditions. Indeed, their numerous functions are the result of their large structural heterogeneity, 202 unique disaccharide units have been identified in mammals (Clerc et al., 2019), responsible for the binding of a plethora of proteins including cytokines and chemokines, enzymes and enzyme inhibitors, ECM proteins, and membrane receptors (Kjellen and Lindahl, 2018). A comprehensive GAG-interactome composed of 827 proteins has been recently published (Vallet et al., 2021). Six main classes of GAGs have been described so far: hyaluronan or hyaluronic acid (HA), chondroitin sulfate (CS) and dermatan sulfate (DS), keratan sulfate (KS), heparan sulfate (HS), and heparin (Hep) (Figure 1); all GAG classes were found in normal and diseased arteries (Wight, 1980).
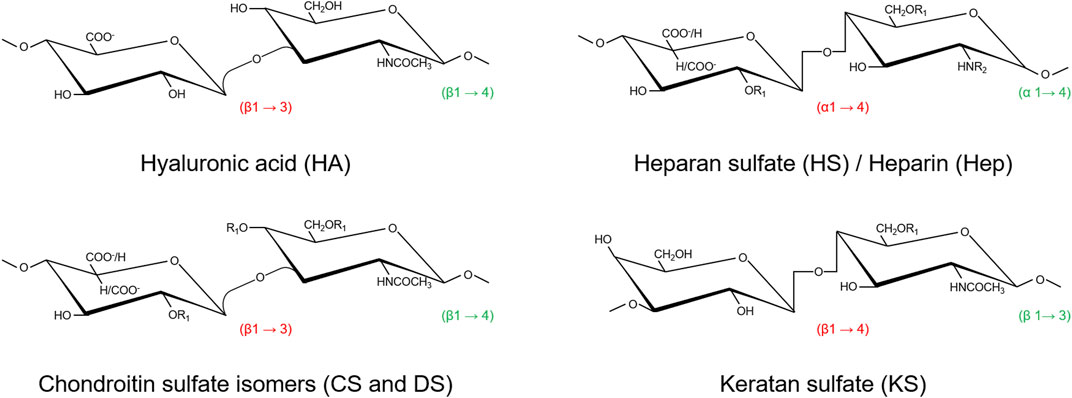
FIGURE 1. Structures of the repeating disaccharide units representative of the six GAGs classes [modified from (Vynios et al., 2002)]. Both DS and HS/Hep are co-polymers of two types of disaccharide repeats where glucuronate is variably substituted by its carbon-5 epimer iduronate. Hep has both a higher degree of sulfation and epimerization than HS. Except for HA, sulfation may occur in several positions, thus giving these glycans a characteristic high negative charge. Saccharides are reported as chair conformations. Configurations of the O-glycosidic bonds are reported in red (within disaccharide units) and in green (between adjacent disaccharide units). R1 = SO3−; R2 = COCH3/SO3−.
Besides HA, that is neither sulphated nor covalently linked to a protein core, GAGs together with their protein cores form distinct PG families. PGs can be found in the ECM, in the basement membrane (pericellular PGs), associated with the cell surface (transmembrane, GPI-anchored), or inside the cells (serglycin, the only currently known) (Iozzo and Schaefer, 2015). To date, more than 20 different PGs isoforms have been identified in normal and diseased blood vessels (Wight, 2018). In vascular tissue, versican is the main CS-PG (Yao et al., 1994), whereas DS chains are found linked to decorin and biglycan, two homologous small leucine rich repeat PGs (SLRPs) (Hultgardh-Nilsson et al., 2015). KS is present in fibromodulin and lumican, two more SLRPs (Hultgardh-Nilsson et al., 2015), whereas HS is the main GAG in perlecan (Kinsella and Wight, 2005), syndecans (Gopal, 2020), and glypicans (Filmus et al., 2008). Among them, versican, decorin, biglycan, as well as fibromodulin and lumican, are classified as extracellular PGs, perlecan is a pericellular PG, whereas syndecans and glypicans are localized on the cell surface (Theocharis et al., 2016) (Figure 2).
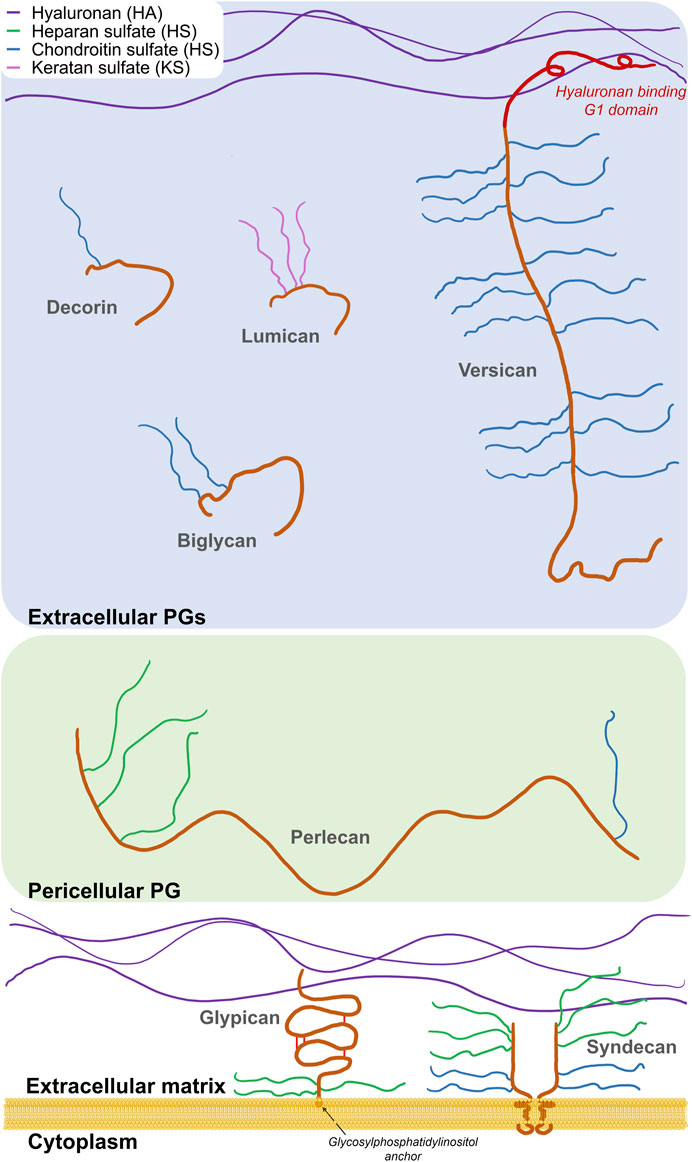
FIGURE 2. Schematic representation of the main vascular PGs according to their localization. Three main classes are reported: cell-associated, pericellular, and extracellular proteoglycans. Protein moieties are shown as orange backbones (versican G1 domain is highlighted in red).
With the exception of HA, GAGs are polymerized in the Golgi apparatus, starting from a tetrasaccharide linker consisting of xylose–galactose–galactose–uronic acid residues, by the sequential and repetitive addition of constituent monosaccharide residues. Post-synthetic chain modifications, such as epimerization of GlcA to iduronic acid and sulfation at specific positions, occur during polymerization, whereas selective removal of 6-O sulfates by specific sulfatases may occur in the extracellular space. Their structural heterogeneity, in terms of chain length, degree of iduronation, degree and pattern and sulfation, which is further increased by dynamic modifications in response to cellular and environmental stimuli, is so significant that, virtually, there are not two identical glycosaminoglycans in the body.
HA is a major constituent of the pericellular matrix and a key player in maintaining endothelial glycocalyx integrity, providing structural support and acting as signaling molecule by binding specific receptors on the cell surface. HA is synthesized by membrane-bound synthases as a high molecular weight GAG (up to 3–4 MDa), consisting of repeating residues of GlcNAc and GlcA linked each other by β-1,3- and β-1,4-glycosidic bonds. The synthesis takes place on the inner side of the plasma membrane and, during HA elongation, chains are extruded through pore-like structures to the cell surface without any further post-synthetic modifications (i.e. sulfation or epimerization) (Moretto et al., 2015). HA accumulates in aged vessels, stimulating vascular smooth muscle cells dedifferentiation and neointima formation (Moretto et al., 2015). Furthermore, during glycocalyx shedding, HA cleavage by hyaluronidase one into pro-inflammatory low molecular weight (<500 kDa) fragments contributes to endothelial derangement (Girish and Kemparaju, 2007; Vigetti et al., 2014).
Nanofibrous Scaffold Design for Vascular Tissue Engineering
A broad range of fabrication technologies are in use for the production of small diameter vascular grafts. In this section, the most used ones will be described, along with the materials used for their manufacture (Figure 3).
Solvent Casting
Solvent casting is one of the earliest and most widely used techniques. A liquid solution of the polymer of choice for the fabrication of the final vascular graft is poured into a cylindrical mold containing an inner rod. The solution is then solidified and the system is disassembled. If polymers are dissolved in volatile solvents, then the solution is cured and the solvent is left to evaporate. Thermally induced phase separation (TIPS) can be used to introduce porosity to the scaffold, in order to improve cell infiltration. Another strategy to further modulate scaffolds’ porosity is to freeze-dry the polymer solution, by which ice crystals are formed and then sublimated leaving a pore behind. The rate of temperature decrease can influence pore size and pore connectivity. By this approach, scaffolds of silk fibroin loaded with extracellular vesicles isolated from Adipose-derived Mesenchymal Stromal Cells (ADMSC) were fabricated (Cunnane et al., 2020). These grafts presented 100% patency at the early stages of the study (4 weeks), compared to the bare scaffold and scaffold seeded with ADMCS prior to implantation. To improve mechanical strength, the lyophilized silk fibroin scaffolds were reinforced with a layer of electrospun polycaprolactone (PCL) (Gupta et al., 2020).
Porous structures can be formed also by using so-called porogens, by the salt-leaching method. As an example, salt is introduced into the polymer solution, which is then cured and immersed in water to promote the dissolution of the salt, resulting in pore formation (Figure 4). This technique was used to fabricate, for example, elastin-like recombinamer (Fernández-Colino et al., 2019) and fast degrading poly(glycerol sebacate) (PGS) (Wu et al., 2012) scaffolds with promising results. Furthermore, the modification of these scaffolds with PGS derivatives with slower degradation kinetics were shown to improve outcomes in vivo (Fu et al., 2020).
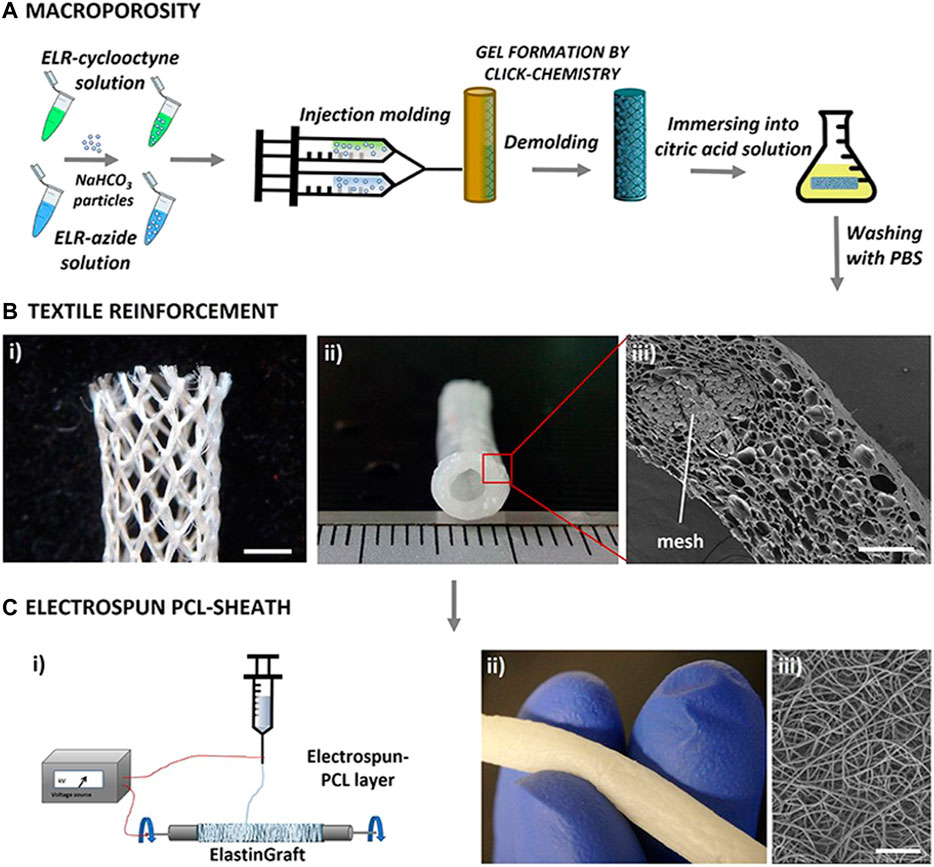
FIGURE 4. Fabrication method of porous tubular scaffolds from elastin-like recombinamers by salt leaching/gas foaming technique and electrospinning [modified from (Fernández-Colino et al., 2019)].
Another strategy to produce porous scaffolds is to use inverted colloid crystals. Microparticles of a material that is immiscible in the polymer of choice are fabricated, tightly packed in the molds, and then fused by annealing, before adding and curing the main polymer. Finally, the microparticles are dissolved, leaving a scaffold with highly interconnected pore network (Joao et al., 2014). While these techniques offer scalability and fast production, they fail to mimic the complexity of the ECM and the microenvironment in which cells are embedded.
Electrospinning
Electrospinning has emerged as one of the most prolific techniques to fabricate vascular grafts, because of its ability to form fibrous scaffolds that replicate the approximate diameter of ECM fibers and scalability. Fibers form on a grounded collecting mandrel, or static flat stage, when a polymer solution is ejected through a charged needle. The fibers obtained with this technique can range from the nano-to the microscale, depending on polymer concentration, flow rate, applied voltage, and air gap between the spinneret and the fabrication target. The rotation speed of the collecting mandrel can be tuned to obtain fibers oriented parallel to each other or circumferentially oriented, in a similar way to the ECM in the media layer (Uttayarat et al., 2010). At low (or no) rotational speeds, the fibers are deposited in a random manner (Figure 5).
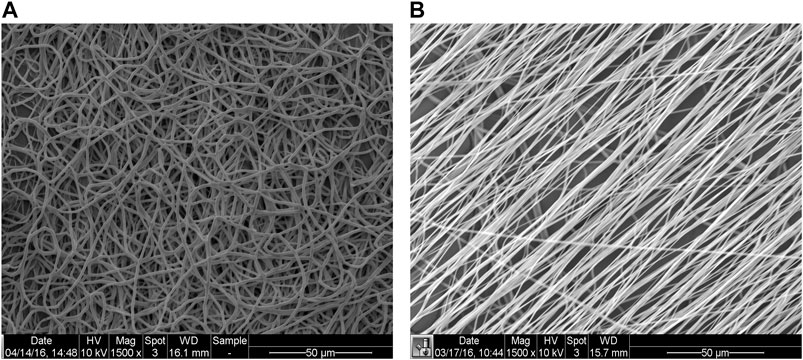
FIGURE 5. Representative scanning electron microscopy (SEM) images of random (mandrel speed = 500 rpm) and aligned (mandrel speed = 4000 rpm) PCL fibers (panels A and B, respectively) [modified from (Idini et al., 2019)].
Several polymers, either of natural (e.g. fibrinogen, collagens, silk fibroin, chitosan, alginate) or synthetic [e.g. polyurethane, PCL, polylactic acid, poly(propylene carbonate)] origin, have been investigated to produce matrices composed of nanofibers for biomedical applications (Agarwal et al., 2008). Among the former, silk fibroin represents a promising substrate for vascular tissue engineering applications, due to its mechanical properties, biocompatibility and slow degradation (Marelli et al., 2010; Catto et al., 2015; Marcolin et al., 2017).
Multimaterial scaffolds can be fabricated by mixing various polymers into one syringe, by simultaneously applying voltage to different needles or by sequentially spinning different materials. The porosity of scaffolds can be improved by co-electrospinning a polymer, such as PCL, gelatin or PGS, with polyvinyl alcohol, which can be dissolved and washed away after scaffold fabrication (Khosravi et al., 2016; Tan et al., 2016). More complex scaffolds can be obtained by sequential spinning of the same material with different molecular weight (de Valence et al., 2012) or by sequentially spinning different materials, such as PCL, elastin and collagen, to obtain a tri-layered graft (McClure et al., 2010). Often, more bioactive materials have weaker mechanical properties and, thus, synthetic materials have to be added to improve their mechanics. One way to implement this is by co-axial electrospinning in a core-shell manner, in which a strong polymer, such as PCL, is used in the core and a bioactive material, such as collagen, as the shell (Duan et al., 2016). Scaffolds can be mechanically reinforced also by electrospinning an outer layer of PCL, which acts as a sheath. By this approach, Elliott et al. fabricated aligned electrospun fibrin scaffolds with a coverage of PCL, to avoid suture rupture (Elliott et al., 2019).
To improve mechanical properties of electrospun scaffolds, hybrid approaches, combining electrospinning with fused deposition modeling (FDM) (Centola et al., 2010; Wu et al., 2020) or melt electrowriting (MEW) (Brown et al., 2011) were explored too. MEW combines electrospinning and FDM, by applying a voltage to a needle and grounding the collecting surface, mostly using molten polymers and avoiding the use of solvents. MEW has been used both to include reinforcements to electrospun meshes (Jungst et al., 2019) as well as to create standalone scaffolds (Saidy et al., 2020). Other techniques were combined with electrospinning for the fabrication of vascular grafts, including TIPS of thermoplastic polyurethane (Mi et al., 2016) or poly(ester-urethane)urea (Soletti et al., 2010), or braiding and TIPS of thermoplastic polyurethane and silk (Mi et al., 2015).
3D Printing
3D printing relies on the accurate deposition of material in the X-Y plane while also moving, gradually, in the Z direction. 3D printing allows users to input patient-specific data and can recreate the tortuosity and multiple branching of small caliber arteries, such as the coronaries. Techniques such as 2-photon (or multiphoton) polymerization or stereolithography use light to polymerize a resin material, in a bath, by using either a focused laser or a mask, and then illuminating the whole layer. Examples of materials used are methacrylated gelatin (GelMA), methacrylated HA (Thomas et al., 2020), polyethylene glycol diacrylate, polypropylene fumarate (Melchiorri et al., 2016), polyester urethane and poly (caprolactone-co-trimethylenecarbonate) diacrylate resins (Baudis et al., 2011; Kuhnt et al., 2019; Baker et al., 2020), methacrylated poly(ethylene glycol-co-depsipeptide) (Elomaa et al., 2015).
3D bioprinting allows the spatial distribution of different materials and cell populations. By co-axially bioprinting endothelial cells (ECs) in a fugitive ink (such as gelatin or Pluronics) in the inner core and GelMA or decellularized ECM hydrogel with smooth muscle cells (SMCs) on the outer shell and then liquefying the fugitive ink upon temperature switch, a hollow endothelialized structure was obtained (Cui et al., 2019; Gao et al., 2019). This approach was developed after initial work based on co-axial printing of endothelial cells in an alginate/ECM bioink in the shell and a core consisting of Ca-containing Pluoronics fugitive ink (Gao et al., 2018) (Figure 6).
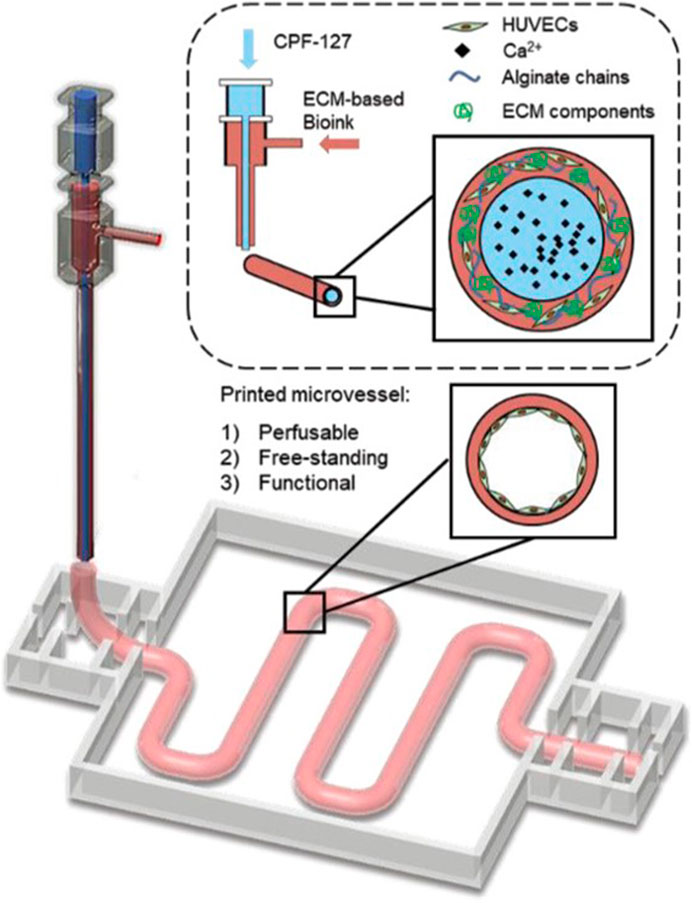
FIGURE 6. 3D bioprinting of vasculature using core-shell approach, where endothelial cells are suspended in an alginate-ECM bioink as the shell and the core is a fugitive bioink composed of Pluronic F127 containing Ca2+ ions (CPF127) [modified from (Gao et al., 2018)].
When using soft materials, such as hydrogels, traditional extrusion bioprinting might be unsuitable to create large constructs before they collapse. Strategies have been developed to overcome this limitation, by bioprinting inside a supporting bath consisting of the cross-linker and particles or gels that exhibit shear-thinning properties, which is discarded after printing (Hinton et al., 2015; Senior et al., 2019). These technique modifications have enabled the printing of large-scale cardiovascular constructs at high-resolution (Mirdamadi et al., 2020).
Cellular Self-Assembly
Trying to overcome issues related to both scaffolds’ biodegradation by the cells and undesired immune responses, scaffold-free strategies have been developed. These vascular grafts are produced by taking advantage of the ability of cells to self-assemble and secrete ECM.
Early work by L’Heureux demonstrated that long-term culture of fibroblasts produces an ECM that could be rolled into a tube able to withstand the physiological pulsatile flow (L'Heureux et al., 1998). Further studies showed this approach effective for arterial bypass grafting in long-term animal models (L'Heureux et al., 2006) as well as for arteriovenous shunt in hemodialysis patients (L'Heureux et al., 2007). However, a major downside of this strategy is the long culture period. The research team has recently modified its protocol by making threads from the ECM laid by the fibroblasts, which were knitted into tubes (L’Heureux, 2020; Magnan et al., 2020) (Figure 7). This method allows to produce a fully human tissue graft, with no foreign material.
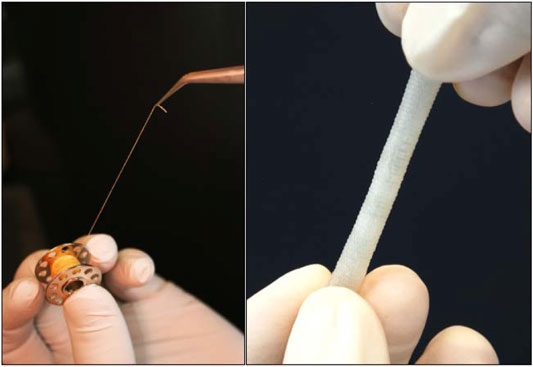
FIGURE 7. A thread (left) and a woven vessel (right) made from human Cell-assembled Extracellular Matrix [modified from (L’Heureux, 2020)].
Another approach to obtain implantable grafts was to seed SMCs onto fast degrading polyglycolic acid meshes and then to mature these constructs in flow bioreactors (Niklason et al., 1999; Niklason et al., 2001). To speed the process and avoid the need of harvesting autologous cells, the strategy was shifted to decellularizing the constructs once mature (Dahl et al., 2003). Such grafts were implanted in end-stage dialysis patients to treat arteriovenous fistulas (Lawson et al., 2016; Kirkton et al., 2019), as well as in patients with peripheral arterial disease (Gutowski et al., 2020). Exploiting the self-assembly approach, Itoh et al. produced hundreds of cell spheroids from a cell suspension of ECs, SMCs, and fibroblasts, and then placed them onto a needle array using a 3D printer printhead. Once the spheroids had fused into a continuous tissue, the needle array was removed and the construct placed in a perfusion reactor for maturation (Itoh et al., 2015). All these techniques require long culture times in highly controlled facilities, which makes these grafts expensive.
Decellularized Matrices
Decellularized vascular grafts theoretically mimic the content and structure of native arteries more accurately than synthetic constructs. Decellularization consists in removing all cellular content while trying to minimally disrupt the ECM, using physical, chemical and/or biological methods and agents. Arteries from cadaveric donors can be employed (Madden et al., 2004; Olausson et al., 2012). Alternatively, there is the possibility of using xenogenic blood vessels, which should have fewer “availability” problems. Decellularized porcine (Tillman et al., 2012), bovine (Daugs et al., 2017) and ovine (Mancuso et al., 2014) arteries have been used as vascular grafts (Figure 8).
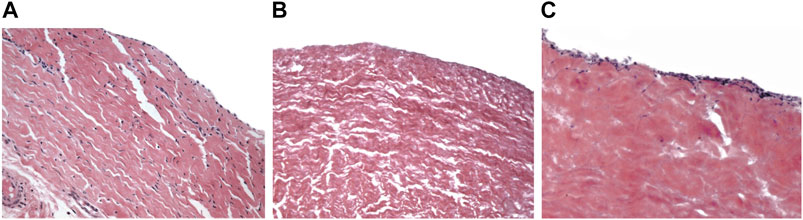
FIGURE 8. Hematoxylin and eosin staining of native bovine pericardium (A) and decellularized bovine pericardium before (B) and after (C) 7 days of culture with bovine fibroblasts. Magnification ×20 [from (Cigliano et al., 2012)].
Decellularized matrices can also be functionalized with bioactive molecules, thus facilitating the repopulation processes as well as reducing adverse events, such as restenosis and thrombosis. In this respect, interesting results were recently obtained by Andreadis’ team that implanted a cell-free small-diameter arterial graft based on small intestinal submucosa (SIS). After coating with Hep-bound vascular endothelial growth factor, implantation in the mouse abdominal aorta and in the sheep carotid artery, showed successful endothelialization. Notably, once integrated, both mechanical properties and vascular contractility of this graft were comparable to native arteries (Koobatian et al., 2016; Smith et al., 2019; Smith et al., 2020).
Although issues were raised on the risk of inflammatory/immune reactions elicited by cell debris, including DNA fragments (Zheng et al., 2005) and alpha-Gal epitopes (Naso et al., 2012), in the last years, decellularized matrices have been widely used in different fields of tissue regeneration. Currently, there is no consensus on the criteria to be used for the decellularization methods (Ji et al., 2019).
Application of Glycosaminoglycans to Vascular Tissue Engineering
Substitution of small-diameter vessels suffers of major problems regarding low-patency rate due to over-proliferation of smooth muscle cells (intimal hyperplasia) and thrombosis due to a lack of a functional endothelium lining the lumen of the graft. Hence, the need to design “smart substitutes” containing the chemical cues able to induce the population of the scaffold by host cells and the formation of a physiological artery wall.
Because of their pleiotropic functions and their physicochemical properties, GAGs have been extensively used in many tissue engineering applications including wound healing, as well as bone, cartilage, muscle, liver and nerve regeneration, as very recently reviewed by Sodhi H and Panitch A (Sodhi and Panitch, 2020). Furthermore, in the last 20 years, many studies have addressed the issues on small-diameter vascular regeneration by combining the most recent fabrication methods with the biofunctionalization with GAGs for the development of effective vascular grafts. We have tried to sum up the main findings of these substantial studies in two tables, according to the typology of GAG used, i.e. high and low molecular weight HA and HA derivatives (HYAFF-11) (Table 1), and Hep (Table 2). Also CS have been employed in a few studies for scaffolds functionalization (see below).
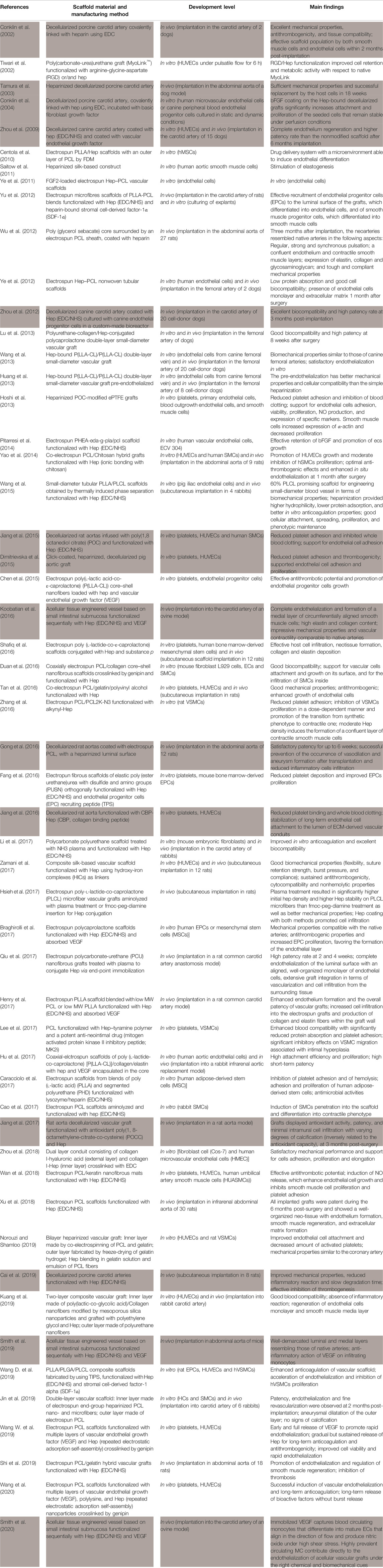
TABLE 2. Heparin-functionalized vascular scaffolds for small-caliber artery grafting. Acellular tissue engineered vessels (A-TEVs) (highlighted in gray).
Hyaluronan-Based Vascular Constructs
Since discovery in 1934, HA has been extensively studied, and thanks to its viscoelastic properties, physiological activity, and biocompatibility, it has been used in a wide range of medical fields from orthopedics to cosmetics (Abatangelo et al., 2020). Due to the high-water solubility and the propensity to form very viscous solutions, its use in vascular tissue engineering requires the crosslinking with other natural (e.g. type I collagen) or synthetic materials (e.g. PCL) or its chemical modification to obtain semisynthetic polymers (Table 1). Among the latter, HYAFF-11, an HA derivative obtained by esterification with benzyl alcohol, was proven to be useful for small-diameter vascular grafts fabrication, also in a large animal model. This polymer is biocompatible, bioresorbable, able to interact with polar molecules, and it can be processed to obtain several types of devices such as tubes, membranes, non-woven fabrics, gauzes, and sponges (Vindigni et al., 2009). HYAFF-11 tubes, obtained by coagulation of a HYAFF-11/DMSO solution around a cylindrical bar in an ethanol bath, were successfully implanted in the abdominal aorta of 30 rats (2 mm diameter, 1 cm length) (Lepidi et al., 2006a; Lepidi et al., 2006b), as well as in the carotid artery of 10 pigs (4 mm diameter, 5 cm length) (Zavan et al., 2008), as temporary absorbable guides to promote regeneration of vascular structures. These studies showed the potential of these HA-based grafts to guide the development of a functional neo-artery, consisting of a confluent endothelium lining the luminal surface and a vascular wall with organized layers of elastin fibers. Interesting in vitro results were also obtained with electrospun PCL or collagen type I scaffolds functionalized with either high or low molecular weight HA, showing excellent biocompatibility and the potential to guide the formation of a polarized functional endothelium (Table 1). Recently, Yuan et al. (Yuan et al., 2016) obtained highly aligned HA/PLLA nanofibers in core-shell structure by coaxial stable jet electrospinning, which was shown effective in inducing human umbilical arterial smooth muscle cells elongation, orientation, proliferation, and differentiation toward a contractile phenotype. Circumferentially aligned HA/PLLA nanofibers scaffolds were successfully implanted in the carotid artery of six rabbits, showing high patency and efficacious vascular regeneration, 6 weeks post-surgery (Yuan et al., 2016).
Heparin-Based Vascular Constructs
Since its discovery in 1916, Hep has been widely clinically used to treat thrombotic disorders, as it amplifies the inhibitory activity of antithrombin III toward thrombin by facilitating the formation of a ternary complex with factor Xa. Although Hep and HS share the same biosynthetic pathway, the former has higher degree of sulfation (2.6 vs. 0.6 sulfate groups per disaccharide) and epimerization (up to 90 vs. 20% iduronation) (Liu and Linhardt, 2014). Initially known for its anticoagulant properties, many evidences showed Hep’s usefulness as anti-inflammatory agent in the treatment of venous thromboembolism and other vascular diseases. Hep’s pleiotropic roles include the inhibitory activities toward neutrophils functions, endothelial activation and smooth muscle cells proliferation (Yang et al., 2012; Poterucha et al., 2017), well-known key events in atherogenesis and vascular graft failure. Due to its capacity to bind and release growth factors, such as VEGF and bFGF, and to modulate angiogenesis, together with its anti-thrombotic properties, Hep has been used to functionalize many different systems including hydrogels, films, and electrospun fibers (Aslani et al., 2020). Table 2 summarizes the multitude of studies, published in the last 20 years, that exploited the angiogenic regulatory activities of Hep for the development of effective small diameter vascular grafts. Among them, the great majority developed electrospun scaffolds made of exclusively synthetic (e.g. poly-Ɛ-caprolactone, poly-L-lactide-co-caprolactone, poly-L-lactic acid) or hybrid (containing collagen, silk, chitosan, keratin, etc.) mats functionalized with Hep, mainly via chemical crosslinking using N-Ethyl-N′-(3-dimethylaminopropyl) carbodiimide/N-hydroxy succinimide (EDC/NHS). Besides, acellular tissue engineered vessels (A-TEVs) consisting of vascular tissue (mainly carotid artery or aorta segments from pig, dog, or rat) or small intestinal submucosa, undergone a decellularization process and, subsequently, coated with Hep and, in some cases, angiogenic growth factors, have been fabricated.
Most of the reported studies, performed in vitro by culturing either endothelial cells or smooth muscle cells, showed good mechanical properties, enhanced biocompatibility and anti-thrombogenicity of these grafts, and the potential of Hep to promote endothelialization and smooth muscle cells differentiation toward a contractile phenotype. Besides, some interesting results were obtained in vivo with both synthetic grafts and A-TEVs.
Wu et al. (Wu et al., 2012) designed a cell-free fast-degrading elastomeric graft consisting of a PGS core surrounded by a PCL sheath, coated with Hep, which was successfully implanted in the abdominal aorta of 21 rats (porous PCL scaffolds were implanted in six rats as controls). The graft was rapidly substituted by a neo-artery with mechanical and functional properties very similar to those of the native one.
To improve endothelialization and reduce thrombotic events and restenosis, Song’s team developed two methods for optimal surface functionalization of synthetic grafts with Hep. In the first one, electrospun polycarbonate-urethane nanofibrous scaffolds underwent plasma treatment followed by Hep conjugation via end-point immobilization. Grafts were implanted in a rat common carotid artery anastomosis model, showing high patency rate and extensive graft integration (Qiu et al., 2017). In the second one, electrospun scaffolds were obtained using blends of high and low molecular weight elastomeric polymers to improve both functionalization and mechanical properties of the grafts. PLLA scaffolds with 5% low molecular weight PCL were functionalized with Hep, loaded with VEGF and implanted into the carotid artery of rats, evidencing a synergistic effect of these two angiogenic factors on graft patency, endothelialization, and neo-artery formation (Henry et al., 2017).
As above mentioned, Andreadis’ team designed an A-TEV from decellularized SIS, coated it sequentially with Hep and VEGF, and then implanted it in both mouse abdominal aorta and sheep carotid artery (Koobatian et al., 2016; Smith et al., 2019; Smith et al., 2020). They obtained promising results including complete endothelialization and formation of a medial layer of circumferentially aligned smooth muscle cells, high elastin and collagen content, impressive mechanical properties and vascular contractility comparable to native arteries. They showed that VEGF was essential for modulating the inflammatory response of monocytes and the regeneration of a functional artery wall (Smith et al., 2019). Additionally, they demonstrated that VEGF was able to capture circulating monocytes that differentiated into mature endothelial cells, therefore directly contributing to the endothelialization of acellular vascular grafts (Smith et al., 2020).
Chondroitin Sulfate-Based Vascular Constructs
Differently from both HA and Hep, only few in vitro studies dealt with usefulness of CS for functionalization of small-diameter vascular grafts. In particular, Lerouge’s team functionalized poly(ethylene terephthalate) scaffolds with CS (via EDC/NHS) and assessed their functionality culturing HUVECs and VSMCs from rat embryonic thoracic aorta. Overall, these studies showed that CS coating prevented platelet adhesion and activation, while promoting HUVECs growth and resistance to flow-induced shear stress, and survival and inward penetration of VSMCs (Thalla et al., 2014; Savoji et al., 2017). The paucity of these studies could be related with CS implications in atherogenesis. In fact, CS is a major GAG found in both normal vessels and atherosclerotic lesions that participates in apolipoprotein B100 binding, therefore contributing to lipids accumulation into the subendothelial space of arteries. These events are mediated by specific interactions between a proteoglycan-binding site in Apolipoprotein B100, consisting of a few basic amino acids, and the negatively charged CS (Boren et al., 1998), with increasing affinity for over-sulfated chains (Sambandam et al., 1991).
Conclusion
GAGs represent key players in vascular physiology as well as in the pathogenesis of atherosclerosis. A deep knowledge of GAGs structural complexity, which accounts for their numerous functions, still represents a challenge but also a huge potential source of information to pave the way to vascular tissue engineering. In this review, we have provided readers with an overview of the numerous efforts performed in the last 20 years, in the attempt to develop functional resorbable scaffolds for small-diameter vascular regeneration, using GAGs as molecular cues to guide the correct endothelialization of the luminal surface and neo-artery formation. Although many progresses have been obtained both in vitro and in vivo using HA, HA derivatives, or Hep as bioactive molecules, none of the mentioned devices has reached the clinical trial yet, leaving the field open to further studies, including those exploring the effects of specific sulfated saccharide sequences or synthetic glycans related molecules on vascular regeneration.
Author Contributions
AL, GN, JF-P, and LM contributed to manuscript conceptualization and data analysis. All authors contributed to manuscript writing, revision, read, and approved the submitted version.
Funding
This work is supported by the partners of Regenerative Medicine Crossing Borders (RegMed XB), a public-private partnership that uses regenerative medicine strategies to cure common chronic diseases. This collaboration project is financed by the Dutch Ministry of Economic Affairs by means of the PPP Allowance made available by the Top Sector Life Sciences and Health to stimulate public-private partnerships. AL, GN, and MF thank the University of Sassari for its financial support (Fondo di Ateneo per la Ricerca 2020).
Conflict of Interest
The authors declare that the research was conducted in the absence of any commercial or financial relationships that could be construed as a potential conflict of interest.
Acknowledgments
AL thanks Regione Autonoma della Sardegna for its financial support (POR-FSE 2014-2020-Asse Prioritario 3 “Istruzione e Formazione”-Obiettivo Tematico: 10, Priorità d’investimento: 10ii, Obiettivo Specifico: 10.5, Azione dell’Accordo di Partenariato 10.5.12-C.U.P. J86C18000270002). GN thanks “Programma Operativo Nazionale (PON) Ricerca e Innovazione 2014-2020—Asse I “Capitale Umano”, Azione I.2—A.I.M. Attraction and International Mobility, Linea 1 Mobilità dei ricercatori, AIM1874325-3, CUP: J54I18000110001” for its financial support.
References
Abatangelo, G., Vindigni, V., Avruscio, G., Pandis, L., and Brun, P. (2020). Hyaluronic Acid: Redefining its Role. Cells 9 (7). doi:10.3390/cells9071743
Agarwal, S., Wendorff, J. H., and Greiner, A. (2008). Use of Technique for Biomedical Applications. Polymer 49 (26), 5603–5621. doi:10.1016/j.polymer.2008.09.014
Arrigoni, C., Camozzi, D., Imberti, B., Mantero, S., and Remuzzi, A. (2006). The Effect of Sodium Ascorbate on the Mechanical Properties of Hyaluronan-Based Vascular Constructs. Biomaterials 27 (4), 623–630. doi:10.1016/j.biomaterials.2005.06.009
Aslani, S., Kabiri, M., HosseinZadeh, S., Hanaee-Ahvaz, H., Taherzadeh, E. S., and Soleimani, M. (2020). The Applications of Heparin in Vascular Tissue Engineering. Microvasc. Res. 131, 104027. doi:10.1016/j.mvr.2020.104027
Awad, N. K., Niu, H., Ali, U., Morsi, Y. S., and Lin, T. (2018). Electrospun Fibrous Scaffolds for Small-Diameter Blood Vessels: A Review. Membranes (Basel) 8 (1), 5. doi:10.3390/membranes8010015
Baker, M., Wang, R., Damanik, F., Kuhnt, T., Ippel, H., Dijkstra, P., et al. (2020). Biodegradable Poly(ester) Urethane Acrylate Resins for Digital Light Processing: From Polymer Synthesis to 3D Printed Tissue Engineering Constructs.
Baudis, S., Nehl, F., Ligon, S. C., Nigisch, A., Bergmeister, H., Bernhard, D., et al. (2011). Elastomeric Degradable Biomaterials by Photopolymerization-Based CAD-CAM for Vascular Tissue Engineering. Biomed. Mater. 6 (5), 055003. doi:10.1088/1748-6041/6/5/055003
Benjamin, E. J., Blaha, M. J., Chiuve, S. E., Cushman, M., Das, S. R., Deo, R., et al. (2017). Heart Disease and Stroke Statistics-2017 Update: A Report from the American Heart Association. Circulation 135 (10), e146–e603. doi:10.1161/cir.0000000000000485
Borén, J., Olin, K., Lee, I., Chait, A., Wight, T. N., and Innerarity, T. L. (1998). Identification of the Principal Proteoglycan-Binding Site in LDL. A Single-point Mutation in Apo-B100 Severely Affects Proteoglycan Interaction without Affecting LDL Receptor Binding. J. Clin. Invest. 101 (12), 2658–2664. doi:10.1172/jci2265
Braghirolli, D. I., Helfer, V. E., Chagastelles, P. C., Dalberto, T. P., Gamba, D., and Pranke, P. (2017). Electrospun Scaffolds Functionalized with Heparin and Vascular Endothelial Growth Factor Increase the Proliferation of Endothelial Progenitor Cells. Biomed. Mater. 12 (2), 025003. doi:10.1088/1748-605x/aa5bbc
Brown, T. D., Dalton, P. D., and Hutmacher, D. W. (2011). Direct Writing by Way of Melt Electrospinning. Adv. Mater. 23 (47), 5651–5657. doi:10.1002/adma.201103482
Cai, Z., Gu, Y., Cheng, J., Li, J., Xu, Z., Xing, Y., et al. (2019). Decellularization, Cross-Linking and Heparin Immobilization of Porcine Carotid Arteries for Tissue Engineering Vascular Grafts. Cell Tissue Bank 20 (4), 569–578. doi:10.1007/s10561-019-09792-5
Cao, J., Geng, X., Wen, J., Li, Q., Ye, L., Zhang, A., et al. (2017). The Penetration and Phenotype Modulation of Smooth Muscle Cells on Surface Heparin Modified Poly(ɛ-Caprolactone) Vascular Scaffold. J. Biomed. Mater. Res. 105 (10), 2806–2815. doi:10.1002/jbm.a.36144
Caracciolo, P. C., Rial-Hermida, M. I., Montini-Ballarin, F., Abraham, G. A., Concheiro, A., and Alvarez-Lorenzo, C. (2017). Surface-modified Bioresorbable Electrospun Scaffolds for Improving Hemocompatibility of Vascular Grafts. Mater. Sci. Eng. C. 75, 1115–1127. doi:10.1016/j.msec.2017.02.151
Catto, V., Farè, S., Cattaneo, I., Figliuzzi, M., Alessandrino, A., Freddi, G., et al. (2015). Small Diameter Electrospun Silk Fibroin Vascular Grafts: Mechanical Properties, In Vitro Biodegradability, and In Vivo Biocompatibility. Mater. Sci. Eng. C. 54, 101–111. doi:10.1016/j.msec.2015.05.003
Centola, M., Rainer, A., Spadaccio, C., De Porcellinis, S., Genovese, J. A., and Trombetta, M. (2010). Combining Electrospinning and Fused Deposition Modeling for the Fabrication of a Hybrid Vascular Graft. Biofabrication 2 (1), 014102. doi:10.1088/1758-5082/2/1/014102
Chen, X., Wang, J., An, Q., Li, D., Liu, P., Zhu, W., et al. (2015). Electrospun Poly(l-Lactic Acid-Co-Ɛ-Caprolactone) Fibers Loaded with Heparin and Vascular Endothelial Growth Factor to Improve Blood Compatibility and Endothelial Progenitor Cell Proliferation. Colloids Surf. B: Biointerfaces 128, 106–114. doi:10.1016/j.colsurfb.2015.02.023
Cigliano, A., Gandaglia, A., Lepedda, A. J., Zinellu, E., Naso, F., Gastaldello, A., et al. (2012). Fine Structure of Glycosaminoglycans from Fresh and Decellularized Porcine Cardiac Valves and Pericardium. Biochem. Res. Int. 2012, 979351. doi:10.1155/2012/979351
Clerc, O., Mariethoz, J., Rivet, A., Lisacek, F., Pérez, S., and Ricard-Blum, S. (2019). A Pipeline to Translate Glycosaminoglycan Sequences into 3D Models. Application to the Exploration of Glycosaminoglycan Conformational Space. Glycobiology 29 (1), 36–44. doi:10.1093/glycob/cwy084
Conklin, B. S., Richter, E. R., Kreutziger, K. L., Zhong, D.-S., and Chen, C. (2002). Development and Evaluation of a Novel Decellularized Vascular Xenograft. Med. Eng. Phys. 24 (3), 173–183. doi:10.1016/s1350-4533(02)00010-3
Conklin, B. S., Wu, H., Lin, P. H., Lumsden, A. B., and Chen, C. (2004). Basic Fibroblast Growth Factor Coating and Endothelial Cell Seeding of a Decellularized Heparin-Coated Vascular Graft. Artif. Organs 28 (7), 668–675. doi:10.1111/j.1525-1594.2004.00062.x
Cui, H., Zhu, W., Huang, Y., Liu, C., Yu, Z. X., Nowicki, M., et al. (2019). In vitro and In Vivo Evaluation of 3D Bioprinted Small-Diameter Vasculature with Smooth Muscle and Endothelium. Biofabrication 12 (1), 015004. doi:10.1088/1758-5090/ab402c
Cunnane, E. M., Lorentz, K. L., Ramaswamy, A. K., Gupta, P., Mandal, B. B., O’Brien, F. J., et al. (2020). Extracellular Vesicles Enhance the Remodeling of Cell-free Silk Vascular Scaffolds in Rat Aortae. ACS Appl. Mater. Inter. 12 (24), 26955–26965. doi:10.1021/acsami.0c06609
Dahl, S. L. M., Koh, J., Prabhakar, V., and Niklason, L. E. (2003). Decellularized Native and Engineered Arterial Scaffolds for Transplantation. Cel Transpl. 12 (6), 659–666. doi:10.3727/000000003108747136
Daugs, A., Hutzler, B., Meinke, M., Schmitz, C., Lehmann, N., Markhoff, A., et al. (2017). Detergent-Based Decellularization of Bovine Carotid Arteries for Vascular Tissue Engineering. Ann. Biomed. Eng. 45 (11), 2683–2692. doi:10.1007/s10439-017-1892-7
de Valence, S., Tille, J.-C., Giliberto, J.-P., Mrowczynski, W., Gurny, R., Walpoth, B. H., et al. (2012). Advantages of Bilayered Vascular Grafts for Surgical Applicability and Tissue Regeneration. Acta Biomater. 8 (11), 3914–3920. doi:10.1016/j.actbio.2012.06.035
Dimitrievska, S., Cai, C., Weyers, A., Balestrini, J. L., Lin, T., Sundaram, S., et al. (2015). Click-coated, Heparinized, Decellularized Vascular Grafts. Acta Biomater. 13, 177–187. doi:10.1016/j.actbio.2014.11.015
Dogné, S., Flamion, B., and Caron, N. (2018). Endothelial Glycocalyx as a Shield Against Diabetic Vascular Complications. Atvb 38 (7), 1427–1439. doi:10.1161/atvbaha.118.310839
Du, F., Zhao, W., Zhang, M., Mao, H., Kong, D., and Yang, J. (2011). The Synergistic Effect of Aligned Nanofibers and Hyaluronic Acid Modification on Endothelial Cell Behavior for Vascular Tissue Engineering. J. Nanosci. Nanotech. 11 (8), 6718–6725. doi:10.1166/jnn.2011.4750
Duan, N., Geng, X., Ye, L., Zhang, A., Feng, Z., Guo, L., et al. (2016). A Vascular Tissue Engineering Scaffold with Core-Shell Structured Nano-Fibers Formed by Coaxial Electrospinning and its Biocompatibility Evaluation. Biomed. Mater. 11 (3), 035007. doi:10.1088/1748-6041/11/3/035007
Elliott, M. B., Ginn, B., Fukunishi, T., Bedja, D., Suresh, A., Chen, T., et al. (2019). Regenerative and Durable Small-Diameter Graft as an Arterial Conduit. Proc. Natl. Acad. Sci. USA 116 (26), 12710–12719. doi:10.1073/pnas.1905966116
Elomaa, L., Pan, C.-C., Shanjani, Y., Malkovskiy, A., Seppälä, J. V., and Yang, Y. (2015). Three-dimensional Fabrication of Cell-Laden Biodegradable Poly(ethylene Glycol-Co-Depsipeptide) Hydrogels by Visible Light Stereolithography. J. Mater. Chem. B 3 (42), 8348–8358. doi:10.1039/c5tb01468a
Fang, J., Zhang, J., Du, J., Pan, Y., Shi, J., Peng, Y., et al. (2016). Orthogonally Functionalizable Polyurethane with Subsequent Modification with Heparin and Endothelium-Inducing Peptide Aiming for Vascular Reconstruction. ACS Appl. Mater. Inter. 8 (23), 14442–14452. doi:10.1021/acsami.6b04289
Ference, B. A., Ginsberg, H. N., Graham, I., Ray, K. K., Packard, C. J., Bruckert, E., et al. (2017). Low-density Lipoproteins Cause Atherosclerotic Cardiovascular Disease. 1. Evidence from Genetic, Epidemiologic, and Clinical Studies. A Consensus Statement from the European Atherosclerosis Society Consensus Panel. Eur. Heart J. 38 (32), 2459–2472. doi:10.1093/eurheartj/ehx144
Fernández-Colino, A., Wolf, F., Rütten, S., Schmitz-Rode, T., Rodríguez-Cabello, J. C., Jockenhoevel, S., et al. (2019). Small Caliber Compliant Vascular Grafts Based on Elastin-like Recombinamers for In Situ Tissue Engineering. Front. Bioeng. Biotechnol. 7, 340. doi:10.3389/fbioe.2019.00340
Filmus, J., Capurro, M., and Rast, J. (2008). Glypicans. Genome Biol. 9 (5), 224. doi:10.1186/gb-2008-9-5-224
Fu, J., Ding, X., Stowell, C. E. T., Wu, Y.-L., and Wang, Y. (2020). Slow Degrading Poly(glycerol Sebacate) Derivatives Improve Vascular Graft Remodeling in a Rat Carotid Artery Interposition Model. Biomaterials 257, 120251. doi:10.1016/j.biomaterials.2020.120251
Gao, G., Kim, H., Kim, B. S., Kong, J. S., Lee, J. Y., Park, B. W., et al. (2019). Tissue-engineering of Vascular Grafts Containing Endothelium and Smooth-Muscle Using Triple-Coaxial Cell Printing. Appl. Phys. Rev. 6 (4), 041402. doi:10.1063/1.5099306
Gao, G., Park, J. Y., Kim, B. S., Jang, J., and Cho, D. W. (2018). Coaxial Cell Printing of Freestanding, Perfusable, and Functional In Vitro Vascular Models for Recapitulation of Native Vascular Endothelium Pathophysiology. Adv. Healthc. Mater. 7 (23), e1801102. doi:10.1002/adhm.201801102
Girish, K. S., and Kemparaju, K. (2007). The Magic Glue Hyaluronan and its Eraser Hyaluronidase: A Biological Overview. Life Sci. 80 (21), 1921–1943. doi:10.1016/j.lfs.2007.02.037
Gong, W., Lei, D., Li, S., Huang, P., Qi, Q., Sun, Y., et al. (2016). Hybrid Small-Diameter Vascular Grafts: Anti-expansion Effect of Electrospun Poly ε-caprolactone on Heparin-Coated Decellularized Matrices. Biomaterials 76, 359–370. doi:10.1016/j.biomaterials.2015.10.066
Gopal, S. (2020). Syndecans in Inflammation at a Glance. Front. Immunol. 11, 227. doi:10.3389/fimmu.2020.00227
Gupta, P., Lorentz, K. L., Haskett, D. G., Cunnane, E. M., Ramaswamy, A. K., Weinbaum, J. S., et al. (2020). Bioresorbable Silk Grafts for Small Diameter Vascular Tissue Engineering Applications: In vitro and In Vivo Functional Analysis. Acta Biomater. 105, 146–158. doi:10.1016/j.actbio.2020.01.020
Gutowski, P., Gage, S. M., Guziewicz, M., Ilzecki, M., Kazimierczak, A., Kirkton, R. D., et al. (2020). Arterial Reconstruction with Human Bioengineered Acellular Blood Vessels in Patients with Peripheral Arterial Disease. J. Vasc. Surg. 72 (4), 1247–1258. doi:10.1016/j.jvs.2019.11.056
Henry, J. J. D., Yu, J., Wang, A., Lee, R., Fang, J., and Li, S. (2017). Engineering the Mechanical and Biological Properties of Nanofibrous Vascular Grafts for In Situ Vascular Tissue Engineering. Biofabrication 9 (3), 035007. doi:10.1088/1758-5090/aa834b
Hinton, T. J., Jallerat, Q., Palchesko, R. N., Park, J. H., Grodzicki, M. S., Shue, H. J., et al. (2015). Three-dimensional Printing of Complex Biological Structures by Freeform Reversible Embedding of Suspended Hydrogels. Sci. Adv. 1 (9), e1500758. doi:10.1126/sciadv.1500758
Hoshi, R. A., Van Lith, R., Jen, M. C., Allen, J. B., Lapidos, K. A., and Ameer, G. (2013). The Blood and Vascular Cell Compatibility of Heparin-Modified ePTFE Vascular Grafts. Biomaterials 34 (1), 30–41. doi:10.1016/j.biomaterials.2012.09.046
Hsieh, Y. F., Sahagian, K., Huang, F., Xu, K., Patel, S., and Li, S. (2017). Comparison of Plasma and Chemical Modifications of Poly-L-Lactide-Co-Caprolactone Scaffolds for Heparin Conjugation. Biomed. Mater. 12 (6), 065004. doi:10.1088/1748-605x/aa81aa
Hu, Y.-T., Pan, X.-D., Zheng, J., Ma, W.-G., and Sun, L.-Z. (2017). In vitro and In Vivo Evaluation of a Small-Caliber Coaxial Electrospun Vascular Graft Loaded with Heparin and VEGF. Int. J. Surg. 44, 244–249. doi:10.1016/j.ijsu.2017.06.077
Huang, C., Wang, S., Qiu, L., Ke, Q., Zhai, W., and Mo, X. (2013). Heparin Loading and Pre-endothelialization in Enhancing the Patency Rate of Electrospun Small-Diameter Vascular Grafts in a Canine Model. ACS Appl. Mater. Inter. 5 (6), 2220–2226. doi:10.1021/am400099p
Hultgårdh-Nilsson, A., Borén, J., and Chakravarti, S. (2015). The Small Leucine-Rich Repeat Proteoglycans in Tissue Repair and Atherosclerosis. J. Intern. Med. 278 (5), 447–461. doi:10.1111/joim.12400
Idini, M., Wieringa, P., Rocchiccioli, S., Nieddu, G., Ucciferri, N., Formato, M., et al. (2019). Glycosaminoglycan Functionalization of Electrospun Scaffolds Enhances Schwann Cell Activity. Acta Biomater. 96, 188–202. doi:10.1016/j.actbio.2019.06.054
Iozzo, R. V., and Schaefer, L. (2015). Proteoglycan Form and Function: A Comprehensive Nomenclature of Proteoglycans. Matrix Biol. 42, 11–55. doi:10.1016/j.matbio.2015.02.003
Itoh, M., Nakayama, K., Noguchi, R., Kamohara, K., Furukawa, K., Uchihashi, K., et al. (2015). Scaffold-Free Tubular Tissues Created by a Bio-3D Printer Undergo Remodeling and Endothelialization when Implanted in Rat Aortae. PLoS One 10 (9), e0136681. doi:10.1371/journal.pone.0136681
Ji, Y., Zhou, J., Sun, T., Tang, K., Xiong, Z., Ren, Z., et al. (2019). Diverse Preparation Methods for Small Intestinal Submucosa (SIS): Decellularization, Components, and Structure. J. Biomed. Mater. Res. A. 107 (3), 689–697. doi:10.1002/jbm.a.36582
Jia, W., Li, M., Kang, L., Gu, G., Guo, Z., and Chen, Z. (2019). Fabrication and Comprehensive Characterization of Biomimetic Extracellular Matrix Electrospun Scaffold for Vascular Tissue Engineering Applications. J. Mater. Sci. 54 (15), 10871–10883. doi:10.1007/s10853-019-03667-6
Jiang, B., Akgun, B., Lam, R. C., Ameer, G. A., and Wertheim, J. A. (2015). A Polymer-Extracellular Matrix Composite with Improved Thromboresistance and Recellularization Properties. Acta Biomater. 18, 50–58. doi:10.1016/j.actbio.2015.02.015
Jiang, B., Suen, R., Wang, J.-J., Zhang, Z. J., Wertheim, J. A., and Ameer, G. A. (2017). Vascular Scaffolds with Enhanced Antioxidant Activity Inhibit Graft Calcification. Biomaterials 144, 166–175. doi:10.1016/j.biomaterials.2017.08.014
Jiang, B., Suen, R., Wertheim, J. A., and Ameer, G. A. (2016). Targeting Heparin to Collagen within Extracellular Matrix Significantly Reduces Thrombogenicity and Improves Endothelialization of Decellularized Tissues. Biomacromolecules 17 (12), 3940–3948. doi:10.1021/acs.biomac.6b01330
Jin, X., Geng, X., Jia, L., Xu, Z., Ye, L., Gu, Y., et al. (2019). Preparation of Small-Diameter Tissue-Engineered Vascular Grafts Electrospun from Heparin End-Capped PCL and Evaluation in a Rabbit Carotid Artery Replacement Model. Macromol Biosci. 19 (8), e1900114. doi:10.1002/mabi.201900114
João, C. F. C., Vasconcelos, J. M., Silva, J. C., and Borges, J. P. (2014). An Overview of Inverted Colloidal Crystal Systems for Tissue Engineering. Tissue Eng. B: Rev. 20 (5), 437–454. doi:10.1089/ten.teb.2013.0402
Jourde-Chiche, N., Fakhouri, F., Dou, L., Bellien, J., Burtey, S., Frimat, M., et al. (2019). Endothelium Structure and Function in Kidney Health and Disease. Nat. Rev. Nephrol. 15 (2), 87–108. doi:10.1038/s41581-018-0098-z
Jungst, T., Pennings, I., Schmitz, M., Rosenberg, A. J. W. P., Groll, J., and Gawlitta, D. (2019). Heterotypic Scaffold Design Orchestrates Primary Cell Organization and Phenotypes in Cocultured Small Diameter Vascular Grafts. Adv. Funct. Mater. 29 (43), 1905987. doi:10.1002/adfm.201905987
Kang, L., Jia, W., Li, M., Wang, Q., Wang, C., Liu, Y., et al. (2019). Hyaluronic Acid Oligosaccharide-Modified Collagen Nanofibers as Vascular Tissue-Engineered Scaffold for Promoting Endothelial Cell Proliferation. Carbohydr. Polym. 223, 115106. doi:10.1016/j.carbpol.2019.115106
Khosravi, R., Best, C. A., Allen, R. A., Stowell, C. E. T., Onwuka, E., Zhuang, J. J., et al. (2016). Long-Term Functional Efficacy of a Novel Electrospun Poly(Glycerol Sebacate)-Based Arterial Graft in Mice. Ann. Biomed. Eng. 44 (8), 2402–2416. doi:10.1007/s10439-015-1545-7
Kinsella, M. G., and Wight, T. N. (2005). Perlecan: An Extracellular Matrix Heparan Sulfate Proteoglycan that Regulates Key Events in Vascular Development and Disease In Chemistry and Biology of Heparin and Heparan Sulfate. H. G. Garg, R. J. Linhardt, and C. A. Hales (Amsterdam; Elsevier Science), 607–635. doi:10.1016/b978-008044859-6/50023-x
Kirkton, R. D., Santiago-Maysonet, M., Lawson, J. H., Tente, W. E., Dahl, S. L. M., Niklason, L. E., et al. (2019). Bioengineered Human Acellular Vessels Recellularize and Evolve into Living Blood Vessels after Human Implantation. Sci. Transl Med. 11 (485), eaau6934. doi:10.1126/scitranslmed.aau6934
Kjellén, L., and Lindahl, U. (2018). Specificity of Glycosaminoglycan-Protein Interactions. Curr. Opin. Struct. Biol. 50, 101–108. doi:10.1016/j.sbi.2017.12.011
Koobatian, M. T., Row, S., Smith Jr, R. J., Koenigsknecht, C., Andreadis, S. T., and Swartz, D. D. (2016). Successful Endothelialization and Remodeling of a Cell-free Small-Diameter Arterial Graft in a Large Animal Model. Biomaterials 76, 344–358. doi:10.1016/j.biomaterials.2015.10.020
Kruger-Genge, A., Blocki, A., Franke, R. P., and Jung, F. (2019). Vascular Endothelial Cell Biology: An Update. Int. J. Mol. Sci. 20 (18), 4411. doi:10.3390/ijms20184411
Kuang, H., Yang, S., Wang, Y., He, Y., Ye, K., Hu, J., et al. (2019). Electrospun Bilayer Composite Vascular Graft with an Inner Layer Modified by Polyethylene Glycol and Haparin to Regenerate the Blood Vessel. J. Biomed. Nanotechnol 15 (1), 77–84. doi:10.1166/jbn.2019.2666
Kuhnt, T., Marroquín García, R., Camarero-Espinosa, S., Dias, A., ten Cate, A. T., van Blitterswijk, C. A., et al. (2019). Poly(caprolactone-co-trimethylenecarbonate) Urethane Acrylate Resins for Digital Light Processing of Bioresorbable Tissue Engineering Implants. Biomater. Sci. 7 (12), 4984–4989. doi:10.1039/c9bm01042d
L'Heureux, N., Dusserre, N., Konig, G., Victor, B., Keire, P., Wight, T. N., et al. (2006). Human Tissue-Engineered Blood Vessels for Adult Arterial Revascularization. Nat. Med. 12 (3), 361–365. doi:10.1038/nm1364
L'Heureux, N., McAllister, T. N., and de la Fuente, L. M. (2007). Tissue-engineered Blood Vessel for Adult Arterial Revascularization. N. Engl. J. Med. 357 (14), 1451–1453. doi:10.1056/nejmc071536
L'Heureux, N., Pâquet, S., Labbé, R., Germain, L., and Auger, F. A. (1998). A Completely Biological Tissue‐engineered Human Blood Vessel. FASEB j. 12 (1), 47–56. doi:10.1096/fsb2fasebj.12.1.47
Lawson, J. H., Glickman, M. H., Ilzecki, M., Jakimowicz, T., Jaroszynski, A., Peden, E. K., et al. (2016). Bioengineered Human Acellular Vessels for Dialysis Access in Patients with End-Stage Renal Disease: Two Phase 2 Single-Arm Trials. The Lancet 387 (10032), 2026–2034. doi:10.1016/s0140-6736(16)00557-2
Lee, Y., Le Thi, P., Seon, G. M., Ryu, S. B., Brophy, C. M., Kim, Y., et al. (2017). Heparin-functionalized Polymer Graft Surface Eluting MK2 Inhibitory Peptide to Improve Hemocompatibility and Anti-neointimal Activity. J. Controlled Release 266, 321–330. doi:10.1016/j.jconrel.2017.10.002
Lepedda, A. J., Nieddu, G., Piperigkou, Z., Kyriakopoulou, K., Karamanos, N., and Formato, M. (2021). Circulating Heparan Sulfate Proteoglycans as Biomarkers in Health and Disease. Semin. Thromb. Hemost. 47 (3), 295–307. doi:10.1055/s-0041-1725063
Lepidi, S., Abatangelo, G., Vindigni, V., Deriu, G. P., Zavan, B., Tonello, C., et al. (2006a). In vivo regeneration of Small‐diameter (2 Mm) Arteries Using a Polymer Scaffold. FASEB j. 20 (1), 103–105. doi:10.1096/fj.05-4802fje
Lepidi, S., Grego, F., Vindigni, V., Zavan, B., Tonello, C., Deriu, G. P., et al. (2006b). Hyaluronan Biodegradable Scaffold for Small-Caliber Artery Grafting: Preliminary Results in an Animal Model. Eur. J. Vasc. Endovascular Surg. 32 (4), 411–417. doi:10.1016/j.ejvs.2006.02.012
L’Heureux, N. (2020). Cell-assembled Extracellular Matrix (CAM) as a Biomaterial for Building Vascular Grafts. FASEB J. 34 (S1), 1. doi:10.1096/fsb2.v34.S1
Li, Q., Mu, L., Zhang, F., Mo, Z., Jin, C., and Qi, W. (2017). Manufacture and Property Research of Heparin Grafted Electrospinning PCU Artificial Vascular Scaffolds. Mater. Sci. Eng. C 78, 854–861. doi:10.1016/j.msec.2017.04.148
Libby, P., Buring, J. E., Badimon, L., Hansson, G. K., Deanfield, J., Bittencourt, M. S., et al. (2019). Atherosclerosis. Nat. Rev. Dis. Primers 5 (1), 56. doi:10.1038/s41572-019-0106-z
Liu, J., and Linhardt, R. J. (2014). Chemoenzymatic Synthesis of Heparan Sulfate and Heparin. Nat. Prod. Rep. 31 (12), 1676–1685. doi:10.1039/c4np00076e
Liu, R. H., Ong, C. S., Fukunishi, T., Ong, K., and Hibino, N. (2018). Review of Vascular Graft Studies in Large Animal Models. Tissue Eng. Part B: Rev. 24 (2), 133–143. doi:10.1089/ten.teb.2017.0350
Lu, G., Cui, S. J., Geng, X., Ye, L., Chen, B., Feng, Z. G., et al. (2013). Design and Preparation of Polyurethane-Collagen/heparin-Conjugated Polycaprolactone Double-Layer Bionic Small-Diameter Vascular Graft and its Preliminary Animal Tests. Chin. Med. J. (Engl) 126 (7), 1310–1316.
Machin, D. R., Phuong, T. T., and Donato, A. J. (2019). The Role of the Endothelial Glycocalyx in Advanced Age and Cardiovascular Disease. Curr. Opin. Pharmacol. 45, 66–71. doi:10.1016/j.coph.2019.04.011
Madden, R. L., Lipkowitz, G. S., Browne, B. J., and Kurbanov, A. (2004). Experience with Cryopreserved Cadaveric Femoral Vein Allografts Used for Hemodialysis Access. Ann. Vasc. Surg. 18 (4), 453–458. doi:10.1007/s10016-004-0055-0
Magnan, L., Labrunie, G., Fénelon, M., Dusserre, N., Foulc, M.-P., Lafourcade, M., et al. (2020). Human Textiles: A Cell-Synthesized yarn as a Truly "bio" Material for Tissue Engineering Applications. Acta Biomater. 105, 111–120. doi:10.1016/j.actbio.2020.01.037
Mancuso, L., Gualerzi, A., Boschetti, F., Loy, F., and Cao, G. (2014). Decellularized Ovine Arteries as Small-Diameter Vascular Grafts. Biomed. Mater. 9 (4), 045011. doi:10.1088/1748-6041/9/4/045011
Marcolin, C., Draghi, L., Tanzi, M., and Fare, S. (2017). Electrospun Silk Fibroin-Gelatin Composite Tubular Matrices as Scaffolds for Small Diameter Blood Vessel Regeneration. J. Mater. Sci. Mater. Med. 28 (5), 80. doi:10.1007/s10856-017-5884-9
Marelli, B., Alessandrino, A., Farè, S., Freddi, G., Mantovani, D., and Tanzi, M. C. (2010). Compliant Electrospun Silk Fibroin Tubes for Small Vessel Bypass Grafting. Acta Biomater. 6 (10), 4019–4026. doi:10.1016/j.actbio.2010.05.008
McClure, M. J., Sell, S. A., Simpson, D. G., Walpoth, B. H., and Bowlin, G. L. (2010). A Three-Layered Electrospun Matrix to Mimic Native Arterial Architecture Using Polycaprolactone, Elastin, and Collagen: a Preliminary Study. Acta Biomater. 6 (7), 2422–2433. doi:10.1016/j.actbio.2009.12.029
Melchiorri, A. J., Hibino, N., Best, C. A., Yi, T., Lee, Y. U., Kraynak, C. A., et al. (2016). 3D-Printed Biodegradable Polymeric Vascular Grafts. Adv. Healthc. Mater. 5 (3), 319–325. doi:10.1002/adhm.201500725
Mi, H.-Y., Jing, X., McNulty, J., Salick, M. R., Peng, X.-F., and Turng, L.-S. (2016). Approaches to Fabricating Multiple-Layered Vascular Scaffolds Using Hybrid Electrospinning and Thermally Induced Phase Separation Methods. Ind. Eng. Chem. Res. 55 (4), 882–892. doi:10.1021/acs.iecr.5b03462
Mi, H.-Y., Jing, X., Yu, E., McNulty, J., Peng, X.-F., and Turng, L.-S. (2015). Fabrication of Triple-Layered Vascular Scaffolds by Combining Electrospinning, Braiding, and Thermally Induced Phase Separation. Mater. Lett. 161, 305–308. doi:10.1016/j.matlet.2015.08.119
Mirdamadi, E., Tashman, J. W., Shiwarski, D. J., Palchesko, R. N., and Feinberg, A. W. (2020). FRESH 3D Bioprinting a Full-Size Model of the Human Heart. ACS Biomater. Sci. Eng. 6 (11), 6453–6459. doi:10.1021/acsbiomaterials.0c01133
Moretto, P., Karousou, E., Viola, M., Caon, I., D'Angelo, M. L., De Luca, G., et al. (2015). Regulation of Hyaluronan Synthesis in Vascular Diseases and Diabetes. J. Diabetes Res. 2015, 167283. doi:10.1155/2015/167283
Naso, F., Gandaglia, A., Iop, L., Spina, M., and Gerosa, G. (2012). Alpha-Gal Detectors in Xenotransplantation Research: a Word of Caution. Xenotransplantation 19 (4), 215–220. doi:10.1111/j.1399-3089.2012.00714.x
Niklason, L. E., Abbott, W., Gao, J., Klagges, B., Hirschi, K. K., Ulubayram, K., et al. (2001). Morphologic and Mechanical Characteristics of Engineered Bovine Arteries. J. Vasc. Surg. 33 (3), 628–638. doi:10.1067/mva.2001.111747
Niklason, L. E., Gao, J., Abbott, W. M., Hirschi, K. K., Houser, S., Marini, R., et al. (1999). Functional Arteries Grown In Vitro. Science 284 (5413), 489–493. doi:10.1126/science.284.5413.489
Norouzi, S. K., and Shamloo, A. (2019). Bilayered Heparinized Vascular Graft Fabricated by Combining Electrospinning and Freeze Drying Methods. Mater. Sci. Eng. C 94, 1067–1076. doi:10.1016/j.msec.2018.10.016
Olausson, M., Patil, P. B., Kuna, V. K., Chougule, P., Hernandez, N., Methe, K., et al. (2012). Transplantation of an Allogeneic Vein Bioengineered with Autologous Stem Cells: a Proof-Of-Concept Study. The Lancet 380 (9838), 230–237. doi:10.1016/s0140-6736(12)60633-3
Pandis, L., Zavan, B., Abatangelo, G., Lepidi, S., Cortivo, R., and Vindigni, V. (2010). Hyaluronan-based Scaffold for In Vivo Regeneration of the Rat Vena Cava: Preliminary Results in an Animal Model. J. Biomed. Mater. Res. A. 93 (4), 1289–1296. doi:10.1002/jbm.a.32626
Pandis, L., Zavan, B., Bassetto, F., Ferroni, L., Iacobellis, L., Abatangelo, G., et al. (2011). Hyaluronic Acid Biodegradable Material for Reconstruction of Vascular Wall: a Preliminary Study in Rats. Microsurgery 31 (2), 138–145. doi:10.1002/micr.20856
Pashneh-Tala, S., MacNeil, S., and Claeyssens, F. (2016). The Tissue-Engineered Vascular Graft-Past, Present, and Future. Tissue Eng. Part B: Rev. 22 (1), 68–100. doi:10.1089/ten.teb.2015.0100
Peck, M., Gebhart, D., Dusserre, N., McAllister, T. N., and L'Heureux, N. (2012). The Evolution of Vascular Tissue Engineering and Current State of the Art. Cells Tissues Organs 195 (1-2), 144–158. doi:10.1159/000331406
Pitarresi, G., Fiorica, C., Palumbo, F. S., Rigogliuso, S., Ghersi, G., and Giammona, G. (2014). Heparin Functionalized Polyaspartamide/polyester Scaffold for Potential Blood Vessel Regeneration. J. Biomed. Mater. Res. 102 (5), 1334–1341. doi:10.1002/jbm.a.34818
Poterucha, T. J., Libby, P., and Goldhaber, S. Z. (2017). More Than an Anticoagulant: Do Heparins Have Direct Anti-inflammatory Effects? Thromb. Haemost. 117 (3), 437–444. doi:10.1160/th16-08-0620
Qiu, X., Lee, B. L.-P., Ning, X., Murthy, N., Dong, N., and Li, S. (2017). End-point Immobilization of Heparin on Plasma-Treated Surface of Electrospun Polycarbonate-Urethane Vascular Graft. Acta Biomater. 51, 138–147. doi:10.1016/j.actbio.2017.01.012
Raman, R., Sasisekharan, V., and Sasisekharan, R. (2005). Structural Insights into Biological Roles of Protein-Glycosaminoglycan Interactions. Chem. Biol. 12 (3), 267–277. doi:10.1016/j.chembiol.2004.11.020
Remuzzi, A., Mantero, S., Colombo, M., Morigi, M., Binda, E., Camozzi, D., et al. (2004). Vascular Smooth Muscle Cells on Hyaluronic Acid: Culture and Mechanical Characterization of an Engineered Vascular Construct. Tissue Eng. 10 (5-6), 699–710. doi:10.1089/1076327041348347
Saidy, N. T., Shabab, T., Bas, O., Rojas-Gonzalez, D. M., Menne, M., Henry, T., et al. (2020). Melt Electrowriting of Complex 3D Anatomically Relevant Scaffolds. Front. Bioeng. Biotechnol. 8, 793. doi:10.3389/fbioe.2020.00793
Saitow, C., Kaplan, D. L., and Castellot, J. J. (2011). Heparin Stimulates Elastogenesis: Application to Silk-Based Vascular Grafts. Matrix Biol. 30 (5-6), 346–355. doi:10.1016/j.matbio.2011.04.005
Sambandam, T., Baker, J. R., Christner, J. E., and Ekborg, S. L. (1991). Specificity of the Low Density Lipoprotein-Glycosaminoglycan Interaction. Arterioscler Thromb. 11 (3), 561–568. doi:10.1161/01.atv.11.3.561
Savoji, H., Maire, M., Lequoy, P., Liberelle, B., De Crescenzo, G., Ajji, A., et al. (2017). Combining Electrospun Fiber Mats and Bioactive Coatings for Vascular Graft Prostheses. Biomacromolecules 18 (1), 303–310. doi:10.1021/acs.biomac.6b01770
Senior, J. J., Cooke, M. E., Grover, L. M., and Smith, A. M. (2019). Fabrication of Complex Hydrogel Structures Using Suspended Layer Additive Manufacturing (SLAM). Adv. Funct. Mater. 29 (49), 1904845. doi:10.1002/adfm.201904845
Shafiq, M., Jung, Y., and Kim, S. H. (2016). Covalent Immobilization of Stem Cell Inducing/recruiting Factor and Heparin on Cell-free Small-Diameter Vascular Graft for Acceleratedin Situtissue Regeneration. J. Biomed. Mater. Res. 104 (6), 1352–1371. doi:10.1002/jbm.a.35666
Shi, J., Chen, S., Wang, L., Zhang, X., Gao, J., Jiang, L., et al. (2019). Rapid Endothelialization and Controlled Smooth Muscle Regeneration by Electrospun Heparin‐loaded Polycaprolactone/gelatin Hybrid Vascular Grafts. J. Biomed. Mater. Res. 107 (6), 2040–2049. doi:10.1002/jbm.b.34295
Smith, R. J., Nasiri, B., Kann, J., Yergeau, D., Bard, J. E., Swartz, D. D., et al. (2020). Endothelialization of Arterial Vascular Grafts by Circulating Monocytes. Nat. Commun. 11 (1), 1622. doi:10.1038/s41467-020-15361-2
Smith, R. J., Yi, T., Nasiri, B., Breuer, C. K., and Andreadis, S. T. (2019). Implantation of VEGF‐functionalized Cell‐free Vascular Grafts: Regenerative and Immunological Response. FASEB j. 33 (4), 5089–5100. doi:10.1096/fj.201801856r
Sodhi, H., and Panitch, A. (2020). Glycosaminoglycans in Tissue Engineering: A Review. Biomolecules 11 (1). doi:10.3390/biom11010029
Soletti, L., Hong, Y., Guan, J., Stankus, J. J., El-Kurdi, M. S., Wagner, W. R., et al. (2010). A Bilayered Elastomeric Scaffold for Tissue Engineering of Small Diameter Vascular Grafts. Acta Biomater. 6 (1), 110–122. doi:10.1016/j.actbio.2009.06.026
Song, H.-H. G., Rumma, R. T., Ozaki, C. K., Edelman, E. R., and Chen, C. S. (2018). Vascular Tissue Engineering: Progress, Challenges, and Clinical Promise. Cell Stem Cell 22 (3), 340–354. doi:10.1016/j.stem.2018.02.009
Tamura, N., Nakamura, T., Terai, H., Iwakura, A., Nomura, S., Shimizu, Y., et al. (2003). A New Acellular Vascular Prosthesis as a Scaffold for Host Tissue Regeneration. Int. J. Artif. Organs 26 (9), 783–792.
Tan, Z., Wang, H., Gao, X., Liu, T., and Tan, Y. (2016). Composite Vascular Grafts with High Cell Infiltration by Co-electrospinning. Mater. Sci. Eng. C 67, 369–377. doi:10.1016/j.msec.2016.05.067
Thalla, P. K., Fadlallah, H., Liberelle, B., Lequoy, P., De Crescenzo, G., Merhi, Y., et al. (2014). Chondroitin Sulfate Coatings Display Low Platelet but High Endothelial Cell Adhesive Properties Favorable for Vascular Implants. Biomacromolecules 15 (7), 2512–2520. doi:10.1021/bm5003762
Theocharis, A. D., Skandalis, S. S., Gialeli, C., and Karamanos, N. K. (2016). Extracellular Matrix Structure. Adv. Drug Deliv. Rev. 97, 4–27. doi:10.1016/j.addr.2015.11.001
Thomas, A., Orellano, I., Lam, T., Noichl, B., Geiger, M.-A., Amler, A.-K., et al. (2020). Vascular Bioprinting with Enzymatically Degradable Bioinks via Multi-Material Projection-Based Stereolithography. Acta Biomater. 117, 121–132. doi:10.1016/j.actbio.2020.09.033
Tillman, B. W., Yazdani, S. K., Neff, L. P., Corriere, M. A., Christ, G. J., Soker, S., et al. (2012). Bioengineered Vascular Access Maintains Structural Integrity in Response to Arteriovenous Flow and Repeated Needle Puncture. J. Vasc. Surg. 56 (3), 783–793. doi:10.1016/j.jvs.2012.02.030
Tiwari, A., Salacinski, H. J., Punshon, G., Hamilton, G., and Seifalian, A. M. (2002). Development of a Hybrid Cardiovascular Graft Using a Tissue Engineering Approach 1. FASEB j. 16 (8), 791–796. doi:10.1096/fj.01-0826com
Tuma, M., Canestrini, S., Alwahab, Z., and Marshall, J. (2016). Trauma and Endothelial Glycocalyx. Shock 46 (4), 352–357. doi:10.1097/shk.0000000000000635
Turner, N. J., Kielty, C. M., Walker, M. G., and Canfield, A. E. (2004). A Novel Hyaluronan-Based Biomaterial (Hyaff-11) as a Scaffold for Endothelial Cells in Tissue Engineered Vascular Grafts. Biomaterials 25 (28), 5955–5964. doi:10.1016/j.biomaterials.2004.02.002
Uchimido, R., Schmidt, E. P., and Shapiro, N. I. (2019). The Glycocalyx: a Novel Diagnostic and Therapeutic Target in Sepsis. Crit. Care 23 (1), 16. doi:10.1186/s13054-018-2292-6
Uttayarat, P., Perets, A., Li, M., Pimton, P., Stachelek, S. J., Alferiev, I., et al. (2010). Micropatterning of Three-Dimensional Electrospun Polyurethane Vascular Grafts. Acta Biomater. 6 (11), 4229–4237. doi:10.1016/j.actbio.2010.06.008
Vallet, S. D., Clerc, O., and Ricard-Blum, S. (2021). Glycosaminoglycan-Protein Interactions: The First Draft of the Glycosaminoglycan Interactome. J. Histochem. Cytochem. 69 (2), 93–104. doi:10.1369/0022155420946403
Vigetti, D., Karousou, E., Viola, M., Deleonibus, S., De Luca, G., and Passi, A. (2014). Hyaluronan: Biosynthesis and Signaling. Biochim. Biophys. Acta (Bba) - Gen. Subjects 1840 (8), 2452–2459. doi:10.1016/j.bbagen.2014.02.001
Vindigni, V., Cortivo, R., Iacobellis, L., Abatangelo, G., and Zavan, B. (2009). Hyaluronan Benzyl Ester as a Scaffold for Tissue Engineering. Ijms 10 (7), 2972–2985. doi:10.3390/ijms10072972
Vynios, D. H., Karamanos, N. K., and Tsiganos, C. P. (2002). Advances in Analysis of Glycosaminoglycans: its Application for the Assessment of Physiological and Pathological States of Connective Tissues. J. Chromatogr. B Analyt Technol. Biomed. Life Sci. 781 (1-2), 21–38. doi:10.1016/s1570-0232(02)00498-1
Wan, X., Wang, Y., Jin, X., Li, P., Yuan, J., and Shen, J. (2018). Heparinized PCL/keratin Mats for Vascular Tissue Engineering Scaffold with Potential of Catalytic Nitric Oxide Generation. J. Biomater. Sci. Polym. Edition 29 (14), 1785–1798. doi:10.1080/09205063.2018.1504192
Wang, D., Wang, X., Li, X., Jiang, L., Chang, Z., and Li, Q. (2020). Biologically Responsive, Long-Term Release Nanocoating on an Electrospun Scaffold for Vascular Endothelialization and Anticoagulation. Mater. Sci. Eng. C 107, 110212. doi:10.1016/j.msec.2019.110212
Wang, D., Wang, X., Zhang, Z., Wang, L., Li, X., Xu, Y., et al. (2019). Programmed Release of Multimodal, Cross-Linked Vascular Endothelial Growth Factor and Heparin Layers on Electrospun Polycaprolactone Vascular Grafts. ACS Appl. Mater. Inter. 11 (35), 32533–32542. doi:10.1021/acsami.9b10621
Wang, S., Mo, X., Jiang, B. J., Gao, C. J., Qiu, H. S., Zhuang, Y. G., et al. (2013). Fabrication of Small-Diameter Vascular Scaffolds by Heparin-Bonded P(LLA-CL) Composite Nanofibers to Improve Graft Patency. Ijn 8, 2131–2139. doi:10.2147/ijn.s44956
Wang, W., Hu, J., He, C., Nie, W., Feng, W., Qiu, K., et al. (2015). Heparinized PLLA/PLCL Nanofibrous Scaffold for Potential Engineering of Small‐diameter Blood Vessel: Tunable Elasticity and Anticoagulation Property. J. Biomed. Mater. Res. 103 (5), 1784–1797. doi:10.1002/jbm.a.35315
Wang, W., Liu, D., Li, D., Du, H., Zhang, J., You, Z., et al. (2019). Nanofibrous Vascular Scaffold Prepared from Miscible Polymer Blend with Heparin/stromal Cell-Derived Factor-1 Alpha for Enhancing Anticoagulation and Endothelialization. Colloids Surf. B: Biointerfaces 181, 963–972. doi:10.1016/j.colsurfb.2019.06.065
Wight, T. N. (2018). A Role for Proteoglycans in Vascular Disease. Matrix Biol. 71-72, 396–420. doi:10.1016/j.matbio.2018.02.019
Wu, D. J., van Dongen, K., Szymczyk, W., Besseling, P. J., Cardinaels, R. M., Marchioli, G., et al. (2020). Optimization of Anti-kinking Designs for Vascular Grafts Based on Supramolecular Materials. Front. Mater. 7 (220). doi:10.3389/fmats.2020.00220
Wu, W., Allen, R. A., and Wang, Y. (2012). Fast-degrading Elastomer Enables Rapid Remodeling of a Cell-free Synthetic Graft into a Neoartery. Nat. Med. 18 (7), 1148–1153. doi:10.1038/nm.2821
Xu, Z., Gu, Y., Li, J., Feng, Z., Guo, L., Tong, Z., et al. (2018). Vascular Remodeling Process of Heparin-Conjugated Poly(ε-Caprolactone) Scaffold in a Rat Abdominal Aorta Replacement Model. J. Vasc. Res. 55 (6), 338–349. doi:10.1159/000494509
Yang, Z., Tu, Q., Wang, J., and Huang, N. (2012). The Role of Heparin Binding Surfaces in the Direction of Endothelial and Smooth Muscle Cell Fate and Re-endothelialization. Biomaterials 33 (28), 6615–6625. doi:10.1016/j.biomaterials.2012.06.055
Yao, L. Y., Moody, C., Schönherr, E., Wight, T. N., and Sandell, L. J. (1994). Identification of the Proteoglycan Versican in Aorta and Smooth Muscle Cells by DNA Sequence Analysis, In Situ Hybridization and Immunohistochemistry. Matrix Biol. 14 (3), 213–225. doi:10.1016/0945-053x(94)90185-6
Yao, T., Baker, M. B., and Moroni, L. (2020). Strategies to Improve Nanofibrous Scaffolds for Vascular Tissue Engineering. Nanomaterials (Basel) 10 (5). doi:10.3390/nano10050887
Yao, Y., Wang, J., Cui, Y., Xu, R., Wang, Z., Zhang, J., et al. (2014). Effect of Sustained Heparin Release from PCL/chitosan Hybrid Small-Diameter Vascular Grafts on Anti-thrombogenic Property and Endothelialization. Acta Biomater. 10 (6), 2739–2749. doi:10.1016/j.actbio.2014.02.042
Ye, L., Wu, X., Duan, H. Y., Geng, X., Chen, B., Gu, Y. Q., et al. (2012). The In Vitro and In Vivo Biocompatibility Evaluation of Heparin-Poly(ε‐caprolactone) Conjugate for Vascular Tissue Engineering Scaffolds. J. Biomed. Mater. Res. 100A (12), 3251–3258. doi:10.1002/jbm.a.34270
Ye, L., Wu, X., Mu, Q., Chen, B., Duan, Y., Geng, X., et al. (2011). Heparin-Conjugated PCL Scaffolds Fabricated by Electrospinning and Loaded with Fibroblast Growth Factor 2. J. Biomater. Sci. Polym. Ed. 22 (1-3), 389–406. doi:10.1163/092050610x487710
Yu, J., Wang, A., Tang, Z., Henry, J., Li-Ping Lee, B., Zhu, Y., et al. (2012). The Effect of Stromal Cell-Derived Factor-1α/heparin Coating of Biodegradable Vascular Grafts on the Recruitment of Both Endothelial and Smooth Muscle Progenitor Cells for Accelerated Regeneration. Biomaterials 33 (32), 8062–8074. doi:10.1016/j.biomaterials.2012.07.042
Yuan, H., Qin, J., Xie, J., Li, B., Yu, Z., Peng, Z., et al. (2016). Highly Aligned Core-Shell Structured Nanofibers for Promoting Phenotypic Expression of vSMCs for Vascular Regeneration. Nanoscale 8 (36), 16307–16322. doi:10.1039/c6nr05075a
Zamani, M., Khafaji, M., Naji, M., Vossoughi, M., Alemzadeh, I., and Haghighipour, N. (2017). A Biomimetic Heparinized Composite Silk-Based Vascular Scaffold with Sustained Antithrombogenicity. Sci. Rep. 7 (1), 4455. doi:10.1038/s41598-017-04510-1
Zavan, B., Vindigni, V., Lepidi, S., Iacopetti, I., Avruscio, G., Abatangelo, G., et al. (2008). Neoarteries Grown In Vivo Using a Tissue‐engineered Hyaluronan‐based Scaffold. FASEB j. 22 (8), 2853–2861. doi:10.1096/fj.08-107284
Zhang, J., Wang, J., Wei, Y., Gao, C., Chen, X., Kong, W., et al. (2016). ECM-mimetic Heparin Glycosamioglycan-Functionalized Surface Favors Constructing Functional Vascular Smooth Muscle Tissue In Vitro. Colloids Surf. B: Biointerfaces 146, 280–288. doi:10.1016/j.colsurfb.2016.06.023
Zheng, M. H., Chen, J., Kirilak, Y., Willers, C., Xu, J., and Wood, D. (2005). Porcine Small Intestine Submucosa (SIS) Is Not an Acellular Collagenous Matrix and Contains Porcine DNA: Possible Implications in Human Implantation. J. Biomed. Mater. Res. 73B (1), 61–67. doi:10.1002/jbm.b.30170
Zhou, J., Ying, H., Wang, M., Su, D., Lu, G., and Chen, J. (2018). Dual Layer Collagen-GAG Conduit that Mimic Vascular Scaffold and Promote Blood Vessel Cells Adhesion, Proliferation and Elongation. Mater. Sci. Eng. C 92, 447–452. doi:10.1016/j.msec.2018.06.072
Zhou, M., Liu, Z., Liu, C., Jiang, X., Wei, Z., Qiao, W., et al. (2012). Tissue Engineering of Small-Diameter Vascular Grafts by Endothelial Progenitor Cells Seeding Heparin-Coated Decellularized Scaffolds. J. Biomed. Mater. Res. 100B (1), 111–120. doi:10.1002/jbm.b.31928
Zhou, M., Liu, Z., Wei, Z., Liu, C., Qiao, T., Ran, F., et al. (2009). Development and Validation of Small-Diameter Vascular Tissue from a Decellularized Scaffold Coated with Heparin and Vascular Endothelial Growth Factor. Artif. Organs 33 (3), 230–239. doi:10.1111/j.1525-1594.2009.00713.x
Keywords: glycosaminoglycans, scaffolds, tissue engineering, vascular regeneration, vascular disease
Citation: Lepedda AJ, Nieddu G, Formato M, Baker MB, Fernández-Pérez J and Moroni L (2021) Glycosaminoglycans: From Vascular Physiology to Tissue Engineering Applications. Front. Chem. 9:680836. doi: 10.3389/fchem.2021.680836
Received: 15 March 2021; Accepted: 03 May 2021;
Published: 18 May 2021.
Edited by:
Laura Russo, Università di Milano-Bicocca, ItalyReviewed by:
MariaCristina Tanzi, Politecnico di Milano, ItalyIman Shabani, Amirkabir University of Technology, Iran
Copyright © 2021 Lepedda, Nieddu, Formato, Baker, Fernández-Pérez and Moroni. This is an open-access article distributed under the terms of the Creative Commons Attribution License (CC BY). The use, distribution or reproduction in other forums is permitted, provided the original author(s) and the copyright owner(s) are credited and that the original publication in this journal is cited, in accordance with accepted academic practice. No use, distribution or reproduction is permitted which does not comply with these terms.
*Correspondence: Lorenzo Moroni, l.moroni@maastrichtuniversity.nl