- Program in Vector-borne Disease, Department of Veterinary Microbiology and Pathology, Washington State University, Pullman, WA, United States
Understanding what influences the ability of some arthropods to harbor and transmit pathogens may be key for controlling the spread of vector-borne diseases. Arthropod immunity has a central role in dictating vector competence for pathogen acquisition and transmission. Microbial infection elicits immune responses and imparts stress on the host by causing physical damage and nutrient deprivation, which triggers evolutionarily conserved stress response pathways aimed at restoring cellular homeostasis. Recent studies increasingly recognize that eukaryotic stress responses and innate immunity are closely intertwined. Herein, we describe two well-characterized and evolutionarily conserved mechanisms, the Unfolded Protein Response (UPR) and the Integrated Stress Response (ISR), and examine evidence that these stress responses impact immune signaling. We then describe how multiple pathogens, including vector-borne microbes, interface with stress responses in mammals. Owing to the well-conserved nature of the UPR and ISR, we speculate that similar mechanisms may be occurring in arthropod vectors and ultimately impacting vector competence. We conclude this Perspective by positing that novel insights into vector competence will emerge when considering that stress-signaling pathways may be influencing the arthropod immune network.
Introduction
Among arthropods, the adaptation to blood-feeding is a life history trait that evolved independently at least 20 times (1). From the arthropod’s perspective, a hematophageous lifestyle has both benefits and drawbacks. Blood is a good source of proteins and lipids, which are necessary for development and egg production (2–4). However, blood-feeding comes with a variety of risks and stressors (5) including long periods between nutrient supplementation (6–13), thermal stress associated with the influx of a hot blood meal (5, 14–16), heme toxicity (17–30) and excess amounts of ions and water (31). Cells respond to acute environmental changes by activating stress responses that temporarily increase tolerance limits in adverse conditions and/or eliminate stressful stimuli. Being able to respond to stressful stimuli is an evolutionary advantage, which explains the highly conserved nature of cellular stress responses across eukaryotes (32–40).
For arthropods that transmit disease, another stressor is the presence of pathogens with incoming blood meals (41–44). Although vector-borne pathogens do not typically kill their arthropod vectors (45), infection does impart stress on the host by parasitizing nutrients, secreting toxic by-products and/or causing physical damage (46). For this reason, arthropod immune processes responding to infection are a key factor influencing vector competence (47–53). From mammals, it is now recognized that innate immunity is tightly intertwined with cellular stress responses and may represent an ancient mode of host defense against infection (32–34, 54–60). Whether stress-responses also intersect with arthropod immunity and how this may influence vector competence of blood-feeding arthropods is not known. Herein we briefly outline current knowledge of two well-characterized cellular stress response mechanisms, the Unfolded Protein Response (UPR) and the Integrated Stress Response (ISR) and discuss evidence that stress signaling impacts immunity. We then cite examples of cellular stress responses mediating outcomes at the host-pathogen interface in mammals and conclude that, given the well-conserved nature of the UPR and the ISR, similar crosstalk may be occurring in arthropods that would fundamentally impact vector competence.
Arthropod Innate Immune Signaling
Arthropod innate immune pathways are best characterized in the model organism Drosophila which are briefly summarized owing to space constraints. The Toll pathway is generally characterized as responding to Gram-positive bacteria and fungi, resulting in activation of Rel-family transcription factors Dorsal and Dif (Dorsal-related immunity factor) (61–64). The IMD (immune deficiency) pathway is analogous to the tumor necrosis factor receptor (TNFR) pathway in mammals (65) and is initiated by Gram-negative bacteria, although exceptions outside of Drosophila have been observed (66–71). The Janus Kinase (JAK)-signal transducers and activators of transcription (STAT) pathway, first described in mammals, is activated in the presence of bacterial or protozoan pathogens as well as more than 35 ligands, including interferons (IFN) and interleukins (IL) (72, 73). In arthropods, the JAK-STAT pathway is induced by the endogenous cytokine Unpaired (Upd) (74). Owing to space constraints, we refer readers to excellent reviews that comprehensively cover arthropod Toll, IMD and JAK-STAT signaling (65, 75, 76).
The Unfolded Protein Response
The UPR is a cellular stress response mechanism that is highly conserved across species, from single-celled eukaryotes to mammals (Figure 1). The UPR is triggered when the endoplasmic reticulum (ER) is under stress, which can result from a variety of stimuli such as oxidative stress, changes in temperature or pH, lack of nutrients or infection (32–34, 77–84). Such conditions can impart stress when protein-folding requirements exceed the processing capacity of the ER, causing an accumulation of unfolded proteins in the ER lumen. The UPR is activated through any combination of 3 transmembrane receptors: PERK (PKR-like ER kinase), ATF6 (activating transcription factor 6) or IRE1α (inositol-requiring enzyme 1α). In a non-stressed state, the negative regulator, BiP (binding immunoglobulin protein; also known as GRP78), keeps all three receptors in an inactive state by binding to them. Upon activation, BiP disassociates from the receptors, thereby activating signaling. Disassociation allows IRE1α and PERK to oligomerize and autophophorylate (85–87), whereas ATF6 is released for migration to the Golgi (88, 89). If homeostasis cannot be restored, the UPR will switch from pro-survival to proapoptotic outcomes (90).
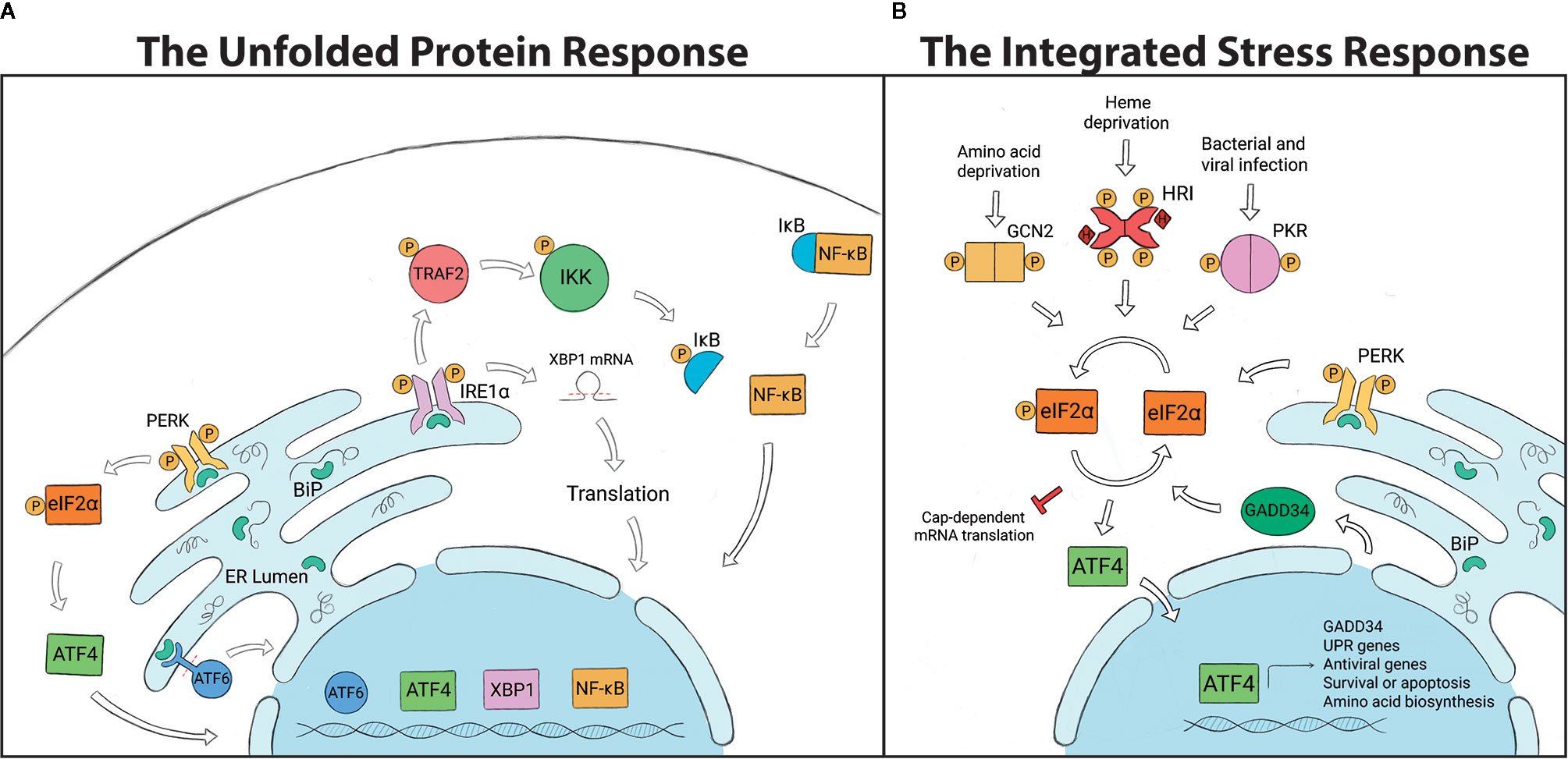
Figure 1 (A) The Unfolded Protein Response (UPR) is regulated by three transmembrane endoplasmic reticulum (ER) receptors: PERK, ATF6 and IRE1α. BiP binds to and holds the receptors in an inactive state under non-stressed conditions. The accumulation of unfolded proteins in the ER leads to the disassociation of BiP from the receptors and UPR activation. PERK phosphorylates eIF2α and induces ATF4, which controls transcription of chop and gadd34. ATF6 is cleaved and translocated to the nucleus to regulate expression of GRP94, p58IPK, and UPR-associated proteins. IRE1α splices xbp1, which is translated into a protein that controls genes involved in lipid biosynthesis, ER associated degradation pathway (ERAD) and chaperone production. IRE1α can also recruit TRAF2, which leads to NF-KB, ASK1 and JNK signaling. (B) The Integrated Stress Response (ISR) is initiated by stress-specific stimuli that activate kinases GCN2, HRI, PKR and PERK, which all converge on the regulatory molecule eIF2α. Phosphorylation of eIF2α halts global protein translation and upregulates the transcription factor ATF4, which determines cell fate. To terminate the ISR, ATF4 induces GADD34 expression which dephosphorylates eIF2α and allows global protein translation to resume.
PERK is a type I transmembrane protein kinase that has dual roles in the UPR as well as the ISR (91). When activated, PERK dimerizes, autophosphorylates and then also phosphorylates the regulatory molecule, eIF2α (eukaryotic translation initiation factor 2α). Phosphorylated eIF2α promotes cell survival by temporarily inhibiting protein translation, which decreases the amount of proteins entering the ER and alleviates the demand for protein folding. While inhibiting the translation of most mRNAs, eIF2α selectively induces the expression of some proteins including ATF4 (activating transcription factor 4). ATF4 can activate transcription of the growth arrest and DNA damage-inducible protein, GADD34, which negatively regulates eIF2α phosphorylation, or CHOP (C/EBP homologous protein), which is a proapoptotic factor (85, 92, 93).
ATF6 is a type II transmembrane protein that contains a bZIP transcription factor within the cytosolic domain. Once BiP disassociates from ATF6, it is transported to the Golgi compartment by COPII-containing vesicles (33, 94, 95). ATF6 is proteolytically processed by the Golgi-resident proteases S1P and S2P (site-1/2 proteases), which cleave the amino-terminal portion and allow the bZIP transcription factor to translocate to the nucleus (81, 94, 96). ATF6 upregulates the expression of GRP94 (endoplasmin) to increase the ER’s folding capacity and p58IPK to induce the ER associated degradation pathway (ERAD). ATF6 also induces the expression of other UPR-associated proteins including BiP and XBP1 (X-box binding protein) (32, 60, 97, 98).
IRE1α is a type I transmembrane protein with a cytosolic serine/threonine kinase domain and an RNase (ribonuclease) domain. When the ER is stressed, IRE1α autophosphorylates and splices the inactive mRNA xbp1U into xbp1S, which is then translated into a protein (33, 81, 99, 100). XBP1 translocates to the nucleus where it induces genes that are involved in lipid biosynthesis, ERAD and chaperone production (100, 101). IRE1α signaling also limits the amount of new proteins entering the ER through regulated IRE1α-dependent decay (RIDD), which degrades mRNA (102). With high levels of ER stress, IRE1α recruits the adaptor protein TRAF2 (TNF receptor-associated factor 2) a component of the TNFR pathway. IRE1α-TRAF2 association recruits the IKK complex, leading to NF-κB activation and proinflammatory responses (33, 103, 104). Alternatively, IRE1α-mediated signaling through TRAF2 can also lead to ASK1 (apoptosis signal regulating kinase 1; also known as mitogen-activated protein kinase 5, MAP3K5) activation and downstream JNK (JUN N-terminal kinase) signaling to induce apoptotic outcomes (33, 100, 104, 105). Less is known about UPR mechanisms in arthropods, but genome comparisons indicate that UPR-encoding genes are well-conserved between species, which may suggest similar mechanisms of action (Table 1).
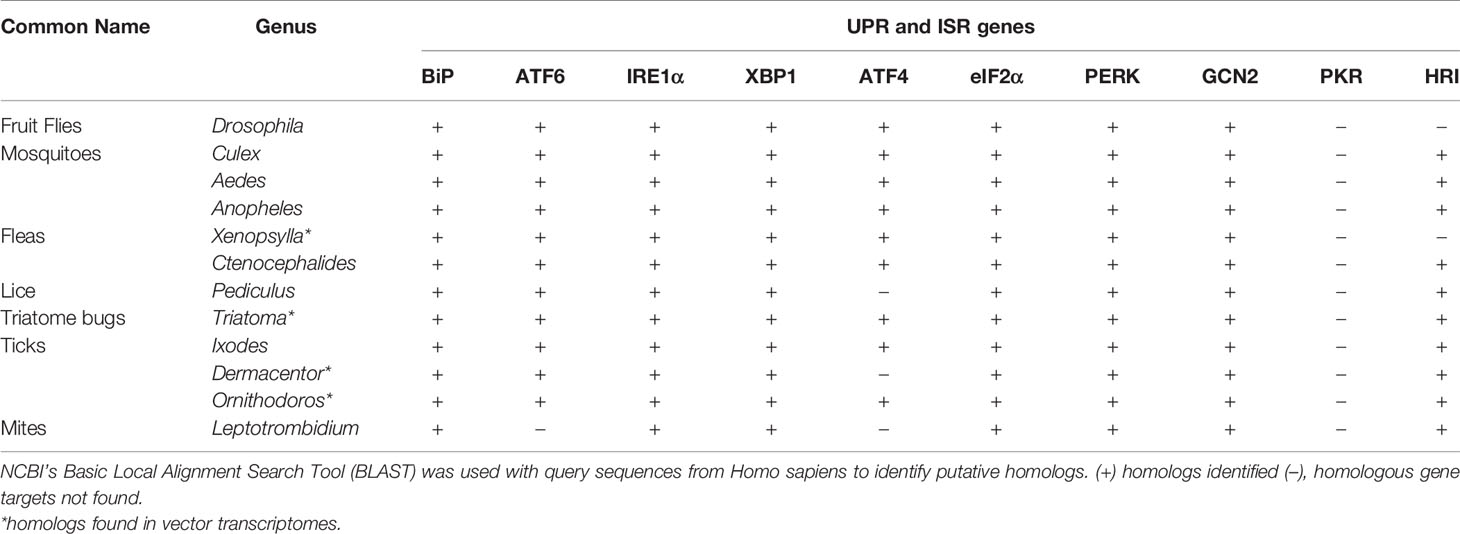
Table 1 Distribution of Unfolded Protein Response (UPR) and Integrated Stress Response (ISR) genes across arthropod vectors.
The Integrated Stress Response
The ISR is responsible for alleviating cellular stress and restoring homeostasis in eukaryotes (93, 106–109) (Figure 1). In mammalian cells, the ISR can be activated by one of four stress-sensing kinases: PKR (protein kinase double-stranded RNA-dependent), GCN2 (general control nonderepressible 2), HRI (heme-regulated inhibitor) and PERK (110, 111). These serine-threonine kinases are stimulated by pathological and physiological changes in the cellular environment. GCN2, a highly conserved cytoplasmic kinase, is stimulated by UV irradiation and nutrient deprivation (e.g. amino acid, glucose) (107, 108, 112). PERK is stimulated when misfolded proteins accumulate in the ER, causing ER stress (91, 113, 114). PKR is activated primarily in response to viral infections, as well as bacterial infections and oxidative stress (109, 115–117). Unlike the other kinases, HRI is mostly expressed in erythrocytes and acts as a heme sensor that is activated by iron deficiency (118).
All four stress-sensing kinases converge on a common regulatory factor: the phosphorylation of eIF2α (106, 110). Under non-stressed conditions, protein translation is initiated when the eIF2 complex (consisting of eIF2α, eIF2β and eIF2γ) binds with GTP and Met-tRNA (initiator methionyl tRNA). The ternary complex then associates with the 40S ribosome subunit to form the 43S pre‐initiation complex that binds to the 5’ end of mRNA and scans for start codons. Upon recognition, eIF2-GTP (active state) is hydrolyzed to eIF2-GDP (inactive state) and causes a conformational change to the pre-initiation complex, halting the mRNA scanning process and allowing protein translation to begin (119–123). eIF2B, a guanine nucleotide exchange factor, is essential for recycling GDP to GTP for new rounds of protein translation. Phosphorylated eIF2α attenuates protein synthesis owing to its increased affinity for eIF2B that prevents eIF2-GDP to eIF2-GTP exchange (110, 121–123).
As previously discussed in reference to PERK signaling, ATF4 is activated downstream of eIF2α phosphorylation and can act as both a transcriptional activator and repressor of genes important for determining cell fate, including chop and gadd34 (124–126). In response to prolonged ER-stress, ATF4 interacts with CHOP to generate reactive oxygen species (ROS), a key signal for mediating cell death (125). Once cellular stress is resolved, ATF4 induces GADD34 which interacts with protein phosphatase 1 (PP1) to dephosphorylate eIF2α and terminate the ISR (126).
The ISR has known roles in regulating host immunity. In response to ISR activation, cells will form cytoplasmic aggregates of untranslated mRNA and proteins termed “stress granules” that influence immune signaling. Phosphorylated eIF2α induces stress granules, which become cell signaling hubs that can intercept molecules from other pathways to modulate processes such as immunity (35, 127, 128). For example, TRAF2 is sequestered into stress granules, which ultimately suppresses NF‐κB-mediated inflammation (127). The ISR can also act as an antiviral defense mechanism (129–131). A stress granule-nucleating protein G3BP1 (Ras-GTPase-activating protein SH3 domain binding protein 1) recruits and activates PKR to suppress viral protein synthesis. It also activates innate immune responses through NF-κB and JNK pathways and promotes the expression of proinflammatory cytokines such as IL-17 (117). The arthropod ISR is less-studied when compared to mammals, but comparative genomic analyses demonstrate that many ISR genes are well-conserved in arthropod vectors (Table 1).
Interspecies Interactions
UPR-Pathogen Interface
The UPR is increasingly implicated in host defense against infection (132–137). For example, mammalian macrophages limit methicillin resistant Staphylococcus aureus (MRSA) through IRE1α with sustained ROS production and concentrated delivery of ROS to bacteria-containing phagosomes (138). The foodborne pathogen Camplyobacter jejuni activates eIF2α and CHOP, while decreasing expression of perk, ire1α and atf6 in human intestinal epithelial cells. Pharmacologically inducing the UPR prior to infection resulted in decreased C. jejuni invasion (139), highlighting the UPR as a host defense strategy.
Some pathogens manipulate or selectively induce the UPR to promote growth and survival. Legionella pneumophila, causative agent of Legionnaires’ disease, inhibits UPR-induced apoptosis with secreted effector proteins Lgt1 and Lgt2 that block IRE1α-mediated xbp1U splicing (140, 141). The intracellular pathogen Brucella manipulates the UPR in mammalian cells with secreted effectors TcpB and VceC (142–144), which induce IRE1α signaling and NF-кB to potentiate pro-inflammatory responses (81, 144–147). This effector-mediated manipulation appears to benefit the bacterium, with VceC providing an advantage for long-term in vivo colonization (144).
Vector-borne pathogens are also reported to cause ER stress and UPR activation in mammalian hosts. Francisella tularensis, causative agent of Tularemia vectored by ticks (Dermacentor spp. and Amblyomma americanum) and biting insects (148–151), alters the expression of bip, increases IRE1α phosphorylation and ATF6 activation, but decreases PERK phosphorylation and CHOP (152). IRE1α-XBP1 signaling is reported to limit F. tularensis in vivo with increased pathogen burdens in the liver, spleen and lungs of xbp1-/- mice (153). Orientia tsutsugamushi, the causative agent of Scrub Typhus transmitted by trombiculid mites (chiggers), induces the UPR and ERAD in HeLa cells to benefit bacterial growth, owing to the increase in available amino acids (154). The Ixodes scapularis-transmitted bacterium Anaplasma phagocytophilum induces all the three UPR branches in THP-1 cells (155).
Due to their very nature, viral replication requires host cells for protein production, which often engages the UPR. For example, herpesviruses activate one or more of the UPR receptors, but limit downstream signaling to ensure global protein translation, including viral proteins, is not halted (156–158). Many arthropod-transmitted viruses activate one or more UPR sensors in mammalian cells as well. Bluetongue virus, transmitted by Culicoides spp. (biting midges), induces autophagy to benefit viral replication by activating PERK-eIF2α signaling of the UPR (159). Mosquito-transmitted viruses Chikungunya (CHIKV), Dengue (DENV) and West Nile (WNV) all activate one or more UPR sensors in mammalian cells. CHIKV activates BiP and the ATF6 and IRE1α branches of the UPR, but blocks PERK signaling by suppressing eIF2α phosphorylation through the nonstructural viral protein nsP4 (160, 161). The flaviviruses DENV and WNV induce xbp1U splicing, ATF6 proteolysis, and eIF2α phosphorylation to benefit viral propagation in mammalian cells and, in the case of WNV, to inhibit type I IFN signaling (161–164). Tick-borne encephalitis virus (TBEV) and Langat virus (LGTV) are Ixodid-transmitted flaviviruses. TBEV infection activates the IRE1α and ATF6 pathways to facilitate viral replication (165). In contrast, while LGTV infection activates the UPR, PERK signaling restricts viral load (166), highlighting the importance of the UPR as a host defense mechanism.
Much less is known about the UPR-pathogen interface in arthropod vectors. Recent work shows differential regulation of ER-resident proteins involved in ERAD in Borrelia burgdorferi-infected adult I. scapularis (167). There is also evidence that arthropod-transmitted plant pathogens manipulate the UPR in their arthropod vectors. Candidatus Liberibacter asiaticus (CLas), causative agent of Asian citrus greening disease, is vectored by the Asian citrus psyllid Diaphorina citri Kuwayama (D. citri). Infection of D. citri with CLas upregulates expression of ERAD and UPR components. A related bacterium, Candidatus Liberibacter solanacerarum, also upregulates ire1α and multiple genes involved in ERAD in its arthropod vector, the potato psyllid (Bactericera cockerelli) (168). Although more work is required to understand what role the UPR and ERAD-mediated protein degradation have during arthropod infection, these studies demonstrate that vector-borne pathogens are interfacing with the UPR in arthropods.
ISR-Pathogen Interface
The ISR broadly responds to a variety of stress-inducing stimuli, including invasion and damage caused by infection. Listeria monocytogenes-infected mammalian cells activate PERK, ATF4, eIF2α, PKR and induce the expression of CHOP. L. monocytogenes secretes listeriolysin O (LLO), which is a pore-forming hemolysin required for phagosomal escape and bacterial survival. Cells treated with L. monocytogenes LLO resulted in a similar ISR activation phenotype as infection, indicating that this effector is partially responsible for inducing the ISR. Downstream production of type I IFN activates the ISR kinase PKR, increasing its expression and activation, and further stimulating eIF2α signaling (169). Epithelial cell infection with Shigella flexneri disrupts host cell membranes, causing GCN2-mediated eIF2α phosphorylation. This halts global protein translation, leading to stress granule formation and autophagy that eliminates bacteria (170).
These examples reflect the role of the ISR as a host-defense strategy against infection. In response, many pathogens have evolved methods to counteract ISR-mediated defenses. For example, the causative agent of Q fever, Coxiella burnetii, increases eIF2α phosphorylation in a Type IV Secretion System-dependent manner and induces ATF4 and CHOP in human macrophages. However, nuclear translocation of CHOP is blocked by C. burnetii to prevent ER stress-induced apoptosis (171). Coronavirus protein AcP10 and picornavirus protein AiVL promote viral protein synthesis by acting as competitive inhibitors for phosphorylated-eIF2 and eIF2B interactions (172). Excellent reviews summarizing ISR-mediated antiviral responses, including stress granules, and concurrent viral evasion strategies have been published in the past several years which readers are referred to (129, 173).
Interactions between vector-borne pathogens and ISR mechanisms have been reported, with several focusing on insect-borne viruses. In mammalian cells, the sandfly fever Sicilian phlebovirus evades PKR defense mechanisms by expressing a nonstructural protein that binds to eIF2B, blocking translation inhibition and promoting viral replication (174). Rift valley fever virus, transmitted by mosquitoes and sandflies, degrades host PKR and inhibits IFN induction (175–177). WNV inhibits PKR activation and downstream phosphorylation of eIF2α and stress granule formation (178). Zika virus likewise has evolved to evade stress granule formation in host cells by repurposing host proteins, including G3BP1, to facilitate viral replication and repress normal stress granule assembly (179, 180). Other flaviviruses such as WNV, DENV and Japanese encephalitis virus hijack or further inhibit stress granule machinery to benefit replication (181–184). These studies illustrate that vectored pathogens evade ISR signaling to facilitate replication and survival.
Concluding Remarks
Vector-borne pathogens selectively interface with the UPR and the ISR to promote survival and infection in mammals. Given the well-conserved nature of both the UPR and the ISR between evolutionarily distant species (Table 1), it is reasonable to speculate that vectored microbes may also be modulating the stress responses in their arthropod vectors. Moreover, this type of manipulation may be a common survival strategy used by vector-borne pathogens to suppress host defenses and create replicative niches.
Cellular stress responses are increasingly recognized as being closely intertwined with innate immunity (32–34, 54–60). For example, there are multiple reports that mammalian Toll-like receptors (TLRs) influence the UPR and the ISR. TLR2 and TLR4 in mammalian macrophages activate the IRE1α-XBP1 axis, which leads to proinflammatory TNFα and IL-6 cytokine production (153). MRSA induces xbp1U splicing in wildtype bone marrow-derived macrophages, but not in TLR2/4/9-/- or myd88-/- mutant cells, indicating that TLR signaling is required for IRE1α activation (138). The ISR kinase PKR is activated downstream from TLR3 and TLR4, which induces type I IFN (185, 186). Whether a similar phenomenon occurs in arthropods is not known, but considering the well-conserved nature of the Toll pathway between arthropods and mammals (70, 187) it is possible that this type of crosstalk is occurring across species.
Beyond TLR signaling, overlap between the UPR and ISR with other innate immunity components has also been noted. During infection, the mammalian UPR is capable of initiating an immune response by crosstalking with the TNFR pathway (33, 56–58, 188–190). IRE1α can produce proinflammatory responses by signaling through TRAF2, a component of the TNFR pathway, recruiting the IKK complex for phosphorylation and releasing NF-κB for nuclear translocation (32–34). Arthropod immunity may similarly be influenced by the UPR, as the arthropod IMD pathway is analogous to the mammalian TNFR network. PERK was shown to interact with JAK-STAT signaling in mammalian glial cells (191) and several examples of IFN production being influenced by the ISR and UPR have been reported, which could potentially influence JAK-STAT signaling in an indirect manner (169, 175–177, 185, 186, 192). Similar crosstalk between JAK-STAT and cellular stress responses may also be occurring in arthropod vectors.
Since one of the major factors determining vector competence is arthropod immunity (47–53), it is feasible that cellular stress responses may be influencing vector competence. With this in mind, understanding how stress responses may interface with arthropod immunity and conversely how vector-borne pathogens may be inducing or manipulating cellular stress responses could be important for opening new avenues in vector-borne disease control. This knowledge could be leveraged for the future design of disease transmission-blocking strategies to reduce the global burden of vector-borne diseases.
Data Availability Statement
Publicly available datasets were analyzed in this study. This data can be found on NCBI.
Author Contributions
KR, LS-L, JH, and DS wrote this perspective. EF designed and constructed Figure 1 and contributed to intellectual discussions. All authors contributed to the article and approved the submitted version.
Funding
This work was supported by National Institute of Health grants GM008336 to JH and R21AI139772, R21AI148578 and Washington State University to DKS. The content is solely the responsibility of the authors and does not necessarily represent the official views of the National Institute of Allergy and Infectious Diseases or the National Institutes of Health.
Conflict of Interest
The authors declare that the research was conducted in the absence of any commercial or financial relationships that could be construed as a potential conflict of interest.
References
1. Mans BJ. Evolution of vertebrate hemostatic and inflammatory control mechanisms in blood-feeding arthropods. J Innate Immun (2011) 3:41–51. doi: 10.1159/000321599
2. Lehane MJ. The Biology of Blood-Sucking in Insects. 2nd ed. Cambridge: Cambridge University Press (2005). doi: 10.1017/CBO9780511610493
3. Coast GM. Neuroendocrine control of ionic homeostasis in blood-sucking insects. J Exp Biol (2009) 212:378–86. doi: 10.1242/jeb.024109
4. Sterkel M, Oliveira JHM, Bottino-Rojas V, Paiva-Silva GO, Oliveira PL. The Dose Makes the Poison: Nutritional Overload Determines the Life Traits of Blood-Feeding Arthropods. Trends Parasitol (2017) 33:633–44. doi: 10.1016/j.pt.2017.04.008
5. Benoit JB, Denlinger DL. Bugs battle stress from hot blood. eLife (2017) 6:e33035. doi: 10.7554/eLife.33035
6. Philip CB, Burgdorfer W. Arthropod Vectors as Reservoirs of Microbial Disease Agents. Annu Rev Entomol (1961) 6:391–412. doi: 10.1146/annurev.en.06.010161.002135
7. Fritz GN. Biology and Ecology of Bat Flies (Diptera: Streblidae) on Bats in the Genus Carollia. J Med Entomol (1983) 20:1–10. doi: 10.1093/jmedent/20.1.1
8. Zhao YO, Kurscheid S, Zhang Y, Liu L, Zhang L, Loeliger K, et al. Enhanced Survival of Plasmodium-Infected Mosquitoes during Starvation. PloS One (2012) 7:e40556. doi: 10.1371/journal.pone.0040556
9. McCue MD, Boardman L, Clusella-Trullas S, Kleynhans E, Terblanche JS. The speed and metabolic cost of digesting a blood meal depends on temperature in a major disease vector. J Exp Biol (2016) 219:1893–902. doi: 10.1242/jeb.138669
10. Krasnov BR, Khokhlova IS, Fielden LJ, Burdelova NI. Time of survival under starvation in two flea species (Siphonaptera: Pulicidae) at different air temperatures and relative humidities. J Vector Ecol J Soc Vector Ecol (2002) 27:70–81.
11. Cabello DR. Resistance to starvation of Rhodnius neivai Lent, 1953 (Hemiptera: Reduviidae: Triatominae) under experimental conditions. Mem Inst Oswaldo Cruz (2001) 96:587–91. doi: 10.1590/S0074-02762001000400023
12. Polanco AM, Miller DM, Brewster CC. Survivorship During Starvation for Cimex lectularius L. Insects (2011) 2:232–42. doi: 10.3390/insects2020232
13. Oliver JH. Biology and Systematics of Ticks (Acari:Ixodida). Annu Rev Ecol Syst (1989) 20:397–430. doi: 10.1146/annurev.es.20.110189.002145
14. Benoit JB, Lopez-Martinez G, Patrick KR, Phillips ZP, Krause TB, Denlinger DL. Drinking a hot blood meal elicits a protective heat shock response in mosquitoes. Proc Natl Acad Sci (2011) 108:8026–9. doi: 10.1073/pnas.1105195108
15. Lahondère C, Lazzari CR. Mosquitoes Cool Down during Blood Feeding to Avoid Overheating. Curr Biol (2012) 22:40–5. doi: 10.1016/j.cub.2011.11.029
16. Lahondère C, Lazzari CR. Thermal effect of blood feeding in the telmophagous fly Glossina morsitans morsitans. J Therm Biol (2015) 48:45–50. doi: 10.1016/j.jtherbio.2014.12.009
17. Lane NJ, Harrison JB. An unusual cell surface modification: a double plasma membrane. J Cell Sci (1979) 39:355–72.
18. Silva CP, Silva JR, Vasconcelos FF, Petretski MDA, Damatta RA, Ribeiro AF, et al. Occurrence of midgut perimicrovillar membranes in paraneopteran insect orders with comments on their function and evolutionary significance. Arthropod Struct Dev (2004) 33:139–48. doi: 10.1016/j.asd.2003.12.002
19. Gutiérrez-Cabrera AE, Córdoba-Aguilar A, Zenteno E, Lowenberger C, Espinoza B. Origin, evolution and function of the hemipteran perimicrovillar membrane with emphasis on Reduviidae that transmit Chagas disease. Bull Entomol Res (2016) 106:279–91. doi: 10.1017/S0007485315000929
20. Oliveira MF, Silva JR, Dansa-Petretski M, de Souza W, Lins U, Braga CM, et al. Haem detoxification by an insect. Nature (1999) 400:517–8. doi: 10.1038/22910
21. Oliveira MF, Silva JR, Dansa-Petretski M, de Souza W, Braga CM, Masuda H, et al. Haemozoin formation in the midgut of the blood-sucking insect Rhodnius prolixus. FEBS Lett (2000) 477:95–8. doi: 10.1016/s0014-5793(00)01786-5
22. Oliveira MF, Kycia SW, Gomez A, Kosar AJ, Bohle DS, Hempelmann E, et al. Structural and morphological characterization of hemozoin produced by Schistosoma mansoni and Rhodnius prolixus. FEBS Lett (2005) 579:6010–6. doi: 10.1016/j.febslet.2005.09.035
23. Pagola S, Stephens PW, Bohle DS, Kosar AD, Madsen SK. The structure of malaria pigment beta-haematin. Nature (2000) 404:307–10. doi: 10.1038/35005132
24. Silva JR, Mury FB, Oliveira MF, Oliveira PL, Silva CP, Dansa-Petretski M. Perimicrovillar membranes promote hemozoin formation into Rhodnius prolixus midgut. Insect Biochem Mol Biol (2007) 37:523–31. doi: 10.1016/j.ibmb.2007.01.001
25. Braz GR, Coelho HS, Masuda H, Oliveira PL. A missing metabolic pathway in the cattle tick Boophilus microplus. Curr Biol CB (1999) 9:703–6. doi: 10.1016/s0960-9822(99)80312-1
26. Lara FA, Lins U, Paiva-Silva G, Almeida IC, Braga CM, Miguens FC, et al. A new intracellular pathway of haem detoxification in the midgut of the cattle tick Boophilus microplus: aggregation inside a specialized organelle, the hemosome. J Exp Biol (2003) 206:1707–15. doi: 10.1242/jeb.00334
27. Narasimhan S, Rajeevan N, Liu L, Zhao YO, Heisig J, Pan J, et al. Gut microbiota of the tick vector Ixodes scapularis modulate colonization of the lyme disease spirochete. Cell Host Microbe (2014) 15:58–71. doi: 10.1016/j.chom.2013.12.001
28. Rose C, Belmonte R, Armstrong SD, Molyneux G, Haines LR, Lehane MJ, et al. An investigation into the protein composition of the teneral Glossina morsitans morsitans peritrophic matrix. PloS Negl Trop Dis (2014) 8:e2691. doi: 10.1371/journal.pntd.0002691
29. Graça-Souza AV, Maya-Monteiro C, Paiva-Silva GO, Braz GRC, Paes MC, Sorgine MHF, et al. Adaptations against heme toxicity in blood-feeding arthropods. Insect Biochem Mol Biol (2006) 36:322–35. doi: 10.1016/j.ibmb.2006.01.009
30. Solyman M, Brayton KA, Shaw DK, Omsland A, McGeehan S, Scoles GA, et al. Predicted iron metabolism genes in hard ticks and their response to iron reduction in Dermacentor andersoni cells. Ticks Tick-Borne Dis (2021) 12:101584. doi: 10.1016/j.ttbdis.2020.101584
31. Beyenbach KW, Piermarini PM. Transcellular and paracellular pathways of transepithelial fluid secretion in Malpighian (renal) tubules of the yellow fever mosquito Aedes aegypti. Acta Physiol (2011) 202:387–407. doi: 10.1111/j.1748-1716.2010.02195.x
32. Grootjans J, Kaser A, Kaufman RJ, Blumberg RS. The unfolded protein response in immunity and inflammation. Nat Rev Immunol (2016) 16:469–84. doi: 10.1038/nri.2016.62
33. Hetz C. The unfolded protein response: controlling cell fate decisions under ER stress and beyond. Nat Rev Mol Cell Biol (2012) 13:89–102. doi: 10.1038/nrm3270
34. Schröder M, Kaufman RJ. The mammalian unfolded protein response. Annu Rev Biochem (2005) 74:739–89. doi: 10.1146/annurev.biochem.73.011303.074134
35. Kedersha N, Ivanov P, Anderson P. Stress granules and cell signaling: more than just a passing phase? Trends Biochem Sci (2013) 38:494–506. doi: 10.1016/j.tibs.2013.07.004
36. Protter DSW, Parker R. Principles and Properties of Stress Granules. Trends Cell Biol (2016) 26:668–79. doi: 10.1016/j.tcb.2016.05.004
37. Mahboubi H, Stochaj U. Cytoplasmic stress granules: Dynamic modulators of cell signaling and disease. Biochim Biophys Acta BBA Mol Basis Dis (2017) 1863:884–95. doi: 10.1016/j.bbadis.2016.12.022
38. Zhou Y, Fang L, Wang D, Cai K, Chen H, Xiao S. Porcine Reproductive and Respiratory Syndrome Virus Infection Induces Stress Granule Formation Depending on Protein Kinase R-like Endoplasmic Reticulum Kinase (PERK) in MARC-145 Cells. Front Cell Infect Microbiol (2017) 7:111. doi: 10.3389/fcimb.2017.00111
39. Kültz D. Evolution of the cellular stress proteome: from monophyletic origin to ubiquitous function. J Exp Biol (2003) 206:3119–24. doi: 10.1242/jeb.00549
40. Kültz D. Molecular and evolutionary basis of the cellular stress response. Annu Rev Physiol (2004) 67:225–57. doi: 10.1146/annurev.physiol.67.040403.103635
41. Dostálová A, Volf P. Leishmania development in sand flies: parasite-vector interactions overview. Parasit Vectors (2012) 5:276–6. doi: 10.1186/1756-3305-5-276
42. Maier WA, Becker-Feldman H, Seitz HM. Pathology of malaria-infected mosquitoes. Parasitol Today Pers Ed (1987) 3:216–8. doi: 10.1016/0169-4758(87)90063-9
43. Bacot AW. LXXXI. Further notes on the mechanism of the transmission of plague by fleas. J Hyg (Lond) (1915) 14:774–6.
44. Bacot AW, Martin CJ. LXVII. Observations on the mechanism of the transmission of plague by fleas. J Hyg (Lond) (1914) 13:423–39.
45. Shaw DK, Tate AT, Schneider DS, Levashina EA, Kagan JC, Pal U, et al. Vector Immunity and Evolutionary Ecology: The Harmonious Dissonance. Trends Immunol (2018) 39:862–73. doi: 10.1016/j.it.2018.09.003
46. Casadevall A, Pirofski L. Host-Pathogen Interactions: The Attributes of Virulence. J Infect Dis (2001) 184:337–44. doi: 10.1086/322044
47. Hillyer JF, Schmidt SL, Christensen BM. Hemocyte-mediated phagocytosis and melanization in the mosquito Armigeres subalbatus following immune challenge by bacteria. Cell Tissue Res (2003) 313:117–27. doi: 10.1007/s00441-003-0744-y
48. Garver LS, Dong Y, Dimopoulos G. Caspar Controls Resistance to Plasmodium falciparum in Diverse Anopheline Species. PloS Pathog (2009) 5:e1000335. doi: 10.1371/journal.ppat.1000335
49. Blumberg BJ, Trop S, Das S, Dimopoulos G. Bacteria- and IMD pathway-independent immune defenses against Plasmodium falciparum in Anopheles gambiae. PloS One (2013) 8:e72130. doi: 10.1371/journal.pone.0072130
50. Oliva Chávez AS, Shaw DK, Munderloh UG, Pedra JHF. Tick Humoral Responses: Marching to the Beat of a Different Drummer. Front Microbiol (2017) 8:223. doi: 10.3389/fmicb.2017.00223
51. de la Fuente J, Antunes S, Bonnet S, Cabezas-Cruz A, Domingos AG, Estrada-Peña A, et al. Tick-Pathogen Interactions and Vector Competence: Identification of Molecular Drivers for Tick-Borne Diseases. Front Cell Infect Microbiol (2017) 7:114. doi: 10.3389/fcimb.2017.00114
52. Lane RS. “Competence of ticks as vectors of microbial agents with an emphasis on Borrelia burgdorferi. In: Sonenshine DE, Mather TN, editors. Ecological dynamics of tick-borne zoonoses. New York, NY: Oxford University Press (1994). p. 45–67.
53. Goddard J. Infectious Diseases and Arthropods. Totowa, NJ: Humana Press (2000). Available at: http://www.springer.com/us/book/9781603273992 (Accessed September 28, 2017).
54. Byndloss MX, Keestra-Gounder AM, Bäumler AJ, Tsolis RM. NOD1 and NOD2: New Functions Linking Endoplasmic Reticulum Stress and Inflammation. DNA Cell Biol (2016) 35:311–3. doi: 10.1089/dna.2016.3396
55. Keestra-Gounder AM, Byndloss MX, Seyffert N, Young BM, Chávez-Arroyo A, Tsai AY, et al. NOD1 and NOD2 signalling links ER stress with inflammation. Nature (2016) 532:394–7. doi: 10.1038/nature17631
56. Hu P, Han Z, Couvillon AD, Kaufman RJ, Exton JH. Autocrine tumor necrosis factor alpha links endoplasmic reticulum stress to the membrane death receptor pathway through IRE1alpha-mediated NF-kappaB activation and down-regulation of TRAF2 expression. Mol Cell Biol (2006) 26:3071–84. doi: 10.1128/MCB.26.8.3071-3084.2006
57. Yamazaki H, Hiramatsu N, Hayakawa K, Tagawa Y, Okamura M, Ogata R, et al. Activation of the Akt-NF-kappaB pathway by subtilase cytotoxin through the ATF6 branch of the unfolded protein response. J Immunol Baltim Md 1950 (2009) 183:1480–7. doi: 10.4049/jimmunol.0900017
58. Nakajima S, Kitamura M. Bidirectional regulation of NF-κB by reactive oxygen species: A role of unfolded protein response. Free Radic Biol Med (2013) 65:162–74. doi: 10.1016/j.freeradbiomed.2013.06.020
59. Moretti J, Blander JM. Cell-autonomous stress responses in innate immunity. J Leukoc Biol (2017) 101:77–86. doi: 10.1189/jlb.2MR0416-201R
60. Janssens S, Pulendran B, Lambrecht BN. Emerging functions of the unfolded protein response in immunity. Nat Immunol (2014) 15:910–9. doi: 10.1038/ni.2991
61. Valanne S, Wang J-H, Rämet M. The Drosophila Toll Signaling Pathway. J Immunol (2011) 186:649–56. doi: 10.4049/jimmunol.1002302
62. Engström Y. Induction and regulation of antimicrobial peptides in Drosophila. Dev Comp Immunol (1999) 23:345–58. doi: 10.1016/S0145-305X(99)00016-6
63. Dushay MS, Asling B, Hultmark D. Origins of immunity: Relish, a compound Rel-like gene in the antibacterial defense of Drosophila. Proc Natl Acad Sci (1996) 93:10343–7. doi: 10.1073/pnas.93.19.10343
64. Meng X, Khanuja BS, Ip YT. Toll receptor-mediated Drosophila immune response requires Dif, an NF-κB factor. Genes Dev (1999) 13:792–7.
65. Kleino A, Silverman N. The Drosophila IMD pathway in the activation of the humoral immune response. Dev Comp Immunol (2014) 42:25–35. doi: 10.1016/j.dci.2013.05.014
66. Kaneko T, Yano T, Aggarwal K, Lim J-H, Ueda K, Oshima Y, et al. PGRP-LC and PGRP-LE have essential yet distinct functions in the drosophila immune response to monomeric DAP-type peptidoglycan. Nat Immunol (2006) 7:715–23. doi: 10.1038/ni1356
67. Lu Y, Su F, Li Q, Zhang J, Li Y, Tang T, et al. Pattern recognition receptors in Drosophila immune responses. Dev Comp Immunol (2020) 102:103468. doi: 10.1016/j.dci.2019.103468
68. Hedengren-Olcott M, Olcott MC, Mooney DT, Ekengren S, Geller BL, Taylor BJ. Differential Activation of the NF-κB-like Factors Relish and Dif in Drosophila melanogaster by Fungi and Gram-positive Bacteria. J Biol Chem (2004) 279:21121–7. doi: 10.1074/jbc.M313856200
69. Christophides GK, Zdobnov E, Barillas-Mury C, Birney E, Blandin S, Blass C, et al. Immunity-Related Genes and Gene Families in Anopheles gambiae. Science (2002) 298:159–65. doi: 10.1126/science.1077136
70. Palmer WJ, Jiggins FM. Comparative Genomics Reveals the Origins and Diversity of Arthropod Immune Systems. Mol Biol Evol (2015) 32:2111–29. doi: 10.1093/molbev/msv093
71. Gulia-Nuss M, Nuss AB, Meyer JM, Sonenshine DE, Roe RM, Waterhouse RM, et al. Genomic insights into the Ixodes scapularis tick vector of Lyme disease. Nat Commun (2016) 7:10507. doi: 10.1038/ncomms10507
72. Rawlings JS, Rosler KM, Harrison DA. The JAK/STAT signaling pathway. J Cell Sci (2004) 117:1281–3. doi: 10.1242/jcs.00963
73. Darnell JE. STATs and Gene Regulation. Science (1997) 277:1630–5. doi: 10.1126/science.277.5332.1630
74. Harrison DA, McCoon PE, Binari R, Gilman M, Perrimon N. Drosophila unpaired encodes a secreted protein that activates the JAK signaling pathway. Genes Dev (1998) 12:3252–63.
75. Buchon N, Silverman N, Cherry S. Immunity in Drosophila melanogaster–from microbial recognition to whole-organism physiology. Nat Rev Immunol (2014) 14:796–810. doi: 10.1038/nri3763
76. Lemaitre B, Hoffmann J. The Host Defense of Drosophila melanogaster. Annu Rev Immunol (2007) 25:697–743. doi: 10.1146/annurev.immunol.25.022106.141615
77. Tsunoda S, Avezov E, Zyryanova A, Konno T, Mendes-Silva L, Pinho Melo E, et al. Intact protein folding in the glutathione-depleted endoplasmic reticulum implicates alternative protein thiol reductants. eLife (2014) 3:e03421. doi: 10.7554/eLife.03421
78. Malhotra JD, Miao H, Zhang K, Wolfson A, Pennathur S, Pipe SW, et al. Antioxidants reduce endoplasmic reticulum stress and improve protein secretion. Proc Natl Acad Sci USA (2008) 105:18525–30. doi: 10.1073/pnas.0809677105
79. Lerner AG, Upton J-P, Praveen PVK, Ghosh R, Nakagawa Y, Igbaria A, et al. IRE1α induces thioredoxin-interacting protein to activate the NLRP3 inflammasome and promote programmed cell death under irremediable ER stress. Cell Metab (2012) 16:250–64. doi: 10.1016/j.cmet.2012.07.007
80. Oslowski CM, Hara T, O’Sullivan-Murphy B, Kanekura K, Lu S, Hara M, et al. Thioredoxin-interacting protein mediates ER stress-induced β cell death through initiation of the inflammasome. Cell Metab (2012) 16:265–73. doi: 10.1016/j.cmet.2012.07.005
81. Celli J, Tsolis RM. Bacteria, the ER and the Unfolded Protein Response: Friends or Foes? Nat Rev Microbiol (2015) 13:71–82. doi: 10.1038/nrmicro3393
82. Fu S, Yang L, Li P, Hofmann O, Dicker L, Hide W, et al. Aberrant lipid metabolism disrupts calcium homeostasis causing liver endoplasmic reticulum stress in obesity. Nature (2011) 473:528–31. doi: 10.1038/nature09968
83. Schröder M, Clark R, Liu CY, Kaufman RJ. The unfolded protein response represses differentiation through the RPD3-SIN3 histone deacetylase. EMBO J (2004) 23:2281–92. doi: 10.1038/sj.emboj.7600233
84. Schröder M, Chang JS, Kaufman RJ. The unfolded protein response represses nitrogen-starvation induced developmental differentiation in yeast. Genes Dev (2000) 14:2962–75. doi: 10.1101/gad.852300
85. Bertolotti A, Zhang Y, Hendershot LM, Harding HP, Ron D. Dynamic interaction of BiP and ER stress transducers in the unfolded-protein response. Nat Cell Biol (2000) 2:326–32. doi: 10.1038/35014014
86. Adams CJ, Kopp MC, Larburu N, Nowak PR, Ali MMU. Structure and Molecular Mechanism of ER Stress Signaling by the Unfolded Protein Response Signal Activator IRE1. Front Mol Biosci (2019) 6:11. doi: 10.3389/fmolb.2019.00011
87. Pincus D, Chevalier MW, Aragón T, van Anken E, Vidal SE, El-Samad H, et al. BiP binding to the ER-stress sensor Ire1 tunes the homeostatic behavior of the unfolded protein response. PloS Biol (2010) 8:e1000415. doi: 10.1371/journal.pbio.1000415
88. Shen J, Chen X, Hendershot L, Prywes R. ER stress regulation of ATF6 localization by dissociation of BiP/GRP78 binding and unmasking of Golgi localization signals. Dev Cell (2002) 3:99–111. doi: 10.1016/s1534-5807(02)00203-4
89. Nadanaka S, Okada T, Yoshida H, Mori K. Role of disulfide bridges formed in the luminal domain of ATF6 in sensing endoplasmic reticulum stress. Mol Cell Biol (2007) 27:1027–43. doi: 10.1128/MCB.00408-06
90. Medigeshi GR, Lancaster AM, Hirsch AJ, Briese T, Lipkin WI, DeFilippis V, et al. West Nile Virus Infection Activates the Unfolded Protein Response, Leading to CHOP Induction and Apoptosis. J Virol (2007) 81:10849–60. doi: 10.1128/JVI.01151-07
91. Harding HP, Zhang Y, Ron D. Protein translation and folding are coupled by an endoplasmic-reticulum-resident kinase. Nature (1999) 397:271–4. doi: 10.1038/16729
92. Connor JH, Weiser DC, Li S, Hallenbeck JM, Shenolikar S. Growth Arrest and DNA Damage-Inducible Protein GADD34 Assembles a Novel Signaling Complex Containing Protein Phosphatase 1 and Inhibitor 1. Mol Cell Biol (2001) 21:6841–50. doi: 10.1128/MCB.21.20.6841-6850.2001
93. Harding HP, Zhang Y, Zeng H, Novoa I, Lu PD, Calfon M, et al. An Integrated Stress Response Regulates Amino Acid Metabolism and Resistance to Oxidative Stress. Mol Cell (2003) 11:619–33. doi: 10.1016/S1097-2765(03)00105-9
94. Ye J, Rawson RB, Komuro R, Chen X, Davé UP, Prywes R, et al. ER Stress Induces Cleavage of Membrane-Bound ATF6 by the Same Proteases that Process SREBPs. Mol Cell (2000) 6:1355–64. doi: 10.1016/S1097-2765(00)00133-7
95. Schindler AJ, Schekman R. In vitro reconstitution of ER-stress induced ATF6 transport in COPII vesicles. Proc Natl Acad Sci (2009) 106:17775–80. doi: 10.1073/pnas.0910342106
96. Wu H, Ng BSH, Thibault G. Endoplasmic reticulum stress response in yeast and humans. Biosci Rep (2014) 34:321–30. doi: 10.1042/BSR20140058
97. Adachi Y, Yamamoto K, Okada T, Yoshida H, Harada A, Mori K. ATF6 Is a Transcription Factor Specializing in the Regulation of Quality Control Proteins in the Endoplasmic Reticulum. Cell Struct Funct (2008) 33:75–89. doi: 10.1247/csf.07044
98. Oslowski CM, Urano F. Measuring ER stress and the unfolded protein response using mammalian tissue culture system. Methods Enzymol (2011) 490:71–92. doi: 10.1016/B978-0-12-385114-7.00004-0
99. Yoshida H, Matsui T, Yamamoto A, Okada T, Mori K. XBP1 mRNA Is Induced by ATF6 and Spliced by IRE1 in Response to ER Stress to Produce a Highly Active Transcription Factor. Cell (2001) 107:881–91. doi: 10.1016/S0092-8674(01)00611-0
100. Calfon M, Zeng H, Urano F, Till JH, Hubbard SR, Harding HP, et al. IRE1 couples endoplasmic reticulum load to secretory capacity by processing the XBP-1 mRNA. Nature (2002) 415:92–6. doi: 10.1038/415092a
101. Shoulders MD, Ryno LM, Genereux JC, Moresco JJ, Tu PG, Wu C, et al. Stress-Independent Activation of XBP1s and/or ATF6 Reveals Three Functionally Diverse ER Proteostasis Environments. Cell Rep (2013) 3:1279–92. doi: 10.1016/j.celrep.2013.03.024
102. Hollien J, Lin JH, Li H, Stevens N, Walter P, Weissman JS. Regulated Ire1-dependent decay of messenger RNAs in mammalian cells. J Cell Biol (2009) 186:323–31. doi: 10.1083/jcb.200903014
103. Hu P, Han Z, Couvillon AD, Kaufman RJ, Exton JH. Autocrine Tumor Necrosis Factor Alpha Links Endoplasmic Reticulum Stress to the Membrane Death Receptor Pathway through IRE1α-Mediated NF-κB Activation and Down-Regulation of TRAF2 Expression. Mol Cell Biol (2006) 26:3071–84. doi: 10.1128/MCB.26.8.3071-3084.2006
104. Zhu X, Zhang J, Sun H, Jiang C, Dong Y, Shan Q, et al. Ubiquitination of Inositol-requiring Enzyme 1 (IRE1) by the E3 Ligase CHIP Mediates the IRE1/TRAF2/JNK Pathway. J Biol Chem (2014) 289:30567–77. doi: 10.1074/jbc.M114.562868
105. Urano F, Wang X, Bertolotti A, Zhang Y, Chung P, Harding HP, et al. Coupling of Stress in the ER to Activation of JNK Protein Kinases by Transmembrane Protein Kinase IRE1. Science (2000) 287:664–6. doi: 10.1126/science.287.5453.664
106. Pakos-Zebrucka K, Koryga I, Mnich K, Ljujic M, Samali A, Gorman AM. The integrated stress response. EMBO Rep (2016) 17:1374–95. doi: 10.15252/embr.201642195
107. Ye J, Kumanova M, Hart LS, Sloane K, Zhang H, De Panis DN, et al. The GCN2-ATF4 pathway is critical for tumour cell survival and proliferation in response to nutrient deprivation. EMBO J (2010) 29:2082–96. doi: 10.1038/emboj.2010.81
108. Natarajan K, Meyer MR, Jackson BM, Slade D, Roberts C, Hinnebusch AG, et al. Transcriptional Profiling Shows that Gcn4p Is a Master Regulator of Gene Expression during Amino Acid Starvation in Yeast. Mol Cell Biol (2001) 21:4347–68. doi: 10.1128/MCB.21.13.4347-4368.2001
109. Webster SJ, Ellis L, O’Brien LM, Tyrrell B, Fitzmaurice TJ, Elder MJ, et al. IRE1α mediates PKR activation in response to Chlamydia trachomatis infection. Microbes Infect (2016) 18:472–83. doi: 10.1016/j.micinf.2016.03.010
110. Donnelly N, Gorman AM, Gupta S, Samali A. The eIF2α kinases: their structures and functions. Cell Mol Life Sci (2013) 70:3493–511. doi: 10.1007/s00018-012-1252-6
111. Taniuchi S, Miyake M, Tsugawa K, Oyadomari M, Oyadomari S. Integrated stress response of vertebrates is regulated by four eIF2α kinases. Sci Rep (2016) 6:32886. doi: 10.1038/srep32886
112. Deng J, Harding HP, Raught B, Gingras A-C, Berlanga JJ, Scheuner D, et al. Activation of GCN2 in UV-Irradiated Cells Inhibits Translation. Curr Biol (2002) 12:1279–86. doi: 10.1016/S0960-9822(02)01037-0
113. Harding HP, Zhang Y, Bertolotti A, Zeng H, Ron D. Perk Is Essential for Translational Regulation and Cell Survival during the Unfolded Protein Response. Mol Cell (2000) 5:897–904. doi: 10.1016/S1097-2765(00)80330-5
114. Szegezdi E, Logue SE, Gorman AM, Samali A. Mediators of endoplasmic reticulum stress-induced apoptosis. EMBO Rep (2006) 7:880–5. doi: 10.1038/sj.embor.7400779
115. Lemaire PA, Anderson E, Lary J, Cole JL. Mechanism of PKR Activation by dsRNA. J Mol Biol (2008) 381:351–60. doi: 10.1016/j.jmb.2008.05.056
116. Chukwurah E, Patel RC. Stress-induced TRBP phosphorylation enhances its interaction with PKR to regulate cellular survival. Sci Rep (2018) 8:1020. doi: 10.1038/s41598-018-19360-8
117. Reineke LC, Lloyd RE. The Stress Granule Protein G3BP1 Recruits Protein Kinase R To Promote Multiple Innate Immune Antiviral Responses. J Virol (2015) 89:2575–89. doi: 10.1128/JVI.02791-14
118. Han A-P, Yu C, Lu L, Fujiwara Y, Browne C, Chin G, et al. Heme-regulated eIF2α kinase (HRI) is required for translational regulation and survival of erythroid precursors in iron deficiency. EMBO J (2001) 20:6909–18. doi: 10.1093/emboj/20.23.6909
119. Aitken CE, Lorsch JR. A mechanistic overview of translation initiation in eukaryotes. Nat Struct Mol Biol (2012) 19:568–76. doi: 10.1038/nsmb.2303
120. Sonenberg N, Hinnebusch AG. Regulation of Translation Initiation in Eukaryotes: Mechanisms and Biological Targets. Cell (2009) 136:731–45. doi: 10.1016/j.cell.2009.01.042
121. Krishnamoorthy T, Pavitt GD, Zhang F, Dever TE, Hinnebusch AG. Tight Binding of the Phosphorylated α Subunit of Initiation Factor 2 (eIF2α) to the Regulatory Subunits of Guanine Nucleotide Exchange Factor eIF2B Is Required for Inhibition of Translation Initiation. Mol Cell Biol (2001) 21:5018–30. doi: 10.1128/MCB.21.15.5018-5030.2001
122. Pavitt GD, Ramaiah KVA, Kimball SR, Hinnebusch AG. eIF2 independently binds two distinct eIF2B subcomplexes that catalyze and regulate guanine–nucleotide exchange. Genes Dev (1998) 12:514–26.
123. Adomavicius T, Guaita M, Zhou Y, Jennings MD, Latif Z, Roseman AM, et al. The structural basis of translational control by eIF2 phosphorylation. Nat Commun (2019) 10:2136. doi: 10.1038/s41467-019-10167-3
124. Ohoka N, Yoshii S, Hattori T, Onozaki K, Hayashi H. TRB3, a novel ER stress-inducible gene, is induced via ATF4–CHOP pathway and is involved in cell death. EMBO J (2005) 24:1243–55. doi: 10.1038/sj.emboj.7600596
125. Han J, Back SH, Hur J, Lin Y-H, Gildersleeve R, Shan J, et al. ER-stress-induced transcriptional regulation increases protein synthesis leading to cell death. Nat Cell Biol (2013) 15:481–90. doi: 10.1038/ncb2738
126. Ma Y, Hendershot LM. Delineation of a Negative Feedback Regulatory Loop That Controls Protein Translation during Endoplasmic Reticulum Stress. J Biol Chem (2003) 278:34864–73. doi: 10.1074/jbc.M301107200
127. Kim WJ, Back SH, Kim V, Ryu I, Jang SK. Sequestration of TRAF2 into Stress Granules Interrupts Tumor Necrosis Factor Signaling under Stress Conditions. Mol Cell Biol (2005) 25:2450–62. doi: 10.1128/MCB.25.6.2450-2462.2005
128. Arimoto K, Fukuda H, Imajoh-Ohmi S, Saito H, Takekawa M. Formation of stress granules inhibits apoptosis by suppressing stress-responsive MAPK pathways. Nat Cell Biol (2008) 10:1324–32. doi: 10.1038/ncb1791
129. Hoang H-D, Graber TE, Alain T. Battling for Ribosomes: Translational Control at the Forefront of the Antiviral Response. J Mol Biol (2018) 430:1965–92. doi: 10.1016/j.jmb.2018.04.040
130. Won S, Eidenschenk C, Arnold CN, Siggs OM, Sun L, Brandl K, et al. Increased Susceptibility to DNA Virus Infection in Mice with a GCN2 Mutation. J Virol (2012) 86:1802–8. doi: 10.1128/JVI.05660-11
131. Berlanga JJ, Ventoso I, Harding HP, Deng J, Ron D, Sonenberg N, et al. Antiviral effect of the mammalian translation initiation factor 2α kinase GCN2 against RNA viruses. EMBO J (2006) 25:1730–40. doi: 10.1038/sj.emboj.7601073
132. Amen OM, Sarker SD, Ghildyal R, Arya A. Endoplasmic Reticulum Stress Activates Unfolded Protein Response Signaling and Mediates Inflammation, Obesity, and Cardiac Dysfunction: Therapeutic and Molecular Approach. Front Pharmacol (2019) 10:977. doi: 10.3389/fphar.2019.00977
133. Meyerovich K, Ortis F, Allagnat F, Cardozo AK. Endoplasmic reticulum stress and the unfolded protein response in pancreatic islet inflammation. J Mol Endocrinol (2016) 57:R1–R17. doi: 10.1530/jme-15-0306
134. Ghosh R, Colon-Negron K, Papa FR. Endoplasmic reticulum stress, degeneration of pancreatic islet β-cells, and therapeutic modulation of the unfolded protein response in diabetes. Mol Metab (2019) 27:S60–8. doi: 10.1016/j.molmet.2019.06.012
135. Coope A, Pascoal LB, Botezelli JD, da Silva FAR, de LS Ayrizono M, Rodrigues BL, et al. ER stress activation in the intestinal mucosa but not in mesenteric adipose tissue is associated with inflammation in Crohn’s disease patients. PloS One (2019) 14:e0223105. doi: 10.1371/journal.pone.0223105
136. Fougeray S, Bouvier N, Beaune P, Legendre C, Anglicheau D, Thervet E, et al. Metabolic stress promotes renal tubular inflammation by triggering the unfolded protein response. Cell Death Dis (2011) 2:e143–3. doi: 10.1038/cddis.2011.26
137. Yang W, Sheng F, Sun B, Fischbach S, Xiao X. The role of ORMDL3/ATF6 in compensated beta cell proliferation during early diabetes. Aging (2019) 11:2787–96. doi: 10.18632/aging.101949
138. Abuaita BH, Burkholder KM, Boles BR, O’Riordan MX. The Endoplasmic Reticulum Stress Sensor Inositol-Requiring Enzyme 1α Augments Bacterial Killing through Sustained Oxidant Production. mBio (2015) 6. doi: 10.1128/mBio.00705-15
139. Tentaku A, Shimohata T, Hatayama S, Kido J, Nguyen AQ, Kanda Y, et al. Host cellular unfolded protein response signaling regulates Campylobacter jejuni invasion. PloS One (2018) 13:e0205865. doi: 10.1371/journal.pone.0205865
140. Treacy-Abarca S, Mukherjee S. Legionella suppresses the host unfolded protein response via multiple mechanisms. Nat Commun (2015) 6. doi: 10.1038/ncomms8887
141. Hempstead AD, Isberg RR. Inhibition of host cell translation elongation by Legionella pneumophila blocks the host cell unfolded protein response. Proc Natl Acad Sci (2015) 112:E6790–7. doi: 10.1073/pnas.1508716112
142. Smith JA, Khan M, Magnani DD, Harms JS, Durward M, Radhakrishnan GK, et al. Brucella Induces an Unfolded Protein Response via TcpB That Supports Intracellular Replication in Macrophages. PloS Pathog (2013) 9:e1003785. doi: 10.1371/journal.ppat.1003785
143. Myeni S, Child R, Ng TW, Iii JJK, Wehrly TD, Porcella SF, et al. Brucella Modulates Secretory Trafficking via Multiple Type IV Secretion Effector Proteins. PloS Pathog (2013) 9:e1003556. doi: 10.1371/journal.ppat.1003556
144. de Jong MF, Starr T, Winter MG, den Hartigh AB, Child R, Knodler LA, et al. Sensing of Bacterial Type IV Secretion via the Unfolded Protein Response. mBio (2013) 4. doi: 10.1128/mBio.00418-12
145. Zhou D, Zhi F-J, Qi M-Z, Bai F-R, Zhang G, Li J-M, et al. Brucella induces unfolded protein response and inflammatory response via GntR in alveolar macrophages. Oncotarget (2017) 9:5184–96. doi: 10.18632/oncotarget.23706
146. Taguchi Y, Imaoka K, Kataoka M, Uda A, Nakatsu D, Horii-Okazaki S, et al. Yip1A, a Novel Host Factor for the Activation of the IRE1 Pathway of the Unfolded Protein Response during Brucella Infection. PloS Pathog (2015) 11:e1004747. doi: 10.1371/journal.ppat.1004747
147. Keestra-Gounder AM, Byndloss MX, Seyffert N, Young BM, Chávez-Arroyo A, Tsai AY, et al. NOD1/NOD2 signaling links ER stress with inflammation. Nature (2016) 532:394–7. doi: 10.1038/nature17631
148. Zellner B, Huntley JF. Ticks and Tularemia: Do We Know What We Don’t Know? Front Cell Infect Microbiol (2019) 9:146. doi: 10.3389/fcimb.2019.00146
149. Petersen JM, Schriefer ME. Tularemia: emergence/re-emergence. Vet Res (2005) 36:455–67. doi: 10.1051/vetres:2005006
150. Calhoun EL. Natural Occurrence of Tularemia in the Lone Star Tick, Amblyomma Americanum (Linn.), and in Dogs in Arkansas. Am J Trop Med Hyg (1954) 3:360–6. doi: 10.4269/ajtmh.1954.3.360
151. Goethert HK, Shani I, Telford SR. Genotypic Diversity of Francisella tularensis Infecting Dermacentor variabilis Ticks on Martha’s Vineyard, Massachusetts. J Clin Microbiol (2004) 42:4968–73. doi: 10.1128/JCM.42.11.4968-4973.2004
152. Barel M, Harduin-Lepers A, Portier L, Slomianny M-C, Charbit A. Host glycosylation pathways and the unfolded protein response contribute to the infection by Francisella. Cell Microbiol (2016) 18:1763–81. doi: 10.1111/cmi.12614
153. Martinon F, Chen X, Lee A-H, Glimcher LH. Toll-like receptor activation of XBP1 regulates innate immune responses in macrophages. Nat Immunol (2010) 11:411–8. doi: 10.1038/ni.1857
154. Rodino KG, VieBrock L, Evans SM, Ge H, Richards AL, Carlyon JA. Orientia tsutsugamushi Modulates Endoplasmic Reticulum-Associated Degradation To Benefit Its Growth. Infect Immun (2018) 86. doi: 10.1128/IAI.00596-17
155. Yoshikawa Y, Sugimoto K, Ochiai Y, Ohashi N. Intracellular proliferation of Anaplasma phagocytophilum is promoted via modulation of endoplasmic reticulum stress signaling in host cells. Microbiol Immunol (2020) 64:270–9. doi: 10.1111/1348-0421.12770
156. Isler JA, Skalet AH, Alwine JC. Human Cytomegalovirus Infection Activates and Regulates the Unfolded Protein Response. J Virol (2005) 79:6890–9. doi: 10.1128/JVI.79.11.6890-6899.2005
157. Stahl S, Burkhart JM, Hinte F, Tirosh B, Mohr H, Zahedi RP, et al. Cytomegalovirus Downregulates IRE1 to Repress the Unfolded Protein Response. PloS Pathog (2013) 9:e1003544. doi: 10.1371/journal.ppat.1003544
158. Johnston BP, McCormick C. Herpesviruses and the Unfolded Protein Response. Viruses (2020) 12:17. doi: 10.3390/v12010017
159. Lv S, Sun E-C, Xu Q-Y, Zhang J-K, Wu D-L. Endoplasmic reticulum stress-mediated autophagy contributes to bluetongue virus infection via the PERK-eIF2α pathway. Biochem Biophys Res Commun (2015) 466:406–12. doi: 10.1016/j.bbrc.2015.09.039
160. Rathore APS, Ng M-L, Vasudevan SG. Differential unfolded protein response during Chikungunya and Sindbis virus infection: CHIKV nsP4 suppresses eIF2α phosphorylation. Virol J (2013) 10:36. doi: 10.1186/1743-422X-10-36
161. Li S, Kong L, Yu X. The expanding roles of endoplasmic reticulum stress in virus replication and pathogenesis. Crit Rev Microbiol (2015) 41:150–64. doi: 10.3109/1040841X.2013.813899
162. Lee Y-R, Kuo S-H, Lin C-Y, Fu P-J, Lin Y-S, Yeh T-M, et al. Dengue virus-induced ER stress is required for autophagy activation, viral replication, and pathogenesis both in vitro and in vivo. Sci Rep (2018) 8:489. doi: 10.1038/s41598-017-18909-3
163. Ambrose RL, Mackenzie JM. ATF6 Signaling Is Required for Efficient West Nile Virus Replication by Promoting Cell Survival and Inhibition of Innate Immune Responses. J Virol (2013) 87:2206–14. doi: 10.1128/JVI.02097-12
164. Ambrose RL, Mackenzie JM. West Nile Virus Differentially Modulates the Unfolded Protein Response To Facilitate Replication and Immune Evasion. J Virol (2011) 85:2723–32. doi: 10.1128/JVI.02050-10
165. Yu C, Achazi K, Niedrig M. Tick-borne encephalitis virus triggers inositol-requiring enzyme 1 (IRE1) and transcription factor 6 (ATF6) pathways of unfolded protein response. Virus Res (2013) 178:471–7. doi: 10.1016/j.virusres.2013.10.012
166. Lewy TG, Offerdahl DK, Grabowski JM, Kellman E, Mlera L, Chiramel A, et al. PERK-Mediated Unfolded Protein Response Signaling Restricts Replication of the Tick-Borne Flavivirus Langat Virus. Viruses (2020) 12:328. doi: 10.3390/v12030328
167. Kumar D, Embers M, Mather TN, Karim S. Is selenoprotein K required for Borrelia burgdorferi infection within the tick vector Ixodes scapularis? Parasit Vectors (2019) 12:289. doi: 10.1186/s13071-019-3548-y
168. Ghosh S, Jassar O, Kontsedalov S, Lebedev G, Wang C, Turner D, et al. Ghanim M. A Transcriptomics Approach Reveals Putative Interaction of Candidatus Liberibacter Solanacearum with the Endoplasmic Reticulum of Its Psyllid Vector. Insects (2019) 10:279. doi: 10.3390/insects10090279
169. Valderrama C, Clark A, Urano F, Unanue ER, Carrero JA. Listeria monocytogenes induces an interferon-enhanced activation of the integrated stress response that is detrimental for resolution of infection in mice. Eur J Immunol (2017) 47:830–40. doi: 10.1002/eji.201646856
170. Tattoli I, Sorbara MT, Vuckovic D, Ling A, Soares F, Carneiro LAM, et al. Amino Acid Starvation Induced by Invasive Bacterial Pathogens Triggers an Innate Host Defense Program. Cell Host Microbe (2012) 11:563–75. doi: 10.1016/j.chom.2012.04.012
171. Brann KR, Fullerton MS, Voth DE. Coxiella burnetii Requires Host Eukaryotic Initiation Factor 2α Activity for Efficient Intracellular Replication. Infect Immun (2020) 88. doi: 10.1128/IAI.00096-20
172. Rabouw HH, Visser LJ, Passchier TC, Langereis MA, Liu F, Giansanti P, et al. Inhibition of the integrated stress response by viral proteins that block p-eIF2–eIF2B association. Nat Microbiol (2020) 5:1361–73. doi: 10.1038/s41564-020-0759-0
173. McCormick C, Khaperskyy DA. Translation inhibition and stress granules in the antiviral immune response. Nat Rev Immunol (2017) 17:647–60. doi: 10.1038/nri.2017.63
174. Wuerth JD, Habjan M, Kainulainen M, Berisha B, Bertheloot D, Superti-Furga G, et al. eIF2B as a Target for Viral Evasion of PKR-Mediated Translation Inhibition. mBio (2020) 11. doi: 10.1128/mBio.00976-20
175. Ikegami T, Narayanan K, Won S, Kamitani W, Peters CJ, Makino S. Rift Valley Fever Virus NSs Protein Promotes Post-Transcriptional Downregulation of Protein Kinase PKR and Inhibits eIF2α Phosphorylation. PloS Pathog (2009) 5:e1000287. doi: 10.1371/journal.ppat.1000287
176. Kainulainen M, Habjan M, Hubel P, Busch L, Lau S, Colinge J, et al. Virulence Factor NSs of Rift Valley Fever Virus Recruits the F-Box Protein FBXO3 To Degrade Subunit p62 of General Transcription Factor TFIIH. J Virol (2014) 88:3464–73. doi: 10.1128/JVI.02914-13
177. Kainulainen M, Lau S, Samuel CE, Hornung V, Weber F. NSs Virulence Factor of Rift Valley Fever Virus Engages the F-Box Proteins FBXW11 and β-TRCP1 To Degrade the Antiviral Protein Kinase PKR. J Virol (2016) 90:6140–7. doi: 10.1128/JVI.00016-16
178. Courtney SC, Scherbik SV, Stockman BM, Brinton MA. West Nile Virus Infections Suppress Early Viral RNA Synthesis and Avoid Inducing the Cell Stress Granule Response. J Virol (2012) 86:3647–57. doi: 10.1128/JVI.06549-11
179. Hou S, Kumar A, Xu Z, Airo AM, Stryapunina I, Wong CP, et al. Zika Virus Hijacks Stress Granule Proteins and Modulates the Host Stress Response. J Virol (2017) 91. doi: 10.1128/JVI.00474-17
180. Bonenfant G, Williams N, Netzband R, Schwarz MC, Evans MJ, Pager CT. Zika Virus Subverts Stress Granules To Promote and Restrict Viral Gene Expression. J Virol (2019) 93. doi: 10.1128/JVI.00520-19
181. Emara MM, Brinton MA. Interaction of TIA-1/TIAR with West Nile and dengue virus products in infected cells interferes with stress granule formation and processing body assembly. Proc Natl Acad Sci (2007) 104:9041–6. doi: 10.1073/pnas.0703348104
182. Roth H, Magg V, Uch F, Mutz P, Klein P, Haneke K, et al. Flavivirus Infection Uncouples Translation Suppression from Cellular Stress Responses. mBio (2017) 8. doi: 10.1128/mBio.02150-16
183. Katoh H, Okamoto T, Fukuhara T, Kambara H, Morita E, Mori Y, et al. Japanese Encephalitis Virus Core Protein Inhibits Stress Granule Formation through an Interaction with Caprin-1 and Facilitates Viral Propagation. J Virol (2013) 87:489–502. doi: 10.1128/JVI.02186-12
184. Tu Y-C, Yu C-Y, Liang J-J, Lin E, Liao C-L, Lin Y-L. Blocking Double-Stranded RNA-Activated Protein Kinase PKR by Japanese Encephalitis Virus Nonstructural Protein 2A. J Virol (2012) 86:10347–58. doi: 10.1128/JVI.00525-12
185. Hsu L-C, Park JM, Zhang K, Luo J-L, Maeda S, Kaufman RJ, et al. The protein kinase PKR is required for macrophage apoptosis after activation of Toll-like receptor 4. Nature (2004) 428:341–5. doi: 10.1038/nature02405
186. Diebold SS, Montoya M, Unger H, Alexopoulou L, Roy P, Haswell LE, et al. Viral infection switches non-plasmacytoid dendritic cells into high interferon producers. Nature (2003) 424:324–8. doi: 10.1038/nature01783
187. Hoffmann JA, Reichhart J-M. Drosophila innate immunity: an evolutionary perspective. Nat Immunol (2002) 3:121–6. doi: 10.1038/ni0202-121
188. Nakajima S, Hiramatsu N, Hayakawa K, Saito Y, Kato H, Huang T, et al. Selective abrogation of BiP/GRP78 blunts activation of NF-κB through the ATF6 branch of the UPR: involvement of C/EBPβ and mTOR-dependent dephosphorylation of Akt. Mol Cell Biol (2011) 31:1710–8. doi: 10.1128/MCB.00939-10
189. Naudé PJW, den Boer JA, Luiten PGM, Eisel ULM. Tumor necrosis factor receptor cross-talk. FEBS J (2011) 278:888–98. doi: 10.1111/j.1742-4658.2011.08017.x
190. Oeckinghaus A, Hayden MS, Ghosh S. Crosstalk in NF-κB signaling pathways. Nat Immunol (2011) 12:695–708. doi: 10.1038/ni.2065
191. Meares GP, Liu Y, Rajbhandari R, Qin H, Nozell SE, Mobley JA, et al. PERK-dependent activation of JAK1 and STAT3 contributes to endoplasmic reticulum stress-induced inflammation. Mol Cell Biol (2014) 34:3911–25. doi: 10.1128/MCB.00980-14
Keywords: vector-borne diseases, vector competence, vector-borne pathogens, arthropod immunity, eukaryotic stress response, integrated stress response, unfolded protein response
Citation: Rosche KL, Sidak-Loftis LC, Hurtado J, Fisk EA and Shaw DK (2021) Arthropods Under Pressure: Stress Responses and Immunity at the Pathogen-Vector Interface. Front. Immunol. 11:629777. doi: 10.3389/fimmu.2020.629777
Received: 15 November 2020; Accepted: 30 December 2020;
Published: 15 February 2021.
Edited by:
Nathalie Boulanger, Université de Strasbourg, FranceReviewed by:
Eric Marois, Institut National de la Santé et de la Recherche Médicale (INSERM), FranceMichael Povelones, University of Pennsylvania, United States
Copyright © 2021 Rosche, Sidak-Loftis, Hurtado, Fisk and Shaw. This is an open-access article distributed under the terms of the Creative Commons Attribution License (CC BY). The use, distribution or reproduction in other forums is permitted, provided the original author(s) and the copyright owner(s) are credited and that the original publication in this journal is cited, in accordance with accepted academic practice. No use, distribution or reproduction is permitted which does not comply with these terms.
*Correspondence: Dana K. Shaw, Dana.Shaw@wsu.edu
†These authors have contributed equally to this work