- 1Olivia Newton-John Cancer Research Institute, and School of Cancer Medicine, La Trobe University, Heidelberg, VIC, Australia
- 2Department of Medicine, University of Melbourne, Parkville, VIC, Australia
A growing number of studies have shown that γδ T cells play a pivotal role in mediating the clearance of tumors and pathogen-infected cells with their potent cytotoxic, cytolytic, and unique immune-modulating functions. Unlike the more abundant αβ T cells, γδ T cells can recognize a broad range of tumors and infected cells without the requirement of antigen presentation via major histocompatibility complex (MHC) molecules. Our group has recently demonstrated parts of the mechanisms of T-cell receptor (TCR)-dependent activation of Vγ9Vδ2+ T cells by tumors following the presentation of phosphoantigens, intermediates of the mevalonate pathway. This process is mediated through the B7 immunoglobulin family-like butyrophilin 2A1 (BTN2A1) and BTN3A1 complexes. Such recognition results in activation, a robust immunosurveillance process, and elicits rapid γδ T-cell immune responses. These include targeted cell killing, and the ability to produce copious quantities of cytokines and chemokines to exert immune-modulating properties and to interact with other immune cells. This immune cell network includes αβ T cells, B cells, dendritic cells, macrophages, monocytes, natural killer cells, and neutrophils, hence heavily influencing the outcome of immune responses. This key role in orchestrating immune cells and their natural tropism for tumor microenvironment makes γδ T cells an attractive target for cancer immunotherapy. Here, we review the current understanding of these important interactions and highlight the implications of the crosstalk between γδ T cells and other immune cells in the context of anti-tumor immunity.
Introduction
For the past 37 years, since the first isolation of the TCR γ gene segment (1, 2), the knowledge accumulated about the γδ T-cell lineage has grown exponentially and received strong clinical interest, especially for cancer immunotherapy development (3–15). Similar to the other two lineages of lymphocytes in the jawed vertebrates that utilize somatically recombined receptors for immunosurveillance (B cells and αβ T cells) (16), TCR heterodimers of γδ T cells are generated through somatic rearrangements of genes encoding for TCR δ chain variable (V), diversity (D), joining (J), and constant (C) gene segments, and TCR γ chain V, J, and C gene segments at the thymus (17, 18). Hypothetically, such diverse gene rearrangements can result in a total of 1017 possible distinct γδ TCRs (19). Despite the diverse theoretical γδ TCR repertoire, human γδ T cells can be classified into two major subsets according to their TCR Vδ chain usage: Vδ2+ populations that are usually paired with Vγ9 chain, and Vδ2− populations with diversified Vγ chain usage (6, 20). Among all 8 TCR Vδ gene segments, Vδ1, Vδ2, and Vδ3 are three commonly used segments for δ chain rearrangement (21, 22).
Vγ9Vδ2+ T cells are the most abundant Vδ cell population found in peripheral blood and are activated by phosphorylated non-protein metabolites called phosphoantigens via the BTN2A1/BTN3A1 complexes in a TCR-dependent manner (3, 11, 23, 24). Phosphoantigens are derived from the mevalonate pathway as an intermediate metabolite known as isopentenyl pyrophosphate (IPP) (25), or are generated in the microbial non-mevalonate isoprenoid synthesis pathway as (E)-4-hydroxy-3-methyl-but-2-enyl-pyrophosphate (HMBPP) (26). Following phosphoantigen binding to the intracellular B30.2 domains of BTN3A1 in tumor or pathogen-infected cells (27), BTN3A1 undergoes a conformational change (28–30) and promotes the interaction between BTN2A1 and BTN3A1 intracellular domains (31). Subsequently, the germline-encoded regions of the TCR Vγ9 chain directly bind to BTN2A1 on tumor cells (3, 32, 33), as described by us and confirmed later by others (34–36). An additional but yet to be identified ligand is likely to bind to a separate region within the complementarity-determining region 2δ (CDR2δ) and CDR3γ of the Vγ9Vδ2 TCR for phosphoantigen-mediated Vγ9Vδ2+ T-cell activation (3, 33). In concert with BTN2A1, the phosphoantigen-induced conformational change of BTN3A1 then leads to Vγ9Vδ2+ T-cell activation (31, 33–36) (Figure 1). Accordingly, dysregulation of the mevalonate pathway in tumors was shown to cause activation of Vγ9Vδ2+ T cells via IPP accumulation (37) and induced γδ T-cell chemotaxis toward tumor cells (38, 39). Activated Vγ9Vδ2+ T cells are capable of inducing cytotoxicity via secretion of Th1 cytokines such as tumor necrosis factor-α (TNF-α) and interferon-γ (IFN-γ), pro-apoptotic protease granzyme B, and cytolytic granules containing pore-forming perforin molecules (40–44). Therefore, many clinical studies used aminobisphosphonates (e.g., zoledronate and pamidronate) to inhibit farnesyl pyrophosphate synthase in the mevalonate pathway to promote accumulation of IPP in cells, or synthetic phosphoantigen analogues such as bromohydrin pyrophosphate (BrHPP) and 2-methyl-3-butenyl-1-pyrophosphate (2M3B1PP), to activate Vγ9Vδ2+ T cells in cancer patients (19, 45–47). In recent years, however, agonist antibodies against BTN3A such as clone 20.1 (48–51), CTX-2026 (52), and ICT-01 (53) have been explored as a phosphoantigen-independent approach to activate Vγ9Vδ2+ T cells for targeted cell killing. Moreover, Vγ9Vδ2+ T cells can be activated by other ligands including human MutS homolog 2, stress-induced MHC class I chain-related antigens A and B (MICA/MICB), UL16-binding proteins (ULBPs), nectin-like-5, staphylococcal enterotoxins (SEs), toxic shock syndrome toxin 1 (TSST-1), and F1-ATPase-apolipoprotein-AI through surface receptors, natural killer group 2D (NKG2D), and DNAX accessory molecule-1 (DNAM-1) (12, 13, 17, 19, 54, 55). Other than direct targeted cell killing, activated Vγ9Vδ2+ T cells have been implicated to directly or indirectly interact with a range of immune cells: αβ T cells (56–63), B cells (64–72), natural killer (NK) cells (73–75), monocytes (76–78), macrophages (79–82), neutrophils (78, 83–86), monocyte-derived dendritic cells (moDCs) (87–93), and DCs (72, 76, 94–96), and influence the outcome of the immune responses. The underlying mechanisms of such γδ T-cell crosstalk with other immune cells are summarized in Table 1 and will be thoroughly discussed in the following sections.
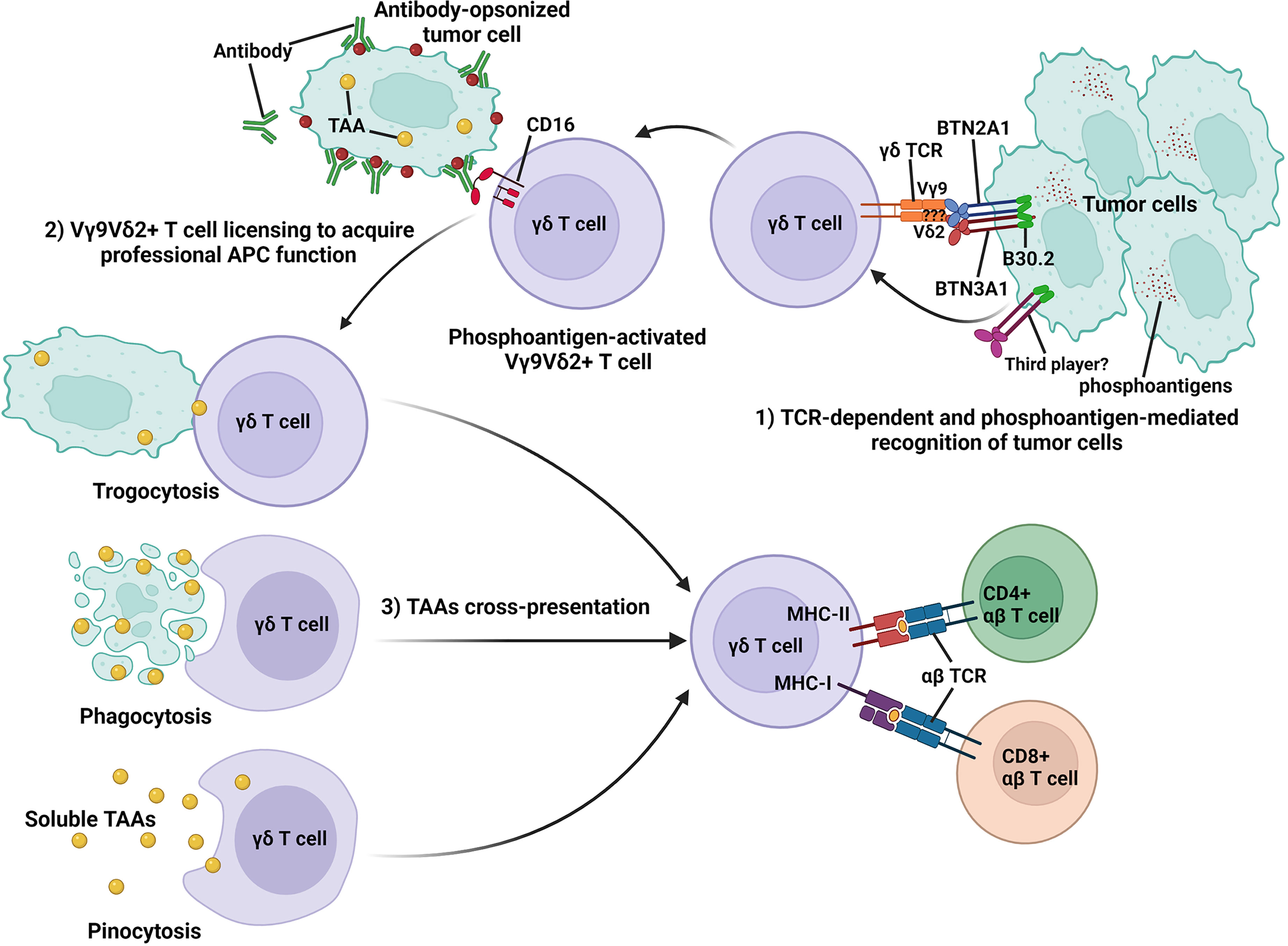
Figure 1 Schematic representation of TCR-dependent and phosphoantigen-mediated recognition of tumor cells by Vγ9Vδ2+ T cells and the acquisition of professional APC function by activated Vγ9Vδ2+ T cells to cross-present TAAs to antigen-specific CD4+ and CD8+ αβ T cells. During the Vγ9Vδ2+ T-cell activation process, accumulated phosphoantigens in tumor cells bind to the intracellular B30.2 domain of BTN3A1. Following phosphoantigen binding, BTN3A1 undergoes conformational changes and induces the interaction between the intracellular domains of BTN2A1 and BTN3A1. BTN2A1 directly binds the TCR Vγ9 chain and leads to T-cell activation in concert with at least one additional ligand. Activated Vγ9Vδ2+ T cells can recognize antibody-opsonized tumor cell via CD16 (FcγRIII) and are licensed to acquire professional APC function via trogocytosis, phagocytosis, and pinocytosis and cross-present antigens from tumor cells to antigen-specific CD4+ and CD8+ αβ T cells.
The non-Vδ2 γδ T cells are mostly identified with Vδ1+ or Vδ3+ TCR chain usage and are localized in the skin, large intestine, spleen, and liver (6, 12, 54). Several studies have shown that Vδ1+ γδ T cells recognize CD1c-phosphomycoketide (110), CD1d-α-GalCer (111), CD1d-sulfatide (112, 113), R-phycoerythrin (PE) (114), ephrin receptor A2 (EphA2) (115), and MHC-related protein 1 (MR1) (116) ligands, and play a crucial role for anti-tumor responses (117–124). Similar to Vδ2+ γδ T cells, the NKG2D-expressing Vδ1+ γδ T cells can be activated by stress-inducible MICA/MICB and ULBP1–6 family proteins, which are frequently upregulated in tumor cells (8, 11). Ligand-bound NKG2D induces cytolytic functions of γδ T cells via granzyme B and perforin secretion to mediate tumor cell killing (125). Several studies have utilized Vδ1+ γδ T-cell populations for adoptive cancer immunotherapy (8, 10, 126), but the clinical outcome so far was limited. The less frequent Vδ3+ γδ T cells were shown to recognize and kill CD1d+ target cells (109) and are activated by annexin A2 ligands on tumor cells that are upregulated under oxidative stress conditions (127). Interestingly, the binding affinity of the Vδ1+ and Vδ3+ γδ TCR ligands identified thus far falls within the range of 3 to 150 µM (55, 128), comparable to the well-studied αβ TCR binding affinities for the peptide–MHC complex (129, 130), suggesting a possible shared TCR docking footprint on the bound ligand (131). With the increasing numbers of non-Vδ2 γδ T-cell ligands uncovered so far (8, 55, 116, 132), different strategies have been developed to utilize activated non-Vδ2 γδ T cells for cancer immunotherapy (10, 19, 128). Of note, activated non-Vδ2 γδ T cells have also been implicated to modulate other immune cells (Table 1) including αβ T cells (102), B cells (133–135), DCs (89, 102–107, 109, 136, 137), macrophages (70, 138), and neutrophils (139).
Human Vδ2+ γδ T cells represent ~0.5% to 10% of all circulating T lymphocytes in healthy adults and can undergo rapid expansion of up to 60% in the periphery during infections, and form between 20% to 30% of total infiltrating CD3+ T cells in the early stage of disease onset (11, 17). Activated Vδ1+ and Vδ2+ γδ T cells upregulate various C-C chemokine receptor (CCR) such as CCR1 and CCR8 (140), CCR2 (141), CCR5 (142), and C-X-C chemokine receptor 3 (CXCR3) (107) to mediate infiltration into the tumor microenvironment (TME). Additionally, tumor cells and tumor-derived fibroblasts express chemokine ligand 2 (CCL2) (141), IFN-γ-inducible protein 10 (IP-10) (107), monocyte chemoattractant protein 1 (MCP-1), macrophage inflammatory protein 1α (MIP-1α), MIP-1β, and regulated on activation, normal T cell expressed and secreted (RANTES) to promote recruitment of activated Vδ1+ and Vδ2+ γδ T cells to the TME (140). Once recruited into the TME, tumor-infiltrating Vδ1+ and Vδ2+ γδ T cells can eliminate tumor cells via TNF-related apoptosis-inducing ligand (TRAIL) (143), Fas/Fas ligand pathway (144), induction of antibody-dependent cellular cytotoxicity (ADCC) on antibody-opsonized tumor cells through CD16 (FcγRIII) (60, 145, 146), perforin/granzymes, IFN-γ/TNF-α secretion, and NKG2D-mediated cytotoxicity (13, 147). As a result of the complex interplay between TME and tumor-infiltrating γδ T cells, activated γδ T cells can be functionally polarized to become the anti-tumor Th1 and follicular Th (Tfh) cells or the pro-tumor Th17 and T regulatory (Treg) cells (12, 132). For example, IPP-activated Vγ9Vδ2+ T cells can be polarized into three distinct subsets based on the presence of different cytokines in the microenvironment: Th1 [interleukin-12 (IL-12) and anti-IL-4 antibody] (148), Th2 (IL-4 and anti-IL-12 antibody) (148), and Th17 [IL-1β, transforming growth factor β (TGF-β), IL-6 and IL-23] (149). Recent reviews on the topic of γδ T-cell polarization has provided comprehensive insight into the different role of γδ Th1, Th2, Th17, Tfh, and Treg cells, and we refer readers to these excellent publications (7, 8, 11, 54, 150–153).
Importantly, the presence of tumor-infiltrating γδ T cells was shown to be the most favorable prognostic marker for overall cancer patients survival in 25 different cancer types and solid tumors (non-brain tumor) (4). Their role in cancer immunosurveillance was clearly evidenced and validated in many tumor models and clinical studies including cutaneous carcinoma (154), melanoma (119, 155, 156), lymphoma (157–159), leukemia (44, 117, 160, 161), gastric (162), colorectal (43, 163, 164), kidney (41), prostate (165, 166), and pancreatic (143) cancers. The ability of γδ T cells to produce large quantities of cytokines and chemokines rapidly and their tendency to reside in blood circulation or in non-lymphoid tissues (e.g., skin, intestines, and lungs) (8, 16, 17), helps to provide the first line of immunosurveillance against aberrant cell growth and infectious diseases, and bridges the innate and adaptive immune responses. Thus, it is important to understand the crosstalk between γδ T cells and other immune cells in the TME and to harness this knowledge for effective cancer immunotherapy development.
Crosstalk between γδ T cells and αβ T cells
The role of antigen processing and presentation to αβ T cells is mostly associated with the classical professional antigen-presenting cells (APCs) like DCs, macrophages, and B cells (167, 168). However, with the unexpected discovery by Brandes et al., it was shown that activated but not resting human Vγ9Vδ2+ T cells were also capable of acquiring professional APC functions (56). Indeed, activated Vγ9Vδ2+ T cells isolated from both healthy individuals and cancer patients’ peripheral blood mononuclear cell (PBMC) exhibited potent APC functions to stimulate robust antigen-specific αβ T-cell responses (169).
During the activation process, human Vγ9Vδ2+ T cells can rapidly gain APC functions by upregulating co-stimulatory (CD40, CD80, and CD86), MHC class I and II molecules (56, 57, 61, 62, 97, 108, 169), and transiently expressed lymph node-homing markers, chemokine receptor CCR4 and CCR7 (62, 68, 97). This allows recruitment of activated γδ T cells from the peripheral sites to secondary lymphoid tissues for antigen presentation and bridges the early phase of rapid innate-like γδ T-cell response to microbial or tumor antigens with the later phase of adaptive immune response involving the antigen-specific CD4+ and CD8+ αβ T cells (14, 15, 17, 168, 170). In a study by Himoudi et al., it was shown that activated human Vγ9Vδ2+ T cells were “licensed” to acquire their APC functions through recognition of antibody-opsonized tumor cells, mediated targeted cell killing by their innate cytotoxicity, and subsequently helped to release tumor-associated antigens (TAAs) into the surrounding microenvironment (60). These TAAs can be taken up by activated γδ T cells via phagocytosis (62, 97, 98, 108), trogocytosis (171), or pinocytosis (57, 58), processed and presented on the cell surface for priming and induction of naïve αβ T cells (59, 60) (Figure 1). Furthermore, it was shown that Vγ9Vδ2+ γδ T cells can uptake microbes and soluble antigens via CD16-mediated phagocytosis, a process that can lead to functional antigen processing and presentation on MHC class II (98), and cross-presentation of immunodominant MHC class I peptides to antigen-specific CD8+ αβ T cells (58, 60, 61). This notion was further supported by the identification of Vγ9Vδ2+ T cells in malaria patients that readily acquired APC functions upon infection and induced CD4+ and CD8+ αβ T-cell activation (61). Interestingly, it was also demonstrated that activated Vγ9Vδ2+ T cells can uptake CD1d-containing membrane fragments from phosphoantigen expressing Cd1d+ target cells via trogocytosis, leading to the presentation of CD1d-restricted antigen and the activation of Vα24Vβ11+ invariant natural killer T cells (iNKT) (172).
When compared to activated αβ T cells and monocytes, activated Vγ9Vδ2+ T cells were shown to be more efficient in presenting antigens and induced 100-fold higher proliferative responses in naïve CD4+ αβ T cells (56). Activated Vγ9Vδ2+ T cells were also able to cross-present antigens to CD8+ αβ T cells with a higher efficiency and reproducibility (57), and induced less CD4+ CD25hi FoxP3+ Treg cell expansion than moDCs (59). Similar results were seen under pathological condition, when it was shown that γδ T cells isolated from gastric cancer patients can acquire APC functions upon activation with cells derived from autologous tumor tissues (99). These clinically relevant tumor-activated γδ T cells induced strong antigen-specific CD4+ and CD8+ αβ T-cell responses and prevented immunosuppression mediated by CD4+ CD25+ Treg cells (99) (Figure 2). Of note, Muto et al. showed that resting Vγ9Vδ2+ T cells can significantly upregulate the expression of scavenger receptor CD36 during activation and that this was mediated by a key transcription factor, CCAAT/enhancer-binding protein α (C/EBPα), that supports acquisition of APC functions in activated Vγ9Vδ2+ T cells (108). In contrast, resting αβ T cells expressed a low level of CD36 and did not upregulate it upon activation (108). In DCs and macrophages, the CD36 receptor was shown to facilitate the uptake of apoptotic cells and cross-presentation (173, 174), potentially explaining the induction of a stronger antigen-specific αβ T-cell response by activated Vγ9Vδ2+ T-cell APC.
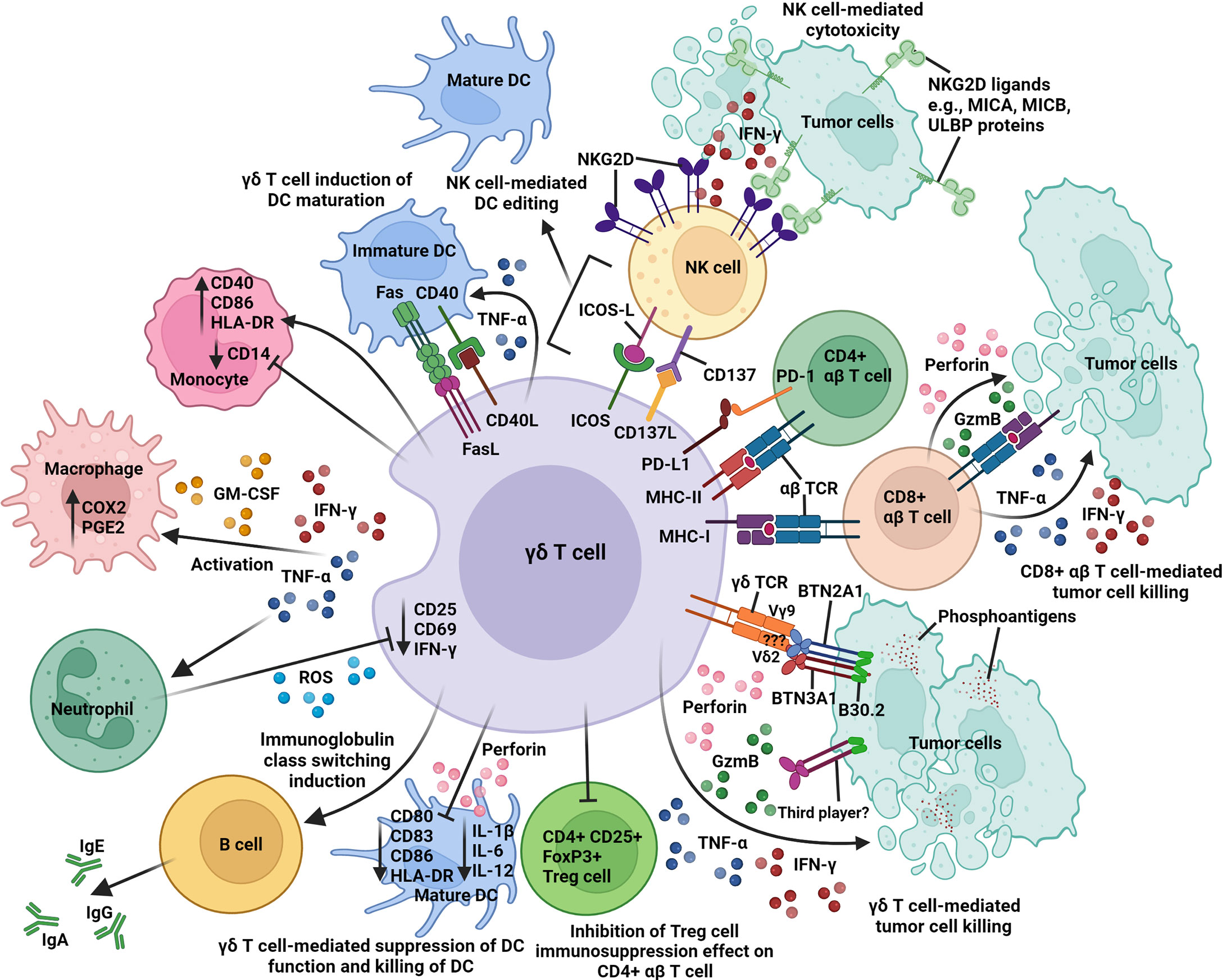
Figure 2 An overview of the intricate network of immune interactions between γδ T cell and other immune cells in the tumor microenvironment. Activated γδ T cells express different surface receptors and molecules (γδ TCR, ICOS, MHC class I and II), ligands (CD40L, CD137L, FasL, and PD-L1), cytokines (IFN-γ and TNF-α), and GM-CSF for contact-dependent and independent crosstalk with tumor cells, CD4+ and CD8+ αβ T cells, NK cells, DCs, macrophages, and neutrophils. Activated γδ T cells cross-present antigens to CD4+ and CD8+ αβ T cells; induce B-cell immunoglobulin class switching; co-stimulate NK cells via CD137/CD137L and ICOS/ICOS-L interactions; induce upregulation of CD40, CD86, and HLA-DR expression on monocyte; promote DC maturation via CD40/CD40L and Fas/FasL interactions; and inhibit the immunosuppression function of CD4+ CD25+ FoxP3+ Treg cells on CD4+ αβ T-cell activity. In contrast, activated γδ T cells can also suppress DC function (downregulation of CD80, CD83, CD86, HLA-DR, IL-1β, IL-6, and IL-12) and mediate DC killing via perforin release. Butyrophilin 2A1 and 3A1 (BTN2A1 and BTN3A1); cyclooxygenase-2 (COX2); granulocyte-macrophage colony stimulating factor (GM-CSF); granzyme B (GzmB); human leukocyte antigen-DR (HLA-DR); immunoglobulin A, E, or G (IgA, IgE, or IgG); inducible T-cell co-stimulator (ICOS); ICOS ligand (ICOS-L); interferon-γ (IFN-γ); major histocompatibility complex class I and II (MHC-I and -II); MHC class I chain-related antigens A and B (MICA and MICB); natural killer group 2D (NKG2D); programmed cell death 1 (PD-1); PD-1 ligand 1 (PD-L1); prostaglandin E2 (PGE2); reactive oxygen species (ROS); T-cell receptor (TCR); tumor necrosis factor-α (TNF-α); UL16-binding protein (ULBP).
The ability to migrate to the tumor site and cross-present TAAs to αβ T cells was also retained when Vδ1+ and Vδ2+ γδ T cells were engineered to express tumor-specific chimeric antigen receptors (CARs) and resulted in an increased cytotoxic level against tumor cells (175). Hence, activated Vγ9Vδ2+ T cells can process and present antigens and provide critical co-stimulatory signals to prime and induce naïve CD4+ (56) and CD8+ (57) αβ T cells for proliferation, differentiation, and cytokine production and to mediate cytotoxic responses against tumors and pathogen-infected cells (176–179). This remarkable ability of γδ T cells to uptake and present antigens and prime αβ T cells has been highlighted by Vantourout et al. (168), and the accumulated data so far have illustrated the potential of harnessing the APC functions of γδ T cells to crosstalk with αβ T cells for immunotherapy development.
Given their natural tropism for TME (14, 119, 175, 180–182), activated γδ T cells could hence be utilized to prolong the intratumoral immune response by cross-presenting TAAs to other tumor-infiltrating lymphocytes and provide an early source of IFN-γ to expand and increase immunogenicity of TAA-specific αβ T cells within the TME (155, 183, 184), and to upregulate expression of MHC class I and II on tumor cells (185, 186) for αβ T cell-mediated killing (Figure 2). The presence of tumor-infiltrating γδ T cells within the TME as revealed by genomic data analysis in over 18,000 human tumors has uncovered a strong correlation to good prognosis (4). In the context of cancer immunotherapy, the capability of activated γδ T cells to cross-present TAAs to αβ T cells could be further boosted through the “licensing” pathway (60, 187) by using therapeutic monoclonal antibodies against tumor cells, e.g., rituximab (anti-CD20) and trastuzumab (anti-HER2/neu) (145, 188, 189). Such combination treatment could greatly improve the outcome of γδ T-cell cancer immunotherapy.
Activated Vγ9Vδ2+ T cells can also modulate αβ T-cell activity indirectly by co-stimulating NK cells via inducible T-cell co-stimulator (ICOS)/ICOS-L and CD137/CD137L engagements to enhance IFN-γ and TNF-α production (100, 101), which, in turn, helps to support αβ T-cell activation (190). Another study has shown that activated Vγ9Vδ2+ T cells can induce B-cell and DC maturation and subsequently leads to alloreactive stimulation of αβ T-cell proliferation and IFN-γ production by mature B cells and DCs (72). The interactions between γδ T cells and other immune cells (B cells, DCs, and NK cells) will be discussed later in this review.
Despite their ability to exert positive immune modulation functions on αβ T cells, activated γδ T cells can also negatively regulate αβ T-cell response by upregulating an immune checkpoint inhibitory ligand, programmed cell death 1 ligand 1 (PD-L1) (11, 151, 191). The suppressive phenotype of activated Vδ2+ γδ T cells on autologous αβ T cells was shown to be mediated by the PD-1/PD-L1 interactions and correlated well with the strength of Vδ2+ γδ TCR signaling during the activation process but was independent of TGF-β and FoxP3 expression (192) (Figure 2). Daley et al. showed that tumor-infiltrating γδ T cells with high expression levels of checkpoint inhibitory ligands PD-L1 and Galectin-9 could inhibit αβ T-cell activation through checkpoint receptor ligation (193). The immunosuppressive effect can also be mediated by the interaction between CD86 on activated Vδ2+ γδ T cell and cytotoxic T lymphocyte-associated antigen 4 (CTLA-4) on activated αβ T cells (191). Such γδ T cell-mediated immunosuppression of αβ T cells, however, can be significantly reduced by disrupting PD-1/PD-L1 and CTLA-4/CD86 interactions with blocking antibodies (191, 192). Furthermore, Peng et al. identified tumor-infiltrating Vδ1+ γδ T cells that could suppress naïve/effector αβ T-cell proliferation and IL-2 production through the Toll-like receptor (TLR) 8 signaling pathway and may lead to tumor immune escape (102). The immunosuppressive activity of Vδ1+ γδ T cells can be reversed using TLR8 ligands, and this signaling involved the myeloid differentiation primary response 88 (MyD88), TNFR-associated factor 6 (TRAF6), IKB kinase α (IKKα), IKKβ, and mitogen-activated protein kinase 14 (MAPK14), but not transforming growth factor-β-activated kinase 1 (TAK1), Jun N-terminal kinase (JNK), and extracellular signal-regulated kinase (ERK) molecules in Vδ1+ γδ T cells (102). It was also reported that γδ+ NKG2A+ intraepithelial lymphocytes (IELs) can mediate suppression of CD8+ αβ+ IEL cytotoxic responses (IFN-γ and granzyme B) in patients with celiac disease through TGF-β secretion (194). The immunosuppressive effect on CD8+ αβ+ IELs can be further enhanced upon γδ+ IELs NKG2A receptor ligation with the cognate ligand, human leukocyte antigen-E (HLA-E) (194). This immunosuppressive effect can be reduced by blocking NKG2A/HLA-E interaction and TGF-β with blocking antibodies (194). Therefore, it is important to consider these negative immunomodulatory roles of γδ T cells when designing novel immunotherapeutics.
Apart from the PD-1/PD-L1 and CTLA-4/CD86 immune checkpoint axes, other non-conventional checkpoint receptors [killer Ig-like inhibitory receptors (KIRs), Ig-like transcript 2 (ILT-2), and NKG2A] can be expressed on Vγ9Vδ2+ T cells, inhibit their cytotoxic function, and prevent tumor cell lysis upon recognition of specific HLA class I ligands on tumor cells (195–203). In this context, the presentation of HLA class I molecules on tumor cells can be a double-edged sword. On one hand, it facilitates the presentation of antigenic peptides to activate CD8+ αβ T cells, but at the same time, it can also inhibit the activation of Vγ9Vδ2+ T cells. Such inhibitory signals on immune cells mediated by KIRs, ILT-2, or NKG2A can be blocked using monoclonal antibodies targeting KIRs (lirilumab and IPH4102), ILT-2 (anti-ILT-2, anti-HLA-G1, anti-FasL), or NKG2A (monalizumab) (204, 205). In a study by André et al., treatment with monalizumab indeed led to enhanced anti-tumor immune responses elicited by T and NK cells (206). As a type 2 inhibitory membrane receptor, NKG2A carries cytoplasmic immunoreceptor tyrosine-based inhibitory motifs (ITIMs) and forms heterodimers with CD94 to recognize non-classical HLA-E molecule (207). Many human tumors have been shown to express HLA-E including in the colon, cervical, endometrial, head and neck, liver, lung, pancreas, ovarian, and stomach (206). Moreover, a majority of Vγ9Vδ2+ T cells in healthy individuals express NKG2A/CD94 (197, 198, 200, 208), and the expression levels can be induced by IL-15 and TGF-β (209, 210). Therefore, treatments targeting these non-conventional checkpoint receptors on Vγ9Vδ2+ T cells (KIRs, ILT-2, and NKG2A) to disrupt the interactions with their respective HLA class I ligands on tumor cells (HLA-C, HLA-G, and HLA-E) may help to enhance the effectiveness of Vγ9Vδ2+ T cell-based tumor immunotherapy.
Recent work by Payne et al. suggests that BTN3A, itself part of the molecular complex required for phosphoantigen-mediated activation of Vγ9Vδ2+ T cells, can also inhibit tumor-reactive CD8+ αβ T cells when bound to N-mannosylated residues of CD45 by preventing its segregation from the immunological synapse (52). In this study, the suppression of αβ T-cell activation was shown to involve BTN3A1 but not BTN2A1, and the immunosuppressive effect could be blocked by BTN3A1-specific monoclonal antibodies such as clone 20.1, 103.2, and CTX-2026 (52). Targeting BTN3A1 with the agonistic antibody CTX-2026 induced BTN3A1 switching from immunosuppressive to immunostimulatory conformations and promoted coordinated Vγ9Vδ2+ and CD8+ αβ T-cell anti-tumor responses against BTN3A1+ tumors (52). Hence, BTN3A1 may be an attractive immune target for intervention to orchestrate effective and coordinated γδ and αβ T-cell anti-tumor responses.
Crosstalk between γδ T cells and B cells
γδ T cells have been previously reported to interact with B cells and modulate their immune functions (5, 8, 168, 211, 212). Vγ9Vδ2+ T cells can adopt a role similar to T follicular helper (Tfh) cells and provide B-cell help, thereby regulating B-cell maturation. Specifically, a subset of CXCR5+ Vγ9Vδ2+ T cells present in circulation and in tonsil tissue expresses co-stimulatory molecules (ICOS and CD40L) upon antigen stimulation and secrete cytokines (IL-2, IL-4, and IL-10), which can promote the development of antibody-producing B cells via immunoglobulin class switching [including immunoglobulin A (IgA), IgE, IgG1, IgG2, IgG3, and IgG4] (8, 213, 214) in the extra-follicular or within germinal centers (65–67, 69) (Figure 2). Furthermore, upon stimulation with IL-21 and HMBPP, activated tonsillar Vγ9Vδ2+ T cells can express CXCL13 receptor, CXCR5, induce lymphoid-homing phenotype and clustering in germinal centers, and sustain the production of germinal centers (70, 71). Similarly, IPP-stimulated Vδ2+ γδ T cells with functional CCR7 expression can also induce transient lymph node-homing, migration, and clustering of Vδ2+ γδ T cells within B-cell zones of germinal centers in lymphoid tissues (64, 68).
Phosphoantigen-activated Vδ2+ γδ T cells can additionally induce the expression of B-cell co-stimulatory molecules (CD40L, OX40, CD70, and ICOS) and affect the downstream production of circulating IgA, IgG, and IgM antibodies by B cells (68). In patients with specific mutations (RAG1 and CD3D) that impair αβ T-cell function, γδ T cells are responsible for hyper-IgE syndromes or the elevated production of circulating IgA, IgG, and IgM (215, 216). γδ T cells can also suppress antibody responses via the induction of CD4+ Foxp3+ Treg cells (217). Conversely, some B cells can express BTN2A1 and BTN3A1, required for Vγ9Vδ2+ T-cell activation (33–35), thereby directly influencing Vδ2+ γδ T-cell activation (218, 219) as shown by early studies using Daudi cells, a B-cell malignancy cell line (Burkitt’s lymphoma) (220–226). Vγ9Vδ2+ T cells can directly engage BTN2A1 expressed on B cells via the TCR Vγ9 chain (3, 32–36, 227), and in concert with BTN3A1, this results in Vγ9Vδ2+ T-cell activation and expansion (101, 212). Hebbeler et al. showed that the Vγ9Vδ2+ T cells activated and expanded by phosphoantigen or Daudi B lymphoma cells use public TCR Vγ9 clonotypes, and elicit comparable cytotoxic responses against tumor cells (228). Further investigations revealed that the germline-encoded region between TCR Vγ9 CDR2 and CDR3 is responsible for contacting BTN2A1 on target cells (33, 34). Such findings indicate the inherent property of TCR Vγ9 to recognize diverse range of cell types that express BTN2A1 including B cells (212, 227–229). In addition to BTN2A1 and BTN3A1, B cells also express other closely related BTN molecules such as BTN3A2 (in naïve or germinal center B cells), BTN3A3 (in memory B cells), BTN1A1, and BTN2A2 (3, 50). The contribution of these other BTN molecules in B cells for γδ T-cell activation remains elusive. Similarly, circulating activated B7+ CD39+ B cells can stimulate Vδ1+ γδ T-cell proliferation (133, 134). The Vδ1+ γδ T-cell stimulatory ligand is upregulated in B cells upon activation and can induce polyclonal Vδ1+ γδ T-cell responses (133). This B cell-mediated immunostimulatory effect on Vδ1+ γδ T cells can be blocked with antibodies against B7 and CD39 (133, 212).
In summary, Vγ9Vδ2+ T cells can regulate B-cell maturation during development or initiation of an immune response, sustain the production of germinal centers within secondary and possibly tertiary lymphoid structures, and affect the production of circulating (auto)antibodies for humoral immunity (168, 211, 212), while B cells can activate Vδ1+ and Vγ9Vδ2+ T cells (230).
Crosstalk between γδ T cells and NK cells
Human NK cells are important innate immune subset for controlling early tumor growth and metastasis through cell-mediated cytotoxicity and show broad reactivity to tumors that escaped immunosurveillance by loss or aberrant MHC class I expression (14, 231, 232). Being a specialized group of innate lymphoid cells (ILCs), NK cell functions are closely regulated by a range of cytokines such as IFN-γ, TNF-α, IL-2, IL-12, IL-15, IL-18, and IL-21 (233, 234). These effector molecules are important for the initiation of anti-viral and anti-tumor immune responses (235–238). However, more established tumors can evade NK cell surveillance by developing resistance to NK cell-mediated cytotoxicity, leading to tumor immune escape (239).
In order to overcome NK-resistant tumors, Maniar et al. showed that activated human NK cells (CD25hi, CD54hi, CD69hi, and CD137hi) increased surface expression of natural NKG2D receptors to promote tumor cytolysis and death (73). NKG2D is a lectin-like type 2 transmembrane receptor mostly expressed by human NK cells and binds to MHC-related ligands such as ULBPs, MICA, and MICB, which are highly expressed in tumor cells but rarely in healthy cells (231, 240). IPP-activated Vδ2+ γδ T cells upregulate CD137L (4-1BBL), engage with CD137+ NK cells, and can in turn lead to enhanced NKG2D expression and NK cell-mediated cytotoxicity against tumors (73) (Figure 2), highlighting a potential key role for γδ T cells in this process. CD137 or 4-1BB is a member of the tumor necrosis factor receptor superfamily (TNFRSF) and is expressed by a range of immune cells (190). Expression of CD137 on NK cells is induced by IL-2 and IL-15, and following CD137 signaling, it promotes NK cell proliferation and production of IFN-γ, which, in turn, can support NK tumor effector functions (101, 190). This finding was further corroborated by Liu et al., and they demonstrated that in the context of liver fibrosis, γδ T cells engaged with conventional and liver-resident NK cells through CD137/CD137L interactions to promote NK cell-mediated cytotoxicity against activated hepatic stellate cells and conferred immune protection (75).
Similar to NK and CD8+ αβ T cells, human γδ T cells also express NKG2D to detect stress-inducible ligands on tumors and pathogen-infected cells (125, 241–245). Several studies have shown that NKG2D ligation to its cognate ligand can co-stimulate Vγ9Vδ2+ T-cell activation (CD25 and CD69 upregulation) and promotes the release of IFN-γ, TNF-α, and cytolytic granules to mediate killing of NKG2D ligand-expressing tumors (163, 246–251). In the context of leukemia and lymphoma cell recognition by Vγ9Vδ2+ T cells, it was reported that tumor-expressed ULBP1 was a strong marker for tumors susceptible to Vγ9Vδ2+ T cell-mediated cytotoxicity (252). Similarly, it was shown that ULBP1 overexpression in tumor cells can lead to enhanced killing by Vγ9Vδ2+ T cells (253). Hence, blocking NKG2D-mediated Vγ9Vδ2+ T-cell recognition of tumor cells with anti-NKG2D and anti-MICA/B monoclonal antibodies inhibits tumor cell killing to varying degrees (247, 249, 253). Vδ1+ γδ T cells can also recognize and kill NKG2D ligand-expressing tumors via NKG2D receptor (8, 11, 245, 254). The number of Vδ1+ γδ T cells and ULBP3 expression level are negatively correlated with disease progression in chronic lymphocytic leukemia patients (254). A study reported by Kamei et al. demonstrated a longer overall survival in gastric cancer patients with high expression levels of NKG2D and ULBP1 (255). Hence, upregulation of stress-inducible NKG2D ligand in tumor cells and NKG2D receptor in tumor-infiltrating immune cells can help to orchestrate concerted NKG2D-mediated NK, CD8+ αβ, and γδ T-cell anti-tumor responses within the TME. Of note, several anti-cancer drugs have been found to induce expression of NKG2D ligand in tumor cells, including the proteasome inhibitor bortezomib and the alkylating agent temozolomide, and these can help to promote tumor cell lysis by NK and γδ T cells (256, 257). Therefore, it is feasible to target NKG2D and its ligands for γδ T cell-based immunotherapy development.
It was later shown that IPP-activated Vγ9Vδ2+ T cells can upregulate ICOS and signal NK cells via ICOS/ICOS-L engagement to promote CD69 and CD137 expression, which then leads to enhanced production of IFN-γ, TNF-α, MIP-1β, I-309, RANTES, and soluble Fas ligand by activated NK cells (100). Such ICOS/ICOS-L-mediated crosstalk enables NK cells to acquire the “license” to kill mature DCs that may play a role in inflammation and tumor growth (100). These studies have uncovered the immunomodulatory role of IPP-activated Vγ9Vδ2+ T cells to circumvent NK-resistant tumors and to promote NK-mediated DC editing function by modulating NK cell cytotoxicity through CD137/CD137L and ICOS/ICOS-L engagements (73, 101) (Figure 2). Such findings will provide an alternative strategy for γδ T cell-based immunotherapy development against difficult-to-treat solid tumors or to prevent metastasis (239, 258, 259).
However, NK cell activity can also be negatively regulated by γδ T cells. Zoledronate-activated Vδ2+ γδ T cells not only can co-stimulate early NK cell activation for IFN-γ production but also lead to premature ending of the response by inducing cytotoxicity against CD56+ DC-like cells (74). In the absence of activated Vδ2+ γδ T cells, CD56+ DC-like cells survived (74) and maintained NK cell activity through secretion of NK cell-activating cytokines such as IL-1β and IL-18 (260, 261). Therefore, further studies will help to provide a better understanding of the immunosuppressive role of Vδ2+ γδ T cells on NK cells.
Crosstalk between γδ T cells and monocytes/macrophages
γδ T cells share many of their innate functions with other immune cell subsets, including NK cells, monocytes, and macrophages (56, 98, 262, 263). These are integral to the innate inflammatory response against infectious pathogens and tumors, which, in turn, activates a strong and targeted adaptive immune response (170). While the hallmark of Vγ9Vδ2+ T cell is recognition of phosphoantigens produced by bacteria-infected or tumor cells (25, 264), monocytes are adept at potentiating this process by taking up and accumulating phosphoantigen for subsequent presentation to γδ T cells (262, 263). Conversely, the prototypical roles of myeloid cells, such as phagocytosis and MHC class II presentation, are also shared by Vγ9Vδ2+ T cells, which can act as professional APCs (56, 98). The close interconnection between these cell types and partial redundancy in functional properties denotes multiple implications for tumor immunity.
Vγ9Vδ2+ T cells have been shown to activate monocytes, induce adhesion and aggregation, and increase their survival (76, 265). This occurs via production of inflammatory molecules including IFN-γ, TNF-α, granulocyte-macrophage colony stimulating factor (GM-CSF), lymphocyte function-associated antigen 1 (LFA-1), and CCL2 (76, 78). In turn, this leads to changes in monocyte markers such as downregulation of CD14, and upregulation of CD40, CD86, and HLA-DR (76, 77) (Figure 2). Bidirectionally, zoledronate- or HMBPP-primed monocytes can activate Vγ9Vδ2+ T cells through phosphoantigen accumulation and presentation, leading to γδ T-cell proliferation and bacterial pathogen killing (76, 263). However, in vitro, it has also been reported that in the presence of zoledronate, monocytes and Vδ2+ γδ T cells can negatively regulate each other by inducing apoptosis (266, 267). It is interesting to note that the contact-dependent stimulation of Vγ9Vδ2+ T cells by monocytes via the intercellular adhesion molecule 1 (ICAM-1)/LFA-1 engagement can be disrupted by blocking CD11a with monoclonal antibody (78). In contrast to these in vitro results, in vivo treatment with zoledronate or other aminobisphosphonates has shown varying effects, with some studies reporting an increase in circulating monocyte numbers, while others found no difference (77, 268). This suggests that the relationship between these cells may be more nuanced and context-dependent than first thought and will require further investigation.
The crosstalk between γδ T cells and macrophages has not yet been thoroughly elucidated; however, the effects are again cell subtype- and context-dependent. Macrophages have been demonstrated to recruit Vγ9Vδ2+ T cells to the site of infection via IP-10 and CXCR3 receptor–ligand interactions (80). Once this occurs, Vδ2+ γδ T cells can drive the local cytotoxic response via granzyme and perforin release or Fas ligand binding (79, 81, 82). Both Vδ1+ cells and Vδ2+ cells have been shown to produce CCL3, CCL4 (MIP-1α and MIP-1β), and CXCL10, which find their respective cognate receptors expressed by macrophages (70, 138). In vitro, the supernatant of cultured γδ T cells has been shown to induce macrophage activation via IFN-γ, TNF-α, and GM-CSF production, arguing for a tightly regulated and balanced interplay between these immune cell populations (265). This was further demonstrated by studies showing that IFN-γ and TNF-α released by activated Vγ9Vδ2+ T cells can induce cyclooxygenase-2 (COX2) expression and prostaglandin E2 (PGE2) release by both macrophages (Figure 2) and tumor cells, and this downregulates the cytotoxic response of γδ T cells (269, 270) and plays a major role in tumor immune escape (271, 272). Furthermore, galectin-9 on both γδ T cells and pancreatic tumor cells has been shown to bind dectin-1 on tumor-infiltrating macrophages, leading to M2 macrophage polarization and subsequent downregulation of IFN-γ and TNF-α production by γδ T cells (273, 274).
Crosstalk between γδ T cells and neutrophils
Neutrophils are another immune cell population with complex interactions with γδ T cells at peripheral sites of inflammation and in the TME. Zoledronate-activated Vγ9Vδ2+ T cells release cytokines and chemokines such as IFN-γ, TNF-α, IL-6, and MCP-2, and these have been demonstrated in vitro to induce neutrophil migration, activation, phagocytosis, degranulation, and release of α-defensins (83). In a differing context using a bacterial phosphoantigen, HMBPP-activated Vγ9Vδ2+ T cells produce CXCL8 and TNF-α, which together mediate neutrophil recruitment, induce CD11b upregulation and prevent apoptosis, and downregulate CD62L, allowing neutrophil adhesion (78). This finding was further corroborated by Sabbione et al., showing that HMBPP-activated Vδ2+ γδ T cells can stimulate CD11b expression and myeloperoxidase production by neutrophils (86), all of which imply a stimulatory role of γδ T cells towards these granulocytes. In another study, tissue-resident Vδ1+ γδ T cells were shown to regulate the recruitment of neutrophils to the site of bacterial infection via IL-17 secretion (275). In the absence of Vδ1+ γδ T cells, the production of IL-17 is reduced and leads to lower numbers of neutrophil recruitment to the site of infection (275).
Interestingly, activated neutrophils can inhibit CD25 and CD69 expression, IFN-γ production, and cell proliferation of Vδ2+ γδ T cells either spontaneously or in response to HMBPP (86). This is dependent on initial TNF-α production by γδ T cells, which then induces reactive oxygen species (ROS) secretion from neutrophils (86) (Figure 2). These processes can be independent of cell–cell contact; however, the inhibition is more potent if cells are allowed to interact and form conjugates (86). Neutrophils can take up zoledronate, and despite also expressing BTN2A1 and BTN3A1, they do not have the capability of activating Vγ9Vδ2+ T cells, which may be due to their extremely limited production and accumulation of IPP (276–278). Rather, these zoledronate-activated neutrophils inhibit TNF-α and IFN-γ production and proliferation of Vγ9Vδ2+ T cells via ROS, arginase-1, and serine protease production. Some serine proteases are also able to downregulate BTN3A1 expression on PBMCs, which has downstream consequences for BTN-mediated activation of Vδ2+ γδ T cells (84, 85). Furthermore, Vδ1+ γδ T cells have been shown to exhibit reduced proliferation in the presence of hydrogen peroxide as well as decreased glutathione production, which may be indicative of ROS-dependent neutrophil inhibition (139). In some instances, however, neutrophils that have phagocytosed HMBPP-producing bacteria subsequently release HMBPP, which is then able to activate Vγ9Vδ2+ T cells. This results in CD25, CD69, LFA-1, IFN-γ, and TNF-α production and is crucial for initiating an immediate anti-inflammatory response (78).
Functionally, pancreatic tumor cell killing by γδ T cells within a PBMC context is decreased in the presence of neutrophils, in both unstimulated and zoledronate-activated conditions (279). However, when pancreatic tumor cells are co-cultured with purified, expanded γδ T cells and neutrophils, tumor cell lysis is increased compared to co-culture with γδ T cells alone, which can be attributed to elevated granzyme B and IFN-γ production. These conflicting observations may be explained by differences in immune cell subpopulation crosstalk within PBMCs, or by differing polarization of neutrophils: N1 neutrophils are tumor suppressive while N2 neutrophils have a pro-tumoral phenotype (280). It is worth noting that a higher neutrophil-to-lymphocyte ratio in a cohort study of 1,714 cancer patients treated with immune checkpoint inhibitors was recently reported to significantly correlate with low progression-free survival, poor response rates, and low clinical benefit (281). Considering the immunosuppressive functions of activated neutrophils on γδ T-cell activation as discussed above, this may partly contribute to the poor outcomes in cancer patients with higher neutrophil-to-lymphocyte ratios.
Crosstalk between γδ T cells and dendritic cells
DCs are professional APCs, and consist of classical or conventional DCs (cDCs), including cDC1 (CD11c+ and CD141+) and cDC2 (CD11c+ and CD1c+), and plasmacytoid DCs (pDCs, CD11c-, CD123+, and CD303+) (282, 283). Their key role in anti-tumor immunity is well described, but the interactions between DCs and γδ T cells is lacking behind. It has been shown that upon recognition of bacteria-infected or tumor cells, activated Vγ9Vδ2+ T cells can aid DC maturation through cytokine secretion (IFN-γ and TNF-α) (87, 88), and promote maturation of antigen-expressing immature DCs (monocyte-derived) in circulation via contact-dependent mechanisms (Fas/FasL, CD40/CD40L, and TCR/CD1) independent from TLR signaling (89–91, 93) (Figure 2). These Vγ9Vδ2+ T cell-matured DCs upregulate HLA-DR, CD25, CD40, CD80, CD83, and CD86, and are capable of cytokine production (TNF-α, IL-12, and IL-15, but not IL-10), antigen presentation, and stimulation of naïve CD4+ αβ T cells (76, 87, 89, 92, 284–288). In addition, Vγ9Vδ2+ T cell-derived cytokines (IFN-γ and TNF-α) can also enhance TLR-dependent DC maturation, upregulate CCR7 (lymph node-homing receptor), and facilitate their migration to lymphoid tissues for CD4+ αβ T-cell priming (289, 290).
In contrast, the tumor-derived chemokine ligand CXCL10 can promote the expansion of Vδ1+ γδ Treg cells that infiltrate solid tumors and induce immune senescence in DCs, and prevent DC maturation (by inhibiting CD80, CD83, CD86, and HLA-DR expression), DC function (decreased IL-6 and IL-12 production), and DC phenotype (inability to stimulate naïve T-cell proliferation) via the TLR8 signaling pathway or by killing of DCs through a perforin-mediated pathway (102–107) (Figure 2).
In turn, DCs can mediate Vγ9Vδ2+ T-cell activation by sensing/presenting HMBPP and induce γδ T-cell proliferation in the presence of IL-2, IL-15, and IL-21 (76, 94–96). Immature DCs can enhance the ability of Vγ9Vδ2+ T cells to secrete inflammatory cytokines necessary for γδ T-cell maturation (TNF-α) in part due to the ability of DCs to upregulate and/or sense phosphoantigens (88). Mature cDCs and pDCs (monocyte-derived) can secrete cytokines (IL-1β, IL-12, IL-18, IFN-γ, and TNF-α) that activate Vγ9Vδ2+ T cells, enhancing their proliferation and cytotoxic function (IL-18-mediated cytotoxicity against tumor cells) (287, 291–296). In the presence of phosphoantigen, IL-15-producing DCs (monocyte-derived) can also activate γδ T cells in a contact-dependent manner (CD86) and induce secretion of IFN-γ (284, 297, 298). Zoledronate-treated immature and mature DCs (monocyte-derived) can induce phosphoantigen-mediated activation and expansion of effector Vγ9Vδ2+ T cells capable of co-stimulatory and cytotoxic functions via the expression of CD40L (299–303).
In summary, different γδ T-cell subsets can either aid and promote or inhibit DC maturation and function (7, 13, 304, 305), while DCs can activate and expand Vγ9Vδ2+ T cells (7, 13, 304–307). The crosstalk between γδ T cells and DCs can thus have downstream anti- or pro-tumoral effects with therapeutic potential, albeit warranting further investigation using DCs that are not monocyte-derived (8, 150, 308).
Outlook and future perspective
Our understanding on γδ T cells continues to expand and their contributions in bridging the innate and adaptive anti-tumor immune responses are becoming more evident. Multiple studies are now highlighting their role in interacting with and orchestrating a variety of other immune cell subsets as reviewed here. Traditionally, γδ T cell-based cancer immunotherapies have been focused on assessing the efficacy of activated γδ T cells alone in mediating tumor clearance (41–46, 145, 157, 163, 165, 309). Although these past clinical trials have shown that γδ T cell-based immunotherapies were safe and well tolerated in patients, given the limited success to date (8, 10, 19, 101, 310–312), more innovative strategies aiming to overcome the challenges and immunosuppression within the TME should be thoroughly explored. Notably, with the ever-increasing numbers of studies demonstrating the intricate network of immune interactions within the TME, it is high time to deeply explore some of these interactions and to gain valuable insights into the unique immunomodulatory functions of γδ T cells in the context of cancer immunotherapy. Such acquired knowledge can be fully harnessed to develop a multipronged γδ T cell-based immunotherapy focusing on γδ T cells’ capability to influence the activities of other tumor-infiltrating immune cells via rapid cytokine and chemokine secretion, expression of various co-stimulatory molecules, and the professional APC functions in cross-priming and presenting antigens to αβ T cells.
For example, we are now armed with several potent therapeutic agents including the agonist antibodies against BTN3A1 (clone 20.1, CTX-2026, and ICT-01) and BTN2A1 (ICT-0302) that are capable of activating and enhancing the immunomodulatory functions of Vγ9Vδ2+ T cells (48–53, 227, 313, 314). Treatment targeting BTN3A1 (CTX-2026) can induce coordinated Vγ9Vδ2+ and αβ T-cell responses for tumor cell killing and represents a promising therapeutic approach that could be combined with other immune checkpoint inhibitors targeting PD-1/PD-L1 (nivolumab and pembrolizumab), CTLA-4/CD86 (ipilimumab and tremelimumab), KIRs (lirilumab and IPH4102), ILT-2 (anti-ILT-2, anti-HLA-G1, anti-FasL), and NKG2A (monalizumab) to circumvent potential immunosuppression in TME (11, 204, 205). These anti-tumor responses could potentially be further enhanced by inducing the expression of NKG2D ligands in tumor cells using a proteasome inhibitor (bortezomib) and an alkylating agent (temozolomide) to promote orchestrated NKG2D-mediated tumor cell lysis by tumor-infiltrating NK, CD8+ αβ, and γδ T cells (240, 256, 257). Moreover, CD137 (4-1BB) co-stimulation with recombinant human CD137L can boost the therapeutic effect of Vγ9Vδ2+ T cell-based immunotherapy and lead to heightened NK cell-mediated cytotoxicity (73, 75, 101, 315). Taken together, such combined therapeutic treatment will be a powerful approach to elicit concerted anti-tumor responses in different tumor-infiltrating immune cells and help to maximize the efficacy of future γδ T cell-based immunotherapy treatments in cancer patients.
Author Contributions
KFC, JDGD, SO, and AB wrote and prepared the manuscript draft. KFC prepared the figures. All authors contributed to the article and approved the submitted version.
Funding
AB is supported by a fellowship from the DHHS acting through the Victorian Cancer Agency. KFC is awarded the ECRs and MCRs Awards (ABC scheme 2022-2023) funding from La Trobe University (WBS: 3.2515.01.20). JDGD was supported by Cure Cancer Australia through the Cancer Australia Priority—Driven Cancer Research Scheme (#1187815). The contents of the published material are solely the responsibility of La Trobe University and do not reflect the views of Cancer Australia.
Conflict of Interest
AB declares research funding from CSL Ltd. AB and SO are inventors on a patent about mechanisms to activate γδ T cells.
The remaining authors declare that the research was conducted in the absence of any commercial or financial relationships that could be construed as a potential conflict of interest.
Publisher’s Note
All claims expressed in this article are solely those of the authors and do not necessarily represent those of their affiliated organizations, or those of the publisher, the editors and the reviewers. Any product that may be evaluated in this article, or claim that may be made by its manufacturer, is not guaranteed or endorsed by the publisher.
Acknowledgments
In loving memory of the departed ones, author KFC would like to dedicate this article to commemorate his beloved uncle, Liew Yau Kong and uncle’s son, Liew Sam Weng who passed away from COVID-19 in July 2021. Figures 1, 2 are created with BioRender.com.
References
1. Chien Y-H, Becker DM, Lindsten T, Okamura M, Cohen DI, Davis MM. A Third Type of Murine T-Cell Receptor Gene. Nature (1984) 312(5989):31–5. doi: 10.1038/312031a0
2. Hayday AC, Saito H, Gillies SD, Kranz DM, Tanigawa G, Eisen HN, et al. Structure, Organization, and Somatic Rearrangement of T Cell Gamma Genes. Cell (1985) 40(2):259–69. doi: 10.1016/0092-8674(85)90140-0
3. Rigau M, Uldrich AP, Behren A. Targeting Butyrophilins for Cancer Immunotherapy. Trends Immunol (2021) 42(8):670–80. doi: 10.1016/j.it.2021.06.002
4. Gentles AJ, Newman AM, Liu CL, Bratman SV, Feng W, Kim D, et al. The Prognostic Landscape of Genes and Infiltrating Immune Cells Across Human Cancers. Nat Med (2015) 21(8):938–45. doi: 10.1038/nm.3909
5. Zou C, Zhao P, Xiao Z, Han X, Fu F, Fu L. γδ T Cells in Cancer Immunotherapy. Oncotarget (2017) 8(5):8900. doi: 10.18632/oncotarget.13051
6. Godfrey DI, Le Nours J, Andrews DM, Uldrich AP, Rossjohn J. Unconventional T Cell Targets for Cancer Immunotherapy. Immunity (2018) 48(3):453–73. doi: 10.1016/j.immuni.2018.03.009
7. Zhao Y, Niu C, Cui J. Gamma-Delta (γδ) T Cells: Friend or Foe in Cancer Development? J Trans Med (2018) 16(1):1–13. doi: 10.1186/s12967-017-1378-2
8. Silva-Santos B, Mensurado S, Coffelt SB. γδ T Cells: Pleiotropic Immune Effectors With Therapeutic Potential in Cancer. Nat Rev Cancer (2019) 19(7):392–404. doi: 10.1038/s41568-019-0153-5
9. Hayday AC. γδ T Cell Update: Adaptate Orchestrators of Immune Surveillance. J Immunol (2019) 203(2):311–20. doi: 10.4049/jimmunol.1800934
10. Garber K. γδ T Cells Bring Unconventional Cancer-Targeting to the Clinic - Again. Nat Biotechnol (2020) 38(4):389–92. doi: 10.1038/s41587-020-0487-2
11. Kabelitz D, Serrano R, Kouakanou L, Peters C, Kalyan S. Cancer Immunotherapy With γδ T Cells: Many Paths Ahead of Us. Cell Mol Immunol (2020) 17(9):925–39. doi: 10.1038/s41423-020-0504-x
12. Lee HW, Chung YS, Kim TJ. Heterogeneity of Human γδ T Cells and Their Role in Cancer Immunity. Immune Network (2020) 20(1):e5. doi: 10.4110/in.2020.20.e5
13. Liu Y, Zhang C. The Role of Human γδ T Cells in Anti-Tumor Immunity and Their Potential for Cancer Immunotherapy. Cells (2020) 9(5):1206. doi: 10.3390/cells9051206
14. Morandi F, Yazdanifar M, Cocco C, Bertaina A, Airoldi I. Engineering the Bridge Between Innate and Adaptive Immunity for Cancer Immunotherapy: Focus on γδ T and NK Cells. Cells (2020) 9(8):1757. doi: 10.3390/cells9081757
15. Park JH, Lee HK. Function of γδ T Cells in Tumor Immunology and Their Application to Cancer Therapy. Exp Mol Med (2021) 53(3):318–27. doi: 10.1038/s12276-021-00576-0
16. Ribot JC, Lopes N, Silva-Santos B. γδ T Cells in Tissue Physiology and Surveillance. Nat Rev Immunol (2021) 21(4):221–32. doi: 10.1038/s41577-020-00452-4
17. Chien Y-H, Meyer C, Bonneville M. γδ T Cells: First Line of Defense and Beyond. Annu Rev Immunol (2014) 32:121–55. doi: 10.1146/annurev-immunol-032713-120216
18. Godfrey DI, Uldrich AP, McCluskey J, Rossjohn J, Moody DB. The Burgeoning Family of Unconventional T Cells. Nat Immunol (2015) 16(11):1114–23. doi: 10.1038/ni.3298
19. Sebestyen Z, Prinz I, Déchanet-Merville J, Silva-Santos B, Kuball J. Translating Gammadelta (γδ) T Cells and Their Receptors Into Cancer Cell Therapies. Nat Rev Drug Discov (2020) 19(3):169–84. doi: 10.1038/s41573-019-0038-z
20. Kabelitz D, Kalyan S, Oberg H-H, Wesch D. Human Vδ2 Versus non-Vδ2 γδ T Cells in Antitumor Immunity. Oncoimmunology (2013) 2(3):e23304. doi: 10.4161/onci.23304
21. Adams EJ, Gu S, Luoma AM. Human Gamma Delta T Cells: Evolution and Ligand Recognition. Cell Immunol (2015) 296(1):31–40. doi: 10.1016/j.cellimm.2015.04.008
22. Qi C, Wang Y, Li P, Zhao J. Gamma Delta T Cells and Their Pathogenic Role in Psoriasis. Front Immunol (2021) 12. doi: 10.3389/fimmu.2021.627139
23. Peigné C-M, Léger A, Gesnel M-C, Konczak F, Olive D, Bonneville M, et al. The Juxtamembrane Domain of Butyrophilin BTN3A1 Controls Phosphoantigen-Mediated Activation of Human Vγ9vδ2 T Cells. J Immunol (2017) 198(11):4228–34. doi: 10.4049/jimmunol.1601910
24. Wang H, Nada MH, Tanaka Y, Sakuraba S, Morita CT. Critical Roles for Coiled-Coil Dimers of Butyrophilin 3A1 in the Sensing of Prenyl Pyrophosphates by Human Vγ2vδ2 T Cells. J Immunol (2019) 203(3):607–26. doi: 10.4049/jimmunol.1801252
25. Tanaka Y, Morita CT, Tanaka Y, Nieves E, Brenner MB, Bloom BR. Natural and Synthetic non-Peptide Antigens Recognized by Human γδ T Cells. Nature (1995) 375(6527):155–8. doi: 10.1038/375155a0
26. Hintz M, Reichenberg A, Altincicek B, Bahr U, Gschwind RM, Kollas A-K, et al. Identification of (E)-4-Hydroxy-3-Methyl-But-2-Enyl Pyrophosphate as a Major Activator for Human γδ T Cells in Escherichia Coli. FEBS Lett (2001) 509(2):317–22. doi: 10.1016/S0014-5793(01)03191-X
27. Vavassori S, Kumar A, Wan GS, Ramanjaneyulu GS, Cavallari M, El Daker S, et al. Butyrophilin 3A1 Binds Phosphorylated Antigens and Stimulates Human γδ T Cells. Nat Immunol (2013) 14(9):908–16. doi: 10.1038/ni.2665
28. Sandstrom A, Peigné C-M, Léger A, Crooks JE, Konczak F, Gesnel M-C, et al. The Intracellular B30. 2 Domain of Butyrophilin 3A1 Binds Phosphoantigens to Mediate Activation of Human Vγ9vδ2 T Cells. Immunity (2014) 40(4):490–500. doi: 10.1016/j.immuni.2014.03.003
29. Gu S, Sachleben JR, Boughter CT, Nawrocka WI, Borowska MT, Tarrasch JT, et al. Phosphoantigen-Induced Conformational Change of Butyrophilin 3A1 (BTN3A1) and its Implication on Vγ9vδ2 T Cell Activation. Proc Natl Acad Sci (2017) 114(35):E7311–E20. doi: 10.1073/pnas.1707547114
30. Yang Y, Li L, Yuan L, Zhou X, Duan J, Xiao H, et al. A Structural Change in Butyrophilin Upon Phosphoantigen Binding Underlies Phosphoantigen-Mediated Vγ9vδ2 T Cell Activation. Immunity (2019) 50(4):1043–53.e5. doi: 10.1016/j.immuni.2019.02.016
31. Hsiao C-HC, Nguyen K, Jin Y, Vinogradova O, Wiemer AJ. Ligand-Induced Interactions Between Butyrophilin 2A1 and 3A1 Internal Domains in the HMBPP Receptor Complex. Cell Chem Biol (2022) 29(6):985–95.e5. doi: 10.1016/j.chembiol.2022.01.004
32. Behren A, Anaka M, Lo PH, Vella LJ, Davis ID, Catimel J, et al. The Ludwig Institute for Cancer Research Melbourne Melanoma Cell Line Panel. Pigment Cell Melanoma Res (2013) 26(4):597–600. doi: 10.1111/pcmr.12097
33. Rigau M, Ostrouska S, Fulford TS, Johnson DN, Woods K, Ruan Z, et al. Butyrophilin 2A1 is Essential for Phosphoantigen Reactivity by γδ T Cells. Science (2020) 367(6478):eaay5516. doi: 10.1126/science.aay5516
34. Karunakaran MM, Willcox CR, Salim M, Paletta D, Fichtner AS, Noll A, et al. Butyrophilin-2A1 Directly Binds Germline-Encoded Regions of the Vγ9vδ2 TCR and is Essential for Phosphoantigen Sensing. Immunity (2020) 52(3):487–98.e6. doi: 10.1016/j.immuni.2020.02.014
35. Vyborova A, Beringer DX, Fasci D, Karaiskaki F, van Diest E, Kramer L, et al. γ9δ2t Cell Diversity and the Receptor Interface With Tumor Cells. J Clin Invest (2020) 130(9):4637–51. doi: 10.1172/JCI132489
36. Cano CE, Pasero C, De Gassart A, Kerneur C, Gabriac M, Fullana M, et al. BTN2A1, an Immune Checkpoint Targeting Vγ9vδ2 T Cell Cytotoxicity Against Malignant Cells. Cell Rep (2021) 36(2):109359. doi: 10.1016/j.celrep.2021.109359
37. Gober H-J, Kistowska M, Angman L, Jenoü P, Mori L, De Libero G. Human T Cell Receptor γδ Cells Recognize Endogenous Mevalonate Metabolites in Tumor Cells. J Exp Med (2003) 197(2):163–8. doi: 10.1084/jem.20021500
38. Benzaïd I, Mönkkönen H, Stresing V, Bonnelye E, Green J, Mönkkönen J, et al. High Phosphoantigen Levels in Bisphosphonate-Treated Human Breast Tumors Promote Vγ9vδ2 T-Cell Chemotaxis and Cytotoxicity In Vivo. Cancer Res (2011) 71(13):4562–72. doi: 10.1158/0008-5472.CAN-10-3862
39. Ashihara E, Munaka T, Kimura S, Nakagawa S, Nakagawa Y, Kanai M, et al. Isopentenyl Pyrophosphate Secreted From Zoledronate-Stimulated Myeloma Cells, Activates the Chemotaxis of γδt Cells. Biochem Biophys Res Commun (2015) 463(4):650–5. doi: 10.1016/j.bbrc.2015.05.118
40. Kunzmann V, Bauer E, Feurle J, Weissinger F, Tony HP, Wilhelm M. Stimulation of γδ T Cells by Aminobisphosphonates and Induction of Antiplasma Cell Activity in Multiple Myeloma. Blood J Am Soc Hematol (2000) 96(2):384–92. doi: 10.1182/blood.V96.2.384.013k07_384_392
41. Viey E, Fromont G, Escudier B, Morel Y, Da Rocha S, Chouaib S, et al. Phosphostim-Activated γδ T Cells Kill Autologous Metastatic Renal Cell Carcinoma. J Immunol (2005) 174(3):1338–47. doi: 10.4049/jimmunol.174.3.1338
42. Mattarollo SR, Kenna T, Nieda M, Nicol AJ. Chemotherapy and Zoledronate Sensitize Solid Tumour Cells to Vγ9vδ2 T Cell Cytotoxicity. Cancer Immunol Immunother (2007) 56(8):1285–97. doi: 10.1007/s00262-007-0279-2
43. Bouet-Toussaint F, Cabillic F, Toutirais O, Le Gallo M, de la Pintière CT, Daniel P, et al. Vγ9vδ2 T Cell-Mediated Recognition of Human Solid Tumors. Potential for Immunotherapy of Hepatocellular and Colorectal Carcinomas. Cancer Immunol Immunother (2008) 57(4):531–9. doi: 10.1007/s00262-007-0391-3
44. Gertner-Dardenne J, Castellano R, Mamessier E, Garbit S, Kochbati E, Etienne A, et al. Human Vγ9vδ2 T Cells Specifically Recognize and Kill Acute Myeloid Leukemic Blasts. J Immunol (2012) 188(9):4701–8. doi: 10.4049/jimmunol.1103710
45. Abe Y, Muto M, Nieda M, Nakagawa Y, Nicol A, Kaneko T, et al. Clinical and Immunological Evaluation of Zoledronate-Activated Vγ9γδ T-Cell-Based Immunotherapy for Patients With Multiple Myeloma. Exp Hematol (2009) 37(8):956–68. doi: 10.1016/j.exphem.2009.04.008
46. Meraviglia S, Eberl M, Vermijlen D, Todaro M, Buccheri S, Cicero G, et al. In Vivo Manipulation of Vγ9vδ2 T Cells With Zoledronate and Low-Dose Interleukin-2 for Immunotherapy of Advanced Breast Cancer Patients. Clin Exp Immunol (2010) 161(2):290–7. doi: 10.1111/j.1365-2249.2010.04167.x
47. Yazdanifar M, Barbarito G, Bertaina A, Airoldi I. γδ T Cells: The Ideal Tool for Cancer Immunotherapy. Cells (2020) 9(5):1305. doi: 10.3390/cells9051305
48. Harly C, Guillaume Y, Nedellec S, Peigné C-M, Mönkkönen H, Mönkkönen J, et al. Key Implication of CD277/butyrophilin-3 (BTN3A) in Cellular Stress Sensing by a Major Human γδ T-Cell Subset. Blood J Am Soc Hematol (2012) 120(11):2269–79. doi: 10.1182/blood-2012-05-430470
49. Palakodeti A, Sandstrom A, Sundaresan L, Harly C, Nedellec S, Olive D, et al. The Molecular Basis for Modulation of Human Vγ9vδ2 T Cell Responses by CD277/butyrophilin-3 (BTN3A)-Specific Antibodies. J Biol Chem (2012) 287(39):32780–90. doi: 10.1074/jbc.M112.384354
50. Arnett HA, Viney JL. Immune Modulation by Butyrophilins. Nat Rev Immunol (2014) 14(8):559–69. doi: 10.1038/nri3715
51. Starick L, Riano F, Karunakaran MM, Kunzmann V, Li J, Kreiss M, et al. Butyrophilin 3a (BTN3A, CD277)-Specific Antibody 20.1 Differentially Activates Vγ9vδ2 TCR Clonotypes and Interferes With Phosphoantigen Activation. Eur J Immunol (2017) 47(6):982–92. doi: 10.1002/eji.201646818
52. Payne KK, Mine JA, Biswas S, Chaurio RA, Perales-Puchalt A, Anadon CM, et al. BTN3A1 Governs Antitumor Responses by Coordinating αβ and γδ T Cells. Science (2020) 369(6506):942–9. doi: 10.1126/science.aay2767
53. De Gassart A, Le K-S, Brune P, Agaugué S, Sims J, Goubard A, et al. Development of ICT01, a First-in-Class, Anti-BTN3A Antibody for Activating Vγ9vδ2 T Cell–Mediated Antitumor Immune Response. Sci Trans Med (2021) 13(616):eabj0835. doi: 10.1126/scitranslmed.abj0835
54. Wu D, Wu P, Qiu F, Wei Q, Huang J. Human γδt-Cell Subsets and Their Involvement in Tumor Immunity. Cell Mol Immunol (2017) 14(3):245–53. doi: 10.1038/cmi.2016.55
55. Willcox BE, Willcox CR. γδ TCR Ligands: The Quest to Solve a 500-Million-Year-Old Mystery. Nat Immunol (2019) 20(2):121–8. doi: 10.1038/s41590-018-0304-y
56. Brandes M, Willimann K, Moser B. Professional Antigen-Presentation Function by Human γδ T Cells. Science (2005) 309(5732):264–8. doi: 10.1126/science.1110267
57. Brandes M, Willimann K, Bioley G, Lévy N, Eberl M, Luo M, et al. Cross-Presenting Human γδ T Cells Induce Robust CD8+ αβ T Cell Responses. Proc Natl Acad Sci (2009) 106(7):2307–12. doi: 10.1073/pnas.0810059106
58. Meuter S, Eberl M, Moser B. Prolonged Antigen Survival and Cytosolic Export in Cross-Presenting Human γδ T Cells. Proc Natl Acad Sci (2010) 107(19):8730–5. doi: 10.1073/pnas.1002769107
59. Altvater B, Pscherer S, Landmeier S, Kailayangiri S, Savoldo B, Juergens H, et al. Activated Human γδ T Cells Induce Peptide-Specific CD8+ T-Cell Responses to Tumor-Associated Self-Antigens. Cancer Immunol Immunother (2012) 61(3):385–96. doi: 10.1007/s00262-011-1111-6
60. Himoudi N, Morgenstern DA, Yan M, Vernay B, Saraiva L, Wu Y, et al. Human γδ T Lymphocytes are Licensed for Professional Antigen Presentation by Interaction With Opsonized Target Cells. J Immunol (2012) 188(4):1708–16. doi: 10.4049/jimmunol.1102654
61. Howard J, Loizon S, Tyler CJ, Duluc D, Moser B, Mechain M, et al. The Antigen-Presenting Potential of Vγ9vδ2 T Cells During Plasmodium Falciparum Blood-Stage Infection. J Infect Dis (2017) 215(10):1569–79. doi: 10.1093/infdis/jix149
62. Barisa M, Kramer A, Majani Y, Moulding D, Saraiva L, Bajaj-Elliott M, et al. E. Coli Promotes Human Vγ9vδ2 T Cell Transition From Cytokine-Producing Bactericidal Effectors to Professional Phagocytic Killers in a TCR-Dependent Manner. Sci Rep (2017) 7(1):1–12. doi: 10.1038/s41598-017-02886-8
63. Tyler CJ, McCarthy NE, Lindsay JO, Stagg AJ, Moser B, Eberl M. Antigen-Presenting Human γδ T Cells Promote Intestinal CD4+ T Cell Expression of IL-22 and Mucosal Release of Calprotectin. J Immunol (2017) 198(9):3417–25. doi: 10.4049/jimmunol.1700003
64. Groh V, Porcelli S, Fabbi M, Lanier LL, Picker LJ, Anderson T, et al. Human Lymphocytes Bearing T Cell Receptor Gamma/Delta are Phenotypically Diverse and Evenly Distributed Throughout the Lymphoid System. J Exp Med (1989) 169(4):1277–94. doi: 10.1084/jem.169.4.1277
65. Gascan H, Gauchat J-F, Roncarolo M-G, Yssel H, Spits H, De Vries JE. Human B Cell Clones can be Induced to Proliferate and to Switch to IgE and IgG4 Synthesis by Interleukin 4 and a Signal Provided by Activated CD4+ T Cell Clones. J Exp Med (1991) 173(3):747–50. doi: 10.1084/jem.173.3.747
66. Horner AA, Jabara H, Ramesh N, Geha RS. γ/δ T Lymphocytes Express CD40 Ligand and Induce Isotype Switching in B Lymphocytes. J Exp Med (1995) 181(3):1239–44. doi: 10.1084/jem.181.3.1239
67. Nelms K, Keegan AD, Zamorano J, Ryan JJ, Paul WE. The IL-4 Receptor: Signaling Mechanisms and Biologic Functions. Annu Rev Immunol (1999) 17(1):701–38. doi: 10.1146/annurev.immunol.17.1.701
68. Brandes M, Willimann K, Lang AB, Nam K-H, Jin C, Brenner MB, et al. Flexible Migration Program Regulates γδ T-Cell Involvement in Humoral Immunity. Blood (2003) 102(10):3693–701. doi: 10.1182/blood-2003-04-1016
69. Caccamo N, Battistini L, Bonneville M, Poccia F, Fournié JJ, Meraviglia S, et al. CXCR5 Identifies a Subset of Vγ9vδ2 T Cells Which Secrete IL-4 and IL-10 and Help B Cells for Antibody Production. J Immunol (2006) 177(8):5290–5. doi: 10.4049/jimmunol.177.8.5290
70. Vermijlen D, Ellis P, Langford C, Klein A, Engel R, Willimann K, et al. Distinct Cytokine-Driven Responses of Activated Blood γδ T Cells: Insights Into Unconventional T Cell Pleiotropy. J Immunol (2007) 178(7):4304–14. doi: 10.4049/jimmunol.178.7.4304
71. Bansal RR, Mackay CR, Moser B, Eberl M. IL-21 Enhances the Potential of Human γδ T Cells to Provide B-Cell Help. Eur J Immunol (2012) 42(1):110–9. doi: 10.1002/eji.201142017
72. Petrasca A, Doherty DG. Human Vδ2+ γδ T Cells Differentially Induce Maturation, Cytokine Production and Alloreactive T Cell Stimulation by Dendritic Cells and B Cells. Front Immunol (2014) 5:650. doi: 10.3389/fimmu.2014.00650
73. Maniar A, Zhang X, Lin W, Gastman BR, Pauza CD, Strome SE, et al. Human γδ T Lymphocytes Induce Robust NK Cell–Mediated Antitumor Cytotoxicity Through CD137 Engagement. Blood J Am Soc Hematol (2010) 116(10):1726–33. doi: 10.1182/blood-2009-07-234211
74. Nussbaumer O, Gruenbacher G, Gander H, Thurnher M. DC-Like Cell-Dependent Activation of Human Natural Killer Cells by the Bisphosphonate Zoledronic Acid is Regulated by γδ T Lymphocytes. Blood J Am Soc Hematol (2011) 118(10):2743–51. doi: 10.1182/blood-2011-01-328526
75. Liu M, Hu Y, Yuan Y, Tian Z, Zhang C. γδt Cells Suppress Liver Fibrosis via Strong Cytolysis and Enhanced NK Cell-Mediated Cytotoxicity Against Hepatic Stellate Cells. Front Immunol (2019) 10:477. doi: 10.3389/fimmu.2019.00477
76. Eberl M, Roberts GW, Meuter S, Williams JD, Topley N, Moser B. A Rapid Crosstalk of Human γδ T Cells and Monocytes Drives the Acute Inflammation in Bacterial Infections. PloS Pathogens (2009) 5(2):e1000308. doi: 10.1371/journal.ppat.1000308
77. Kalyan S, Chow AW. Linking Innate and Adaptive Immunity: Human V 9v 2 T Cells Enhance CD40 Expression and HMGB-1 Secretion. Mediators Inflamm (2009) 2009. doi: 10.1155/2009/819408
78. Davey MS, Lin C-Y, Roberts GW, Heuston S, Brown AC, Chess JA, et al. Human Neutrophil Clearance of Bacterial Pathogens Triggers Anti-Microbial γδ T Cell Responses in Early Infection. PloS Pathogens (2011) 7(5):e1002040. doi: 10.1371/journal.ppat.1002040
79. Dieli F, Troye-Blomberg M, Ivanyi J, Fournié JJ, Bonneville M, Peyrat MA, et al. Vγ9/Vδ2 T Lymphocytes Reduce the Viability of Intracellular Mycobacterium Tuberculosis. Eur J Immunol (2000) 30(5):1512–9. doi: 10.1002/(SICI)1521-4141(200005)30:5<1512::AID-IMMU1512>3.0.CO;2-3
80. Ferrero E, Biswas P, Vettoretto K, Ferrarini M, Uguccioni M, Piali L, et al. Macrophages Exposed to Mycobacterium Tuberculosis Release Chemokines Able to Recruit Selected Leucocyte Subpopulations: Focus on γδ Cells. Immunology (2003) 108(3):365–74. doi: 10.1046/j.1365-2567.2003.01600.x
81. Qin G, Mao H, Zheng J, Sia SF, Liu Y, Chan P-L, et al. Phosphoantigen-Expanded Human γδ T Cells Display Potent Cytotoxicity Against Monocyte-Derived Macrophages Infected With Human and Avian Influenza Viruses. J Infect Dis (2009) 200(6):858–65. doi: 10.1086/605413
82. Spencer CT, Abate G, Sakala IG, Xia M, Truscott SM, Eickhoff CS, et al. Granzyme A Produced by γ9δ2 T Cells Induces Human Macrophages to Inhibit Growth of an Intracellular Pathogen. PloS Pathogens (2013) 9(1):e1003119. doi: 10.1371/journal.ppat.1003119
83. Agrati C, Cimini E, Sacchi A, Bordoni V, Gioia C, Casetti R, et al. Activated Vγ9vδ2 T Cells Trigger Granulocyte Functions via MCP-2 Release. J Immunol (2009) 182(1):522–9. doi: 10.4049/jimmunol.182.1.522
84. Fazio J, Kalyan S, Wesch D, Kabelitz D. Inhibition of Human γδ T Cell Proliferation and Effector Functions by Neutrophil Serine Proteases. Scand J Immunol (2014) 80(6):381–9. doi: 10.1111/sji.12221
85. Kalyan S, Chandrasekaran V, Quabius ES, Lindhorst TK, Kabelitz D. Neutrophil Uptake of Nitrogen-Bisphosphonates Leads to the Suppression of Human Peripheral Blood γδ T Cells. Cell Mol Life Sci (2014) 71(12):2335–46. doi: 10.1007/s00018-013-1495-x
86. Sabbione F, Gabelloni ML, Ernst G, Gori MS, Salamone G, Oleastro M, et al. Neutrophils Suppress γδ T-Cell Function. Eur J Immunol (2014) 44(3):819–30. doi: 10.1002/eji.201343664
87. Ismaili J, Olislagers V, Poupot R, Fournié J-J, Goldman M. Human γδ T Cells Induce Dendritic Cell Maturation. Clin Immunol (2002) 103(3):296–302. doi: 10.1006/clim.2002.5218
88. Devilder M-C, Maillet S, Bouyge-Moreau I, Donnadieu E, Bonneville M, Scotet E. Potentiation of Antigen-Stimulated Vγ9vδ2 T Cell Cytokine Production by Immature Dendritic Cells (DC) and Reciprocal Effect on DC Maturation. J Immunol (2006) 176(3):1386–93. doi: 10.4049/jimmunol.176.3.1386
89. Leslie DS, Vincent MS, Spada FM, Das H, Sugita M, Morita CT, et al. CD1-Mediated γ/δ T Cell Maturation of Dendritic Cells. J Exp Med (2002) 196(12):1575–84. doi: 10.1084/jem.20021515
90. Eberl M, Jomaa H, Hayday AC. Integrated Immune Responses to Infection–Cross-Talk Between Human γδ T Cells and Dendritic Cells. Immunology (2004) 112(3):364. doi: 10.1111/j.1365-2567.2004.01921.x
91. Münz C, Steinman RM, Fujii S-I. Dendritic Cell Maturation by Innate Lymphocytes: Coordinated Stimulation of Innate and Adaptive Immunity. J Exp Med (2005) 202(2):203–7. doi: 10.1084/jem.20050810
92. Dunne MR, Madrigal-Estebas L, Tobin LM, Doherty DG. (E)-4-Hydroxy-3-Methyl-But-2 Enyl Pyrophosphate-Stimulated Vγ9vδ2 T Cells Possess T Helper Type 1-Promoting Adjuvant Activity for Human Monocyte-Derived Dendritic Cells. Cancer Immunol Immunother (2010) 59(7):1109–20. doi: 10.1007/s00262-010-0839-8
93. van Beek JJ, Wimmers F, Hato SV, de Vries IJM, Skold AE. Dendritic Cell Cross Talk With Innate and Innate-Like Effector Cells in Antitumor Immunity: Implications for DC Vaccination. Crit Reviews™ Immunol (2014) 34(6):517–36. doi: 10.1615/CritRevImmunol.2014012204
94. Pechhold K, Wesch D, Schondelmaier S, Kabelitz D. Primary Activation of V Gamma 9-Expressing Gamma Delta T Cells by Mycobacterium Tuberculosis. Requirement for Th1-Type CD4 T Cell Help and Inhibition by IL-10. J Immunol (1994) 152(10):4984–92.
95. Boullier S, Poquet Y, Debord T, Fournie JJ, Gougeon ML. Regulation by Cytokines (IL-12, IL-15, IL-4 and IL-10) of the Vγ9vδ2 T Cell Response to Mycobacterial Phosphoantigens in Responder and Anergic HIV-Infected Persons. Eur J Immunol (1999) 29(1):90–9. doi: 10.1002/(SICI)1521-4141(199901)29:01<90::AID-IMMU90>3.0.CO;2-1
96. Eberl M, Altincicek B, Kollas AK, Sanderbrand S, Bahr U, Reichenberg A, et al. Accumulation of a Potent γδ T-Cell Stimulator After Deletion of the lytB Gene in Escherichia Coli. Immunology (2002) 106(2):200–11. doi: 10.1046/j.1365-2567.2002.01414.x
97. Zhu Y, Wang H, Xu Y, Hu Y, Chen H, Cui L, et al. Human γδ T Cells Augment Antigen Presentation in Listeria Monocytogenes Infection. Mol Med (2016) 22(1):737–46. doi: 10.2119/molmed.2015.00214
98. Wu Y, Wu W, Wong WM, Ward E, Thrasher AJ, Goldblatt D, et al. Human γδ T Cells: A Lymphoid Lineage Cell Capable of Professional Phagocytosis. J Immunol (2009) 183(9):5622–9. doi: 10.4049/jimmunol.0901772
99. Mao C, Mou X, Zhou Y, Yuan G, Xu C, Liu H, et al. Tumor-Activated T Cells From Gastric Cancer Patients Induce the Antitumor Immune Response of T Cells via Their Antigen-Presenting Cell-Like Effects. J Immunol Res (2014) 2014. doi: 10.1155/2014/593562
100. Cairo C, Surendran N, Harris KM, Mazan-Mamczarz K, Sakoda Y, Diaz-Mendez F, et al. Vγ2vδ2 T-Cell Co-Stimulation Increases Natural Killer Cell Killing of Monocyte-Derived Dendritic Cells. Immunology (2015) 144(3):422–30. doi: 10.1111/imm.12386
101. Pauza CD, Liou M-L, Lahusen T, Xiao L, Lapidus RG, Cairo C, et al. Gamma Delta T Cell Therapy for Cancer: It is Good to be Local. Front Immunol (2018) 9:1305. doi: 10.3389/fimmu.2018.01305
102. Peng G, Wang HY, Peng W, Kiniwa Y, Seo KH, Wang R-F. Tumor-Infiltrating γδ T Cells Suppress T and Dendritic Cell Function via Mechanisms Controlled by a Unique Toll-Like Receptor Signaling Pathway. Immunity (2007) 27(2):334–48. doi: 10.1016/j.immuni.2007.05.020
103. Chen Y, Lin P, Qiu S, Peng X-X, Looi K, Farquhar MG, et al. Autoantibodies to Ca2+ Binding Protein Calnuc is a Potential Marker in Colon Cancer Detection. Int J Oncol (2007) 30(5):1137–44. doi: 10.3892/ijo.30.5.1137
104. Merims S, Dokouhaki P, Joe B, Zhang L. Human Vδ1-T Cells Regulate Immune Responses by Targeting Autologous Immature Dendritic Cells. Hum Immunol (2011) 72(1):32–6. doi: 10.1016/j.humimm.2010.10.011
105. Ma C, Zhang Q, Ye J, Wang F, Zhang Y, Wevers E, et al. Tumor-Infiltrating γδ T Lymphocytes Predict Clinical Outcome in Human Breast Cancer. J Immunol (2012) 189(10):5029–36. doi: 10.4049/jimmunol.1201892
106. Ye J, Ma C, Hsueh EC, Eickhoff CS, Zhang Y, Varvares MA, et al. Tumor-Derived γδ Regulatory T Cells Suppress Innate and Adaptive Immunity Through the Induction of Immunosenescence. J Immunol (2013) 190(5):2403–14. doi: 10.4049/jimmunol.1202369
107. Ye J, Ma C, Wang F, Hsueh EC, Toth K, Huang Y, et al. Specific Recruitment of γδ Regulatory T Cells in Human Breast Cancer. Cancer Res (2013) 73(20):6137–48. doi: 10.1158/0008-5472.CAN-13-0348
108. Muto M, Baghdadi M, Maekawa R, Wada H, Seino K. Myeloid Molecular Characteristics of Human γδ T Cells Support Their Acquisition of Tumor Antigen-Presenting Capacity. Cancer Immunol Immunother (2015) 64(8):941–9. doi: 10.1007/s00262-015-1700-x
109. Mangan BA, Dunne MR, O’Reilly VP, Dunne PJ, Exley MA, O’Shea D, et al. Cutting Edge: CD1d Restriction and Th1/Th2/Th17 Cytokine Secretion by Human Vδ3 T Cells. J Immunol (2013) 191(1):30–4. doi: 10.4049/jimmunol.1300121
110. Roy S, Ly D, Castro CD, Li NS, Hawk AJ, Altman JD, et al. Molecular Analysis of Lipid-Reactive Vdelta1 Gammadelta T Cells Identified by CD1c Tetramers. J Immunol (2016) 196(4):1933–42. doi: 10.4049/jimmunol.1502202
111. Uldrich AP, Le Nours J, Pellicci DG, Gherardin NA, McPherson KG, Lim RT, et al. CD1d-Lipid Antigen Recognition by the γδ TCR. Nat Immunol (2013) 14(11):1137–45. doi: 10.1038/ni.2713
112. Bai L, Picard D, Anderson B, Chaudhary V, Luoma A, Jabri B, et al. The Majority of CD1d-Sulfatide-Specific T Cells in Human Blood Use a Semiinvariant Vδ1 TCR. Eur J Immunol (2012) 42(9):2505–10. doi: 10.1002/eji.201242531
113. Luoma AM, Castro CD, Mayassi T, Bembinster LA, Bai L, Picard D, et al. Crystal Structure of Vδ1 T Cell Receptor in Complex With CD1d-Sulfatide Shows MHC-Like Recognition of a Self-Lipid by Human γδ T Cells. Immunity (2013) 39(6):1032–42. doi: 10.1016/j.immuni.2013.11.001
114. Zeng X, Wei Y-L, Huang J, Newell EW, Yu H, Kidd BA, et al. γδ T Cells Recognize a Microbial Encoded B Cell Antigen to Initiate a Rapid Antigen-Specific Interleukin-17 Response. Immunity (2012) 37(3):524–34. doi: 10.1016/j.immuni.2012.06.011
115. Silva-Santos B, Schamel WW, Fisch P, Eberl M. Gamma-Delta T-Cell Conference 2012: Close Encounters for the Fifth Time. Eur J Immunol (2012) 42(12):3101–5. doi: 10.1002/eji.201270101
116. Le Nours J, Gherardin NA, Ramarathinam SH, Awad W, Wiede F, Gully BS, et al. A Class of γδ T Cell Receptors Recognize the Underside of the Antigen-Presenting Molecule MR1. Science (2019) 366(6472):1522–7. doi: 10.1126/science.aav3900
117. Godder K, Henslee-Downey P, Mehta J, Park B, Chiang K, Abhyankar S, et al. Long Term Disease-Free Survival in Acute Leukemia Patients Recovering With Increased γδ T Cells After Partially Mismatched Related Donor Bone Marrow Transplantation. Bone Marrow Transplant (2007) 39(12):751–7. doi: 10.1038/sj.bmt.1705650
118. Siegers GM, Dhamko H, Wang X-H, Mathieson AM, Kosaka Y, Felizardo TC, et al. Human Vδ1 γδ T Cells Expanded From Peripheral Blood Exhibit Specific Cytotoxicity Against B-Cell Chronic Lymphocytic Leukemia-Derived Cells. Cytotherapy (2011) 13(6):753–64. doi: 10.3109/14653249.2011.553595
119. Donia M, Ellebaek E, Andersen MH, Straten PT, Svane IM. Analysis of Vδ1 T Cells in Clinical Grade Melanoma-Infiltrating Lymphocytes. Oncoimmunology (2012) 1(8):1297–304. doi: 10.4161/onci.21659
120. Knight A, Mackinnon S, Lowdell MW. Human Vdelta1 Gamma-Delta T Cells Exert Potent Specific Cytotoxicity Against Primary Multiple Myeloma Cells. Cytotherapy (2012) 14(9):1110–8. doi: 10.3109/14653249.2012.700766
121. Scheper W, van Dorp S, Kersting S, Pietersma F, Lindemans C, Hol S, et al. γδt Cells Elicited by CMV Reactivation After Allo-SCT Cross-Recognize CMV and Leukemia. Leukemia (2013) 27(6):1328–38. doi: 10.1038/leu.2012.374
122. Di Lorenzo B, Simões AE, Caiado F, Tieppo P, Correia DV, Carvalho T, et al. Broad Cytotoxic Targeting of Acute Myeloid Leukemia by Polyclonal Delta One T Cells. Cancer Immunol Res (2019) 7(4):552–8. doi: 10.1158/2326-6066.CIR-18-0647
123. Mikulak J, Oriolo F, Bruni E, Roberto A, Colombo FS, Villa A, et al. NKp46-Expressing Human Gut-Resident Intraepithelial Vδ1 T Cell Subpopulation Exhibits High Antitumor Activity Against Colorectal Cancer. JCI Insight (2019) 4(24):e125884. doi: 10.1172/jci.insight.125884
124. Wu Y, Kyle-Cezar F, Woolf RT, Naceur-Lombardelli C, Owen J, Biswas D, et al. An Innate-Like Vδ1+ γδ T Cell Compartment in the Human Breast is Associated With Remission in Triple-Negative Breast Cancer. Sci Trans Med (2019) 11(513):eaax9364. doi: 10.1126/scitranslmed.aax9364
125. Bauer S, Groh V, Wu J, Steinle A, Phillips JH, Lanier LL, et al. Activation of NK Cells and T Cells by NKG2D, a Receptor for Stress-Inducible MICA. science (1999) 285(5428):727–9. doi: 10.1126/science.285.5428.727
126. Almeida AR, Correia DV, Fernandes-Platzgummer A, da Silva CL, da Silva MG, Anjos DR, et al. Delta One T Cells for Immunotherapy of Chronic Lymphocytic Leukemia: Clinical-Grade Expansion/Differentiation and Preclinical Proof of Concept. Clin Cancer Res (2016) 22(23):5795–804. doi: 10.1158/1078-0432.CCR-16-0597
127. Marlin R, Pappalardo A, Kaminski H, Willcox CR, Pitard V, Netzer S, et al. Sensing of Cell Stress by Human γδ TCR-Dependent Recognition of Annexin A2. Proc Natl Acad Sci (2017) 114(12):3163–8. doi: 10.1073/pnas.1621052114
128. Davey MS, Willcox CR, Baker AT, Hunter S, Willcox BE. Recasting Human Vδ1 Lymphocytes in an Adaptive Role. Trends Immunol (2018) 39(6):446–59. doi: 10.1016/j.it.2018.03.003
129. Bridgeman JS, Sewell AK, Miles JJ, Price DA, Cole DK. Structural and Biophysical Determinants of αβ T-Cell Antigen Recognition. Immunology (2012) 135(1):9–18. doi: 10.1111/j.1365-2567.2011.03515.x
130. Chan KF, Gully BS, Gras S, Beringer DX, Kjer-Nielsen L, Cebon J, et al. Divergent T-Cell Receptor Recognition Modes of a HLA-I Restricted Extended Tumour-Associated Peptide. Nat Commun (2018) 9(1):1026. doi: 10.1038/s41467-018-03321-w
131. Rossjohn J, Gras S, Miles JJ, Turner SJ, Godfrey DI, McCluskey J. T Cell Antigen Receptor Recognition of Antigen-Presenting Molecules. Annu Rev Immunol (2015) 33:169–200. doi: 10.1146/annurev-immunol-032414-112334
132. Imbert C, Olive D. γδ T Cells in Tumor Microenvironment. Adv Exp Med Biol (2020) 1273:91–104. doi: 10.1007/978-3-030-49270-0_5
133. Orsini DL, van Gils M, Kooy YM, Struyk L, Klein G, Elsen PVD, et al. Functional and Molecular Characterization of B Cell-Responsive Vδ1+ γδ T Cells. Eur J Immunol (1994) 24(12):3199–204. doi: 10.1002/eji.1830241243
134. Häcker G, Adam S, Wagner H. Interaction Between Gamma Delta T Cells and B Cells Regulating IgG Production. Immunology (1995) 84(1):105.
135. Petrasca A, Melo AM, Breen EP, Doherty DG. Human Vδ3+ γδ T Cells Induce Maturation and IgM Secretion by B Cells. Immunol Lett (2018) 196:126–34. doi: 10.1016/j.imlet.2018.02.002
136. Scheper W, Sebestyen Z, Kuball J. Cancer Immunotherapy Using γδt Cells: Dealing With Diversity. Front Immunol (2014) 5:601. doi: 10.3389/fimmu.2014.00601
137. McCarthy NE, Eberl M. Human γδ T-Cell Control of Mucosal Immunity and Inflammation. Front Immunol (2018) 9:985. doi: 10.3389/fimmu.2018.00985
138. Hudspeth K, Fogli M, Correia DV, Mikulak J, Roberto A, Della Bella S, et al. Engagement of NKp30 on Vδ1 T Cells Induces the Production of CCL3, CCL4, and CCL5 and Suppresses HIV-1 Replication. Blood J Am Soc Hematol (2012) 119(17):4013–6. doi: 10.1182/blood-2011-11-390153
139. Mensurado S, Rei M, Lança T, Ioannou M, Goncalves-Sousa N, Kubo H, et al. Tumor-Associated Neutrophils Suppress Pro-Tumoral IL-17+ γδ T Cells Through Induction of Oxidative Stress. PloS Biol (2018) 16(5):e2004990. doi: 10.1371/journal.pbio.2004990
140. Paul S, Lal G. Regulatory and Effector Functions of Gamma–Delta (γδ) T Cells and Their Therapeutic Potential in Adoptive Cellular Therapy for Cancer. Int J Cancer (2016) 139(5):976–85. doi: 10.1002/ijc.30109
141. Lança T, Costa MF, Gonçalves-Sousa N, Rei M, Grosso AR, Penido C, et al. Protective Role of the Inflammatory CCR2/CCL2 Chemokine Pathway Through Recruitment of Type 1 Cytotoxic γδ T Lymphocytes to Tumor Beds. J Immunol (2013) 190(12):6673–80. doi: 10.4049/jimmunol.1300434
142. Glatzel A, Wesch D, Schiemann F, Brandt E, Janssen O, Kabelitz D. Patterns of Chemokine Receptor Expression on Peripheral Blood γδ T Lymphocytes: Strong Expression of CCR5 is a Selective Feature of Vδ2/Vγ9 γδ T Cells. J Immunol (2002) 168(10):4920–9. doi: 10.4049/jimmunol.168.10.4920
143. Tawfik D, Groth C, Gundlach J-P, Peipp M, Kabelitz D, Becker T, et al. TRAIL-Receptor 4 Modulates γδ T Cell-Cytotoxicity Toward Cancer Cells. Front Immunol (2019) 2044. doi: 10.3389/fimmu.2019.02044
144. Li Z, Xu Q, Peng H, Cheng R, Sun Z, Ye Z. IFN-γ Enhances HOS and U2OS Cell Lines Susceptibility to γδ T Cell-Mediated Killing Through the Fas/Fas Ligand Pathway. Int Immunopharmacol (2011) 11(4):496–503. doi: 10.1016/j.intimp.2011.01.001
145. Tokuyama H, Hagi T, Mattarollo SR, Morley J, Wang Q, Fai-So H, et al. Vγ9vδ2 T Cell Cytotoxicity Against Tumor Cells is Enhanced by Monoclonal Antibody Drugs—Rituximab and Trastuzumab. Int J Cancer (2008) 122(11):2526–34. doi: 10.1002/ijc.23365
146. Couzi L, Pitard V, Sicard X, Garrigue I, Hawchar O, Merville P, et al. Antibody-Dependent Anti-Cytomegalovirus Activity of Human γδ T Cells Expressing CD16 (Fcγriiia). Blood J Am Soc Hematol (2012) 119(6):1418–27. doi: 10.1182/blood-2011-06-363655
147. Wesch D, Kabelitz D, Oberg HH. Tumor Resistance Mechanisms and Their Consequences on γδ T Cell Activation. Immunol Rev (2020) 298(1):84–98. doi: 10.1111/imr.12925
148. Wesch D, Glatzel A, Kabelitz D. Differentiation of Resting Human Peripheral Blood γδ T Cells Toward Th1-Or Th2-Phenotype. Cell Immunol (2001) 212(2):110–7. doi: 10.1006/cimm.2001.1850
149. Caccamo N, La Mendola C, Orlando V, Meraviglia S, Todaro M, Stassi G, et al. Differentiation, Phenotype, and Function of Interleukin-17–Producing Human Vγ9vδ2 T Cells. Blood J Am Soc Hematol (2011) 118(1):129–38. doi: 10.1182/blood-2011-01-331298
150. Lafont V, Sanchez F, Laprevotte E, Michaud H-A, Gros L, Eliaou J-F, et al. Plasticity of Gamma Delta T Cells: Impact on the Anti-Tumor Response. Front Immunol (2014) 5:622. doi: 10.3389/fimmu.2014.00622
151. Peters C, Kabelitz D, Wesch D. Regulatory Functions of γδ T Cells. Cell Mol Life Sci (2018) 75(12):2125–35. doi: 10.1007/s00018-018-2788-x
152. Papotto PH, Yilmaz B, Silva-Santos B. Crosstalk Between γδ T Cells and the Microbiota. Nat Microbiol (2021); 6(9):1110–7. doi: 10.1038/s41564-021-00948-2
153. Zarobkiewicz MK, Wawryk-Gawda E, Kowalska W, Janiszewska M, Bojarska-Junak A. γδ T Lymphocytes in Asthma: A Complicated Picture. Archivum Immunol Ther Experimentalis (2021) 69(1):1–15. doi: 10.1007/s00005-021-00608-7
154. Girardi M, Oppenheim DE, Steele CR, Lewis JM, Glusac E, Filler R, et al. Regulation of Cutaneous Malignancy by γδ T Cells. Science (2001) 294(5542):605–9. doi: 10.1126/science.1063916
155. Gao Y, Yang W, Pan M, Scully E, Girardi M, Augenlicht LH, et al. γδ T Cells Provide an Early Source of Interferon γ in Tumor Immunity. J Exp Med (2003) 198(3):433–42. doi: 10.1084/jem.20030584
156. Cordova A, Toia F, La Mendola C, Orlando V, Meraviglia S, Rinaldi G, et al. Characterization of Human γδ T Lymphocytes Infiltrating Primary Malignant Melanomas. PloS One (2012) 7(11):e49878. doi: 10.1371/journal.pone.0049878
157. Wilhelm M, Kunzmann V, Eckstein S, Reimer P, Weissinger F, Ruediger T, et al. γδ T Cells for Immune Therapy of Patients With Lymphoid Malignancies. Blood (2003) 102(1):200–6. doi: 10.1182/blood-2002-12-3665
158. Street SE, Hayakawa Y, Zhan Y, Lew AM, MacGregor D, Jamieson AM, et al. Innate Immune Surveillance of Spontaneous B Cell Lymphomas by Natural Killer Cells and γδ T Cells. J Exp Med (2004) 199(6):879–84. doi: 10.1084/jem.20031981
159. Zumwalde NA, Sharma A, Xu X, Ma S, Schneider CL, Romero-Masters JC, et al. Adoptively Transferred Vγ9vδ2 T Cells Show Potent Antitumor Effects in a Preclinical B Cell Lymphomagenesis Model. JCI Insight (2017) 2(13):e93179. doi: 10.1172/jci.insight.93179
160. Perko R, Kang G, Sunkara A, Leung W, Thomas PG, Dallas MH. Gamma Delta T Cell Reconstitution is Associated With Fewer Infections and Improved Event-Free Survival After Hematopoietic Stem Cell Transplantation for Pediatric Leukemia. Biol Blood Marrow Transplant (2015) 21(1):130–6. doi: 10.1016/j.bbmt.2014.09.027
161. Handgretinger R, Schilbach K. The Potential Role of γδ T Cells After Allogeneic HCT for Leukemia. Blood J Am Soc Hematol (2018) 131(10):1063–72. doi: 10.1182/blood-2017-08-752162
162. Wang J, Lin C, Li H, Li R, Wu Y, Liu H, et al. Tumor-Infiltrating γδt Cells Predict Prognosis and Adjuvant Chemotherapeutic Benefit in Patients With Gastric Cancer. Oncoimmunology (2017) 6(11):e1353858. doi: 10.1080/2162402X.2017.1353858
163. Todaro M, D'Asaro M, Caccamo N, Iovino F, Francipane MG, Meraviglia S, et al. Efficient Killing of Human Colon Cancer Stem Cells by γδ T Lymphocytes. J Immunol (2009) 182(11):7287–96. doi: 10.4049/jimmunol.0804288
164. Meraviglia S, Lo Presti E, Tosolini M, La Mendola C, Orlando V, Todaro M, et al. Distinctive Features of Tumor-Infiltrating γδ T Lymphocytes in Human Colorectal Cancer. Oncoimmunology (2017) 6(10):e1347742. doi: 10.1080/2162402X.2017.1347742
165. Dieli F, Vermijlen D, Fulfaro F, Caccamo N, Meraviglia S, Cicero G, et al. Targeting Human γδ T Cells With Zoledronate and Interleukin-2 for Immunotherapy of Hormone-Refractory Prostate Cancer. Cancer Res (2007) 67(15):7450–7. doi: 10.1158/0008-5472.CAN-07-0199
166. Liu Z, Eltoum I-EA, Guo B, Beck BH, Cloud GA, Lopez RD. Protective Immunosurveillance and Therapeutic Antitumor Activity of γδ T Cells Demonstrated in a Mouse Model of Prostate Cancer. J Immunol (2008) 180(9):6044–53. doi: 10.4049/jimmunol.180.9.6044
167. Zhao RY, Mifsud NA, Xiao K, Chan K-F, Oveissi S, Jackson HM, et al. A Novel HLA-B18 Restricted CD8+ T Cell Epitope is Efficiently Cross-Presented by Dendritic Cells From Soluble Tumor Antigen. PloS One (2012) 7(9):e44707. doi: 10.1371/journal.pone.0044707
168. Vantourout P, Hayday A. Six-Of-the-Best: Unique Contributions of γδ T Cells to Immunology. Nat Rev Immunol (2013) 13(2):88–100. doi: 10.1038/nri3384
169. Khan MWA, Curbishley SM, Chen H-C, Thomas AD, Pircher H, Mavilio D, et al. Expanded Human Blood-Derived γδt Cells Display Potent Antigen-Presentation Functions. Front Immunol (2014) 5:344. doi: 10.3389/fimmu.2014.00344
170. Tyler CJ, Doherty DG, Moser B, Eberl M. Human Vγ9/Vδ2 T Cells: Innate Adaptors of the Immune System. Cell Immunol (2015) 296(1):10–21. doi: 10.1016/j.cellimm.2015.01.008
171. Poupot M, Pont F, Fournié J-J. Profiling Blood Lymphocyte Interactions With Cancer Cells Uncovers the Innate Reactivity of Human γδ T Cells to Anaplastic Large Cell Lymphoma. J Immunol (2005) 174(3):1717–22. doi: 10.4049/jimmunol.174.3.1717
172. Schneiders FL, Prodöhl J, Ruben JM, O'Toole T, Scheper RJ, Bonneville M, et al. CD1d-Restricted Antigen Presentation by Vγ9vδ2-T Cells Requires Trogocytosis. Cancer Immunol Res (2014) 2(8):732–40. doi: 10.1158/2326-6066.CIR-13-0167
173. Albert ML, Pearce SFA, Francisco LM, Sauter B, Roy P, Silverstein RL, et al. Immature Dendritic Cells Phagocytose Apoptotic Cells via αvβ5 and CD36, and Cross-Present Antigens to Cytotoxic T Lymphocytes. J Exp Med (1998) 188(7):1359–68. doi: 10.1084/jem.188.7.1359
174. Greenberg ME, Sun M, Zhang R, Febbraio M, Silverstein R, Hazen SL. Oxidized Phosphatidylserine–CD36 Interactions Play an Essential Role in Macrophage-Dependent Phagocytosis of Apoptotic Cells. J Exp Med (2006) 203(12):2613–25. doi: 10.1084/jem.20060370
175. Capsomidis A, Benthall G, Van Acker HH, Fisher J, Kramer AM, Abeln Z, et al. Chimeric Antigen Receptor-Engineered Human Gamma Delta T Cells: Enhanced Cytotoxicity With Retention of Cross Presentation. Mol Ther (2018) 26(2):354–65. doi: 10.1016/j.ymthe.2017.12.001
176. Korenkov DA, Laurie KL, Reading PC, Carolan LA, Chan KF, Isakova S II, et al. Safety, Immunogenicity and Protection of A(H3N2) Live Attenuated Influenza Vaccines Containing Wild-Type Nucleoprotein in a Ferret Model. Infect Genet Evol (2018) 64:95–104. doi: 10.1016/j.meegid.2018.06.019
177. Grant E, Wu C, Chan K-F, Eckle S, Bharadwaj M, Zou QM, et al. Nucleoprotein of Influenza A Virus is a Major Target of Immunodominant CD8+ T-Cell Responses. Immunol Cell Biol (2013) 91(2):184–94. doi: 10.1038/icb.2012.78
178. Laurie KL, Horman W, Carolan LA, Chan KF, Layton D, Bean A, et al. Evidence for Viral Interference and Cross-Reactive Protective Immunity Between Influenza B Virus Lineages. J Infect Dis (2018) 217(4):548–59. doi: 10.1093/infdis/jix509
179. Buonaguro L, Cerullo V. Pathogens: Our Allies Against Cancer? Mol Ther (2021) 29(1):10–2. doi: 10.1016/j.ymthe.2020.12.005
180. Bachelez H, Flageul B, Degos L, Boumsell L, Bensussan A. TCR γδ Bearing T Lymphocytes Infiltrating Human Primary Cutaneous Melanomas. J Invest Dermatol (1992) 98(3):369–74. doi: 10.1111/1523-1747.ep12499808
181. Lo Presti E, Pizzolato G, Corsale AM, Caccamo N, Sireci G, Dieli F, et al. γδ T Cells and Tumor Microenvironment: From Immunosurveillance to Tumor Evasion. Front Immunol (2018) 9:1395. doi: 10.3389/fimmu.2018.01395
182. Corsale AM, Di Simone M, Lo Presti E, Picone C, Dieli F, Meraviglia S. Metabolic Changes in Tumor Microenvironment: How Could They Affect γδ T Cells Functions? Cells (2021) 10(11):2896. doi: 10.3390/cells10112896
183. Donia M, Hansen M, Sendrup SL, Iversen TZ, Ellebæk E, Andersen MH, et al. Methods to Improve Adoptive T-Cell Therapy for Melanoma: IFN-γ Enhances Anticancer Responses of Cell Products for Infusion. J Invest Dermatol (2013) 133(2):545–52. doi: 10.1038/jid.2012.336
184. McCarthy NE, Bashir Z, Vossenkämper A, Hedin CR, Giles EM, Bhattacharjee S, et al. Proinflammatory Vδ2+ T Cells Populate the Human Intestinal Mucosa and Enhance IFN-γ Production by Colonic αβ T Cells. J Immunol (2013) 191(5):2752–63. doi: 10.4049/jimmunol.1202959
185. Riond J, Rodriguez S, Nicolau M-L, Saati T, Gairin JE. In Vivo Major Histocompatibility Complex Class I (MHCI) Expression on MHCIlow Tumor Cells is Regulated by γδ T and NK Cells During the Early Steps of Tumor Growth. Cancer Immun Archive (2009) 9(1):10. doi: 10.1158/1424-9634.DCL-10.9.1
186. Gocher AM, Workman CJ, Vignali DA. Interferon-γ: Teammate or Opponent in the Tumour Microenvironment? Nat Rev Immunol (2021) 22(3):158–72. doi: 10.1038/s41577-021-00566-3
187. Silva-Santos B, Serre K, Norell H. γδ T Cells in Cancer. Nat Rev Immunol (2015) 15(11):683–91. doi: 10.1038/nri3904
188. Gertner-Dardenne J, Bonnafous C, Bezombes C, Capietto A-H, Scaglione V, Ingoure S, et al. Bromohydrin Pyrophosphate Enhances Antibody-Dependent Cell-Mediated Cytotoxicity Induced by Therapeutic Antibodies. Blood J Am Soc Hematol (2009) 113(20):4875–84. doi: 10.1182/blood-2008-08-172296
189. Capietto A-H, Martinet L, Fournié J-J. Stimulated γδ T Cells Increase the In Vivo Efficacy of Trastuzumab in HER-2+ Breast Cancer. J Immunol (2011) 187(2):1031–8. doi: 10.4049/jimmunol.1100681
190. Hashimoto K. CD137 as an Attractive T Cell Co-Stimulatory Target in the TNFRSF for Immuno-Oncology Drug Development. Cancers (2021) 13(10):2288. doi: 10.3390/cancers13102288
191. Peters C, Oberg H-H, Kabelitz D, Wesch D. Phenotype and Regulation of Immunosuppressive Vδ2-Expressing γδ T Cells. Cell Mol Life Sci (2014) 71(10):1943–60. doi: 10.1007/s00018-013-1467-1
192. Schilbach K, Krickeberg N, Kaißer C, Mingram S, Kind J, Siegers GM, et al. Suppressive Activity of Vδ2+ γδ T Cells on αβ T Cells is Licensed by TCR Signaling and Correlates With Signal Strength. Cancer Immunol Immunother (2020) 69(4):593–610. doi: 10.1007/s00262-019-02469-8
193. Daley D, Zambirinis CP, Seifert L, Akkad N, Mohan N, Werba G, et al. γδ T Cells Support Pancreatic Oncogenesis by Restraining αβ T Cell Activation. Cell (2016) 166(6):1485–99.e15. doi: 10.1016/j.cell.2016.07.046
194. Bhagat G, Naiyer AJ, Shah JG, Harper J, Jabri B, Wang TC, et al. Small Intestinal CD8+ Tcrγδ+ NKG2A+ Intraepithelial Lymphocytes Have Attributes of Regulatory Cells in Patients With Celiac Disease. J Clin Invest (2008) 118(1):281–93. doi: 10.1172/JCI30989
195. Fisch P, Meuer E, Pende D, Rothenfußer S, Viale O, Kock S, et al. Control of B Cell Lymphoma Recognition via Natural Killer Inhibitory Receptors Implies a Role for Human Vγ9/Vδ2 T Cells in Tumor Immunity. Eur J Immunol (1997) 27(12):3368–79. doi: 10.1002/eji.1830271236
196. Halary F, Peyrat MA, Champagne E, Lopez-Botet M, Moretta A, Moretta L, et al. Control of Self-Reactive Cytotoxic T Lymphocytes Expressing γδ T Cell Receptors by Natural Killer Inhibitory Receptors. Eur J Immunol (1997) 27(11):2812–21. doi: 10.1002/eji.1830271111
197. Poccia F, Malkovsky M, Gougeon ML, Bonneville M, Lopez-Botet M, Fournié JJ, et al. γδ T Cell Activation or Anergy During Infections: The Role of Nonpeptidic TCR Ligands and HLA Class I Molecules. J Leukocyte Biol (1997) 62(3):287–91. doi: 10.1002/jlb.62.3.287
198. Poccia F, Cipriani B, Vendetti S, Colizzi V, Poquet Y, Battistini L, et al. CD94/NKG2 Inhibitory Receptor Complex Modulates Both Anti-Viral and Anti-Tumoral Responses of Polyclonal Phosphoantigen-Reactive V Gamma 9V Delta 2 T Lymphocytes. J Immunol (1997) 159(12):6009–17.
199. Bakker AB, Phillips JH, Figdor CG, Lanier LL. Killer Cell Inhibitory Receptors for MHC Class I Molecules Regulate Lysis of Melanoma Cells Mediated by NK Cells, γδ T Cells, and Antigen-Specific CTL. J Immunol (1998) 160(11):5239–45.
200. Angelini DF, Zambello R, Galandrini R, Diamantini A, Placido R, Micucci F, et al. NKG2A Inhibits NKG2C Effector Functions of γδ T Cells: Implications in Health and Disease. J Leukocyte Biol (2011) 89(1):75–84. doi: 10.1189/jlb.0710413
201. Lesport E, Baudhuin J, Sousa S, LeMaoult J, Zamborlini A, Rouas-Freiss N, et al. Inhibition of Human Vγ9vδ2 T-Cell Antitumoral Activity Through HLA-G: Implications for Immunotherapy of Cancer. Cell Mol Life Sci (2011) 68(20):3385–99. doi: 10.1007/s00018-011-0632-7
202. Xiang Z, Tu W. Dual Face of Vγ9vδ2-T Cells in Tumor Immunology: Anti-Versus Pro-Tumoral Activities. Front Immunol (2017) 8:1041. doi: 10.3389/fimmu.2017.01041
203. Niu C, Li M, Zhu S, Chen Y, Zhou L, Xu D, et al. Decitabine Inhibits Gamma Delta T Cell Cytotoxicity by Promoting KIR2DL2/3 Expression. Front Immunol (2018) 9:617. doi: 10.3389/fimmu.2018.00617
204. Naji A, Durrbach A, Carosella ED, Rouas-Freiss N. Soluble HLA-G and HLA-G1 Expressing Antigen-Presenting Cells Inhibit T-Cell Alloproliferation Through ILT-2/ILT-4/FasL-Mediated Pathways. Hum Immunol (2007) 68(4):233–9. doi: 10.1016/j.humimm.2006.10.017
205. Pende D, Falco M, Vitale M, Cantoni C, Vitale C, Munari E, et al. Killer Ig-Like Receptors (KIRs): Their Role in NK Cell Modulation and Developments Leading to Their Clinical Exploitation. Front Immunol (2019) 1179. doi: 10.3389/fimmu.2019.01179
206. André P, Denis C, Soulas C, Bourbon-Caillet C, Lopez J, Arnoux T, et al. Anti-NKG2A mAb is a Checkpoint Inhibitor That Promotes Anti-Tumor Immunity by Unleashing Both T and NK Cells. Cell (2018) 175(7):1731–43.e13. doi: 10.1016/j.cell.2018.10.014
207. Mingari MC, Pietra G, Moretta L. Immune Checkpoint Inhibitors: Anti-NKG2A Antibodies on Board. Trends Immunol (2019) 40(2):83–5. doi: 10.1016/j.it.2018.12.009
208. Fisch P, Moris A, Rammensee H-G, Handgretinger R. Inhibitory MHC Class I Receptors on γδ T Cells in Tumour Immunity and Autoimmunity. Immunol Today (2000) 21(4):187–91. doi: 10.1016/S0167-5699(99)01576-5
209. Mingari MC, Ponte M, Bertone S, Schiavetti F, Vitale C, Bellomo R, et al. HLA Class I-Specific Inhibitory Receptors in Human T Lymphocytes: Interleukin 15-Induced Expression of CD94/NKG2A in Superantigen-or Alloantigen-Activated CD8+ T Cells. Proc Natl Acad Sci (1998) 95(3):1172–7. doi: 10.1073/pnas.95.3.1172
210. Bertone S, Schiavetti F, Bellomo R, Vitale C, Ponte M, Moretta L, et al. Transforming Growth Factor-β-Induced Expression of CD94/NKG2A Inhibitory Receptors in Human T Lymphocytes. Eur J Immunol (1999) 29(1):23–9. doi: 10.1002/(SICI)1521-4141(199901)29:01<23::AID-IMMU23>3.0.CO;2-Y
211. Born WK, Huang Y, Reinhardt RL, Huang H, Sun D, O’Brien RL. γδ T Cells and B Cells. Adv Immunol (2017) 134:1–45. doi: 10.1016/bs.ai.2017.01.002
212. Rampoldi F, Ullrich L, Prinz I. Revisiting the Interaction of γδ T-Cells and B-Cells. Cells (2020) 9(3):743. doi: 10.3390/cells9030743
213. Komatsu N, Jackson HM, Chan K-F, Oveissi S, Cebon J, Itoh K, et al. Fine-Mapping Naturally Occurring NY-ESO-1 Antibody Epitopes in Melanoma Patients’ Sera Using Short Overlapping Peptides and Full-Length Recombinant Protein. Mol Immunol (2013) 54(3):465–71. doi: 10.1016/j.molimm.2013.01.014
214. King HW, Orban N, Riches JC, Clear AJ, Warnes G, Teichmann SA, et al. Single-Cell Analysis of Human B Cell Maturation Predicts How Antibody Class Switching Shapes Selection Dynamics. Sci Immunol (2021) 6(56):eabe6291. doi: 10.1126/sciimmunol.abe6291
215. Ehl S, Schwarz K, Enders A, Duffner U, Pannicke U, Kühr J, et al. A Variant of SCID With Specific Immune Responses and Predominance of γδ T Cells. J Clin Invest (2005) 115(11):3140–8. doi: 10.1172/JCI25221
216. Gil J, Busto EM, Garcillán B, Chean C, García-Rodríguez MC, Díaz-Alderete A, et al. A Leaky Mutation in CD3D Differentially Affects αβ and γδ T Cells and Leads to a Tαβ–Tγδ+ B+ NK+ Human SCID. J Clin Invest (2011) 121(10):3872–6. doi: 10.1172/JCI44254
217. Rezende RM, Da Cunha AP, Kuhn C, Rubino S, M’Hamdi H, Gabriely G, et al. Identification and Characterization of Latency-Associated Peptide-Expressing γδ T Cells. Nat Commun (2015) 6(1):1–12. doi: 10.1038/ncomms9726
218. Rhodes DA, Reith W, Trowsdale J. Regulation of Immunity by Butyrophilins. Annu Rev Immunol (2016) 34:151–72. doi: 10.1146/annurev-immunol-041015-055435
219. Barros RDM, Roberts NA, Dart RJ, Vantourout P, Jandke A, Nussbaumer O, et al. Epithelia Use Butyrophilin-Like Molecules to Shape Organ-Specific γδ T Cell Compartments. Cell (2016) 167(1):203–18.e17. doi: 10.1016/j.cell.2016.08.030
220. Wright A, Lee JE, Link MP, Smith SD, Carroll W, Levy R, et al. Cytotoxic T Lymphocytes Specific for Self Tumor Immunoglobulin Express T Cell Receptor Delta Chain. J Exp Med (1989) 169(5):1557–64. doi: 10.1084/jem.169.5.1557
221. Fisch P, Malkovsky M, Kovats S, Sturm E, Braakman E, Klein BS, et al. Recognition by Human Vγ9/Vδ2 T Cells of a GroEL Homolog on Daudi Burkitt's Lymphoma Cells. Science (1990) 250(4985):1269–73. doi: 10.1126/science.1978758
222. Fisch P, Malkovsky M, Braakman E, Sturm E, Bolhuis R, Prieve A, et al. Gamma/delta T Cell Clones and Natural Killer Cell Clones Mediate Distinct Patterns of non-Major Histocompatibility Complex-Restricted Cytolysis. J Exp Med (1990) 171(5):1567–79. doi: 10.1084/jem.171.5.1567
223. Fisch P, Oettel K, Fudim N, Surfus J, Malkovsky M, Sondel P. MHC-Unrestricted Cytotoxic and Proliferative Responses of Two Distinct Human Gamma/Delta T Cell Subsets to Daudi Cells. J Immunol (1992) 148(8):2315–23.
224. Bukowski JF, Morita CT, Tanaka Y, Bloom BR, Brenner MB, Band H. V Gamma 2V Delta 2 TCR-Dependent Recognition of non-Peptide Antigens and Daudi Cells Analyzed by TCR Gene Transfer. J Immunol (1995) 154(3):998–1006.
225. Fayen J, Tykocinski M. The Expansion of Human γδ T Cells in Response to Daudi Cells Requires the Participation of CD4+ T Cells. Immunology (1999) 97(2):272. doi: 10.1046/j.1365-2567.1999.00761.x
226. Vantourout P, Mookerjee-Basu J, Rolland C, Pont F, Martin H, Davrinche C, et al. Specific Requirements for Vγ9vδ2 T Cell Stimulation by a Natural Adenylated Phosphoantigen. J Immunol (2009) 183(6):3848–57. doi: 10.4049/jimmunol.0901085
227. Le Floch A-C, Imbert C, De Gassart A, Orlanducci F, Le Roy A, Gorvel L, et al. Targeting BTN2A1 By a Unique Activating Mab Improves Vγ9vδ2 T Cell Cytotoxicity Against Primary Acute Lymphoblastic Blasts. Blood (2021) 138:2302. doi: 10.1182/blood-2021-152577
228. Hebbeler AM, Cairo C, Cummings JS, Pauza CD. Individual Vγ2-Jγ1. 2+ T Cells Respond to Both Isopentenyl Pyrophosphate and Daudi Cell Stimulation: Generating Tumor Effectors With Low Molecular Weight Phosphoantigens. Cancer Immunol Immunother (2007) 56(6):819–29. doi: 10.1007/s00262-006-0235-6
229. Pellicci DG, Koay H-F, Berzins SP. Thymic Development of Unconventional T Cells: How NKT Cells, MAIT Cells and γδ T Cells Emerge. Nat Rev Immunol (2020) 20(12):756–70. doi: 10.1038/s41577-020-0345-y
230. Vermijlen D, Gatti D, Kouzeli A, Rus T, Eberl M. γδ T Cell Responses: How Many Ligands Will it Take Till We Know? Semin Cell Dev Biol (2018) 84:75–86. doi: 10.1016/j.semcdb.2017.10.009
231. Bald T, Krummel MF, Smyth MJ, Barry KC. The NK Cell–Cancer Cycle: Advances and New Challenges in NK Cell–Based Immunotherapies. Nat Immunol (2020) 21(8):835–47. doi: 10.1038/s41590-020-0728-z
232. Sivori S, Pende D, Quatrini L, Pietra G, Della Chiesa M, Vacca P, et al. NK Cells and ILCs in Tumor Immunotherapy. Mol Aspects Med (2021) 80:100870. doi: 10.1016/j.mam.2020.100870
233. Guillerey C. NK Cells in the Tumor Microenvironment. Tumor Microenviron (2020) 1273:69–90. doi: 10.1007/978-3-030-49270-0_4
234. Melaiu O, Lucarini V, Cifaldi L, Fruci D. Influence of the Tumor Microenvironment on NK Cell Function in Solid Tumors. Front Immunol (2020) 10:3038. doi: 10.3389/fimmu.2019.03038
235. Chan KF, Carolan LA, Druce J, Chappell K, Watterson D, Young P, et al. Pathogenesis, Humoral Immune Responses, and Transmission Between Cohoused Animals in a Ferret Model of Human Respiratory Syncytial Virus Infection. J Virol (2018) 92(4):e01322–17. doi: 10.1128/JVI.01322-17
236. Chan KF, Carolan LA, Korenkov D, Druce J, McCaw J, Reading PC, et al. Investigating Viral Interference Between Influenza A Virus and Human Respiratory Syncytial Virus in a Ferret Model of Infection. J Infect Dis (2018) 218(3):406–17. doi: 10.1093/infdis/jiy184
237. Björkström NK, Strunz B, Ljunggren H-G. Natural Killer Cells in Antiviral Immunity. Nat Rev Immunol (2021) 22(2):112–23. doi: 10.1038/s41577-021-00558-3
238. Cózar B, Greppi M, Carpentier S, Narni-Mancinelli E, Chiossone L, Vivier E. Tumor-Infiltrating Natural Killer Cells. Cancer Discov (2021) 11(1):34–44. doi: 10.1158/2159-8290.CD-20-0655
239. Wu S-Y, Fu T, Jiang Y-Z, Shao Z-M. Natural Killer Cells in Cancer Biology and Therapy. Mol Cancer (2020) 19(1):1–26. doi: 10.1186/s12943-020-01238-x
240. Liu H, Wang S, Xin J, Wang J, Yao C, Zhang Z. Role of NKG2D and its Ligands in Cancer Immunotherapy. Am J Cancer Res (2019) 9(10):2064.
241. Das H, Groh V, Kuijl C, Sugita M, Morita CT, Spies T, et al. MICA Engagement by Human Vγ2vδ2 T Cells Enhances Their Antigen-Dependent Effector Function. Immunity (2001) 15(1):83–93. doi: 10.1016/S1074-7613(01)00168-6
242. Champsaur M, Lanier LL. Effect of NKG2D Ligand Expression on Host Immune Responses. Immunol Rev (2010) 235(1):267–85. doi: 10.1111/j.0105-2896.2010.00893.x
243. Correia DV, Lopes AC, Silva-Santos B. Tumor Cell Recognition by γδ T Lymphocytes: T-Cell Receptor vs. NK-Cell Receptors. Oncoimmunology (2013) 2(1):e22892. doi: 10.4161/onci.22892
244. Raulet DH, Gasser S, Gowen BG, Deng W, Jung H. Regulation of Ligands for the NKG2D Activating Receptor. Annu Rev Immunol (2013) 31:413–41. doi: 10.1146/annurev-immunol-032712-095951
245. Silva-Santos B, Strid J. Working in “NK Mode”: Natural Killer Group 2 Member D and Natural Cytotoxicity Receptors in Stress-Surveillance by γδ T Cells. Front Immunol (2018) 9:851. doi: 10.3389/fimmu.2018.00851
246. Corvaisier M, Moreau-Aubry A, Diez E, Bennouna J, Mosnier J-F, Scotet E, et al. Vγ9vδ2 T Cell Response to Colon Carcinoma Cells. J Immunol (2005) 175(8):5481–8. doi: 10.4049/jimmunol.175.8.5481
247. Girlanda S, Fortis C, Belloni D, Ferrero E, Ticozzi P, Sciorati C, et al. MICA Expressed by Multiple Myeloma and Monoclonal Gammopathy of Undetermined Significance Plasma Cells Costimulates Pamidronate-Activated γδ Lymphocytes. Cancer Res (2005) 65(16):7502–8. doi: 10.1158/0008-5472.CAN-05-0731
248. Rincon-Orozco B, Kunzmann V, Wrobel P, Kabelitz D, Steinle A, Herrmann T. Activation of Vγ9vδ2 T Cells by NKG2D. J Immunol (2005) 175(4):2144–51. doi: 10.4049/jimmunol.175.4.2144
249. Wrobel P, Shojaei H, Schittek B, Gieseler F, Wollenberg B, Kalthoff H, et al. Lysis of a Broad Range of Epithelial Tumour Cells by Human γδ T Cells: Involvement of NKG2D Ligands and T-Cell Receptor-Versus NKG2D-Dependent Recognition. Scand J Immunol (2007) 66(2-3):320–8. doi: 10.1111/j.1365-3083.2007.01963.x
250. Kong Y, Cao W, Xi X, Ma C, Cui L, He W. The NKG2D Ligand ULBP4 Binds to Tcrγ9/δ2 and Induces Cytotoxicity to Tumor Cells Through Both Tcrγδ and NKG2D. Blood J Am Soc Hematol (2009) 114(2):310–7. doi: 10.1182/blood-2008-12-196287
251. Lawand M, Déchanet-Merville J, Dieu-Nosjean M-C. Key Features of Gamma-Delta T-Cell Subsets in Human Diseases and Their Immunotherapeutic Implications. Front Immunol (2017) 8:761. doi: 10.3389/fimmu.2017.00761
252. Gomes AQ, Correia DV, Grosso AR, Lança T, Ferreira C, Lacerda JF, et al. Identification of a Panel of Ten Cell Surface Protein Antigens Associated With Immunotargeting of Leukemias and Lymphomas by Peripheral Blood γδ T Cells. Haematologica (2010) 95(8):1397. doi: 10.3324/haematol.2009.020602
253. Lanca T, Correia DV, Moita CF, Raquel H, Neves-Costa A, Ferreira C, et al. The MHC Class Ib Protein ULBP1 is a Nonredundant Determinant of Leukemia/Lymphoma Susceptibility to γδ T-Cell Cytotoxicity. Blood J Am Soc Hematol (2010) 115(12):2407–11. doi: 10.1182/blood-2009-08-237123
254. Poggi A, Venturino C, Catellani S, Clavio M, Miglino M, Gobbi M, et al. Vδ1 T Lymphocytes From B-CLL Patients Recognize ULBP3 Expressed on Leukemic B Cells and Up-Regulated by Trans-Retinoic Acid. Cancer Res (2004) 64(24):9172–9. doi: 10.1158/0008-5472.CAN-04-2417
255. Kamei R, Yoshimura K, Yoshino S, Inoue M, Asao T, Fuse M, et al. Expression Levels of UL16 Binding Protein 1 and Natural Killer Group 2 Member D Affect Overall Survival in Patients With Gastric Cancer Following Gastrectomy. Oncol Lett (2018) 15(1):747–54. doi: 10.3892/ol.2017.7354
256. Chitadze G, Lettau M, Luecke S, Wang T, Janssen O, Fürst D, et al. NKG2D-And T-Cell Receptor-Dependent Lysis of Malignant Glioma Cell Lines by Human γδ T Cells: Modulation by Temozolomide and A Disintegrin and Metalloproteases 10 and 17 Inhibitors. Oncoimmunology (2016) 5(4):e1093276. doi: 10.1080/2162402X.2015.1093276
257. Niu C, Jin H, Li M, Zhu S, Zhou L, Jin F, et al. Low-Dose Bortezomib Increases the Expression of NKG2D and DNAM-1 Ligands and Enhances Induced NK and γδ T Cell-Mediated Lysis in Multiple Myeloma. Oncotarget (2017) 8(4):5954. doi: 10.18632/oncotarget.13979
258. Garner H, de Visser KE. Immune Crosstalk in Cancer Progression and Metastatic Spread: A Complex Conversation. Nat Rev Immunol (2020) 20(8):483–97. doi: 10.1038/s41577-019-0271-z
259. Nersesian S, Schwartz SL, Grantham SR, MacLean LK, Lee SN, Pugh-Toole M, et al. NK Cell Infiltration is Associated With Improved Overall Survival in Solid Cancers: A Systematic Review and Meta-Analysis. Trans Oncol (2021) 14(1):100930. doi: 10.1016/j.tranon.2020.100930
260. Son Y-I, Dallal RM, Mailliard RB, Egawa S, Jonak ZL, Lotze MT. Interleukin-18 (IL-18) Synergizes With IL-2 to Enhance Cytotoxicity, Interferon-γ Production, and Expansion of Natural Killer Cells. Cancer Res (2001) 61(3):884–8.
261. Caligiuri MA. Human Natural Killer Cells. Blood J Am Soc Hematol (2008) 112(3):461–9. doi: 10.1182/blood-2007-09-077438
262. Miyagawa F, Tanaka Y, Yamashita S, Minato N. Essential Requirement of Antigen Presentation by Monocyte Lineage Cells for the Activation of Primary Human γδ T Cells by Aminobisphosphonate Antigen. J Immunol (2001) 166(9):5508–14. doi: 10.4049/jimmunol.166.9.5508
263. Roelofs AJ, Jauhiainen M, Mönkkönen H, Rogers MJ, Mönkkönen J, Thompson K. Peripheral Blood Monocytes are Responsible for γδ T Cell Activation Induced by Zoledronic Acid Through Accumulation of IPP/DMAPP. Br J Haematol (2009) 144(2):245–50. doi: 10.1111/j.1365-2141.2008.07435.x
264. Constant P, Davodeau F, Peyrat M-A, Poquet Y, Puzo G, Bonneville M, et al. Stimulation of Human Gamma Delta T Cells by Nonpeptidic Mycobacterial Ligands. Science (1994) 264(5156):267–70. doi: 10.1126/science.8146660
265. Zhao X, Wei Y-Q, Kariya Y, Teshigawara K, Uchida A. Accumulation of γ/δ T Cells in Human Dysgerminoma and Seminoma: Roles in Autologous Tumor Killing and Granuloma Formation. Immunol Investigat (1995) 24(4):607–18. doi: 10.3109/08820139509066861
266. Katsuta M, Takigawa Y, Kimishima M, Inaoka M, Takahashi R, Shiohara T. NK Cells and γδ+ T Cells are Phenotypically and Functionally Defective Due to Preferential Apoptosis in Patients With Atopic Dermatitis. J Immunol (2006) 176(12):7736–44. doi: 10.4049/jimmunol.176.12.7736
267. Fowler DW, Copier J, Dalgleish AG, Bodman-Smith MD. Zoledronic Acid Causes γδ T Cells to Target Monocytes and Down-Modulate Inflammatory Homing. Immunology (2014) 143(4):539–49. doi: 10.1111/imm.12331
268. Welton JL, Morgan MP, Martí S, Stone MD, Moser B, Sewell AK, et al. Monocytes and γδ T Cells Control the Acute-Phase Response to Intravenous Zoledronate: Insights From a Phase IV Safety Trial. J Bone Mineral Res (2013) 28(3):464–71. doi: 10.1002/jbmr.1797
269. Ariasnegrete S, Keller K, Chadee K. Proinflammatory Cytokines Regulate Cyclooxygenase-2 mRNA Expression in Human Macrophages. Biochem Biophys Res Commun (1995) 208(2):582–9. doi: 10.1006/bbrc.1995.1378
270. Gonnermann D, Oberg H-H, Kellner C, Peipp M, Sebens S, Kabelitz D, et al. Resistance of Cyclooxygenase-2 Expressing Pancreatic Ductal Adenocarcinoma Cells Against γδ T Cell Cytotoxicity. Oncoimmunology (2015) 4(3):e988460. doi: 10.4161/2162402X.2014.988460
271. Tong D, Liu Q, Wang L, Xie Q, Pang J, Huang Y, et al. The Roles of the COX2/PGE2/EP Axis in Therapeutic Resistance. Cancer Metastasis Rev (2018) 37(2):355–68. doi: 10.1007/s10555-018-9752-y
272. Hashemi Goradel N, Najafi M, Salehi E, Farhood B, Mortezaee K. Cyclooxygenase-2 in Cancer: A Review. J Cell Physiol (2019) 234(5):5683–99. doi: 10.1002/jcp.27411
273. Daley D, Mani VR, Mohan N, Akkad N, Ochi A, Heindel DW, et al. Dectin 1 Activation on Macrophages by Galectin 9 Promotes Pancreatic Carcinoma and Peritumoral Immune Tolerance. Nat Med (2017) 23(5):556–67. doi: 10.1038/nm.4314
274. Seifert AM, Reiche C, Heiduk M, Tannert A, Meinecke A-C, Baier S, et al. Detection of Pancreatic Ductal Adenocarcinoma With Galectin-9 Serum Levels. Oncogene (2020) 39(15):3102–13. doi: 10.1038/s41388-020-1186-7
275. Shibata K, Yamada H, Hara H, Kishihara K, Yoshikai Y. Resident Vδ1+ γδ T Cells Control Early Infiltration of Neutrophils After Escherichia Coli Infection via IL-17 Production. J Immunol (2007) 178(7):4466–72. doi: 10.4049/jimmunol.178.7.4466
276. Nerdal PT, Peters C, Oberg H-H, Zlatev H, Lettau M, Quabius ES, et al. Butyrophilin 3a/CD277–dependent Activation of Human γδ T Cells: Accessory Cell Capacity of Distinct Leukocyte Populations. J Immunol (2016) 197(8):3059–68. doi: 10.4049/jimmunol.1600913
277. Uhlen M, Karlsson MJ, Zhong W, Tebani A, Pou C, Mikes J, et al. A Genome-Wide Transcriptomic Analysis of Protein-Coding Genes in Human Blood Cells. Science (2019) 366(6472):eaax9198. doi: 10.1126/science.aax9198
278. Monaco G, Lee B, Xu W, Mustafah S, Hwang YY, Carre C, et al. RNA-Seq Signatures Normalized by mRNA Abundance Allow Absolute Deconvolution of Human Immune Cell Types. Cell Rep (2019) 26(6):1627–40.e7. doi: 10.1016/j.celrep.2019.01.041
279. Oberg H-H, Wesch D, Kalyan S, Kabelitz D. Regulatory Interactions Between Neutrophils, Tumor Cells and T Cells. Front Immunol (2019) 10:1690. doi: 10.3389/fimmu.2019.01690
280. Fridlender ZG, Sun J, Kim S, Kapoor V, Cheng G, Ling L, et al. Polarization of Tumor-Associated Neutrophil Phenotype by TGF-β:”N1” Versus “N2” TAN. Cancer Cell (2009) 16(3):183–94. doi: 10.1016/j.ccr.2009.06.017
281. Valero C, Lee M, Hoen D, Weiss K, Kelly DW, Adusumilli PS, et al. Pretreatment Neutrophil-to-Lymphocyte Ratio and Mutational Burden as Biomarkers of Tumor Response to Immune Checkpoint Inhibitors. Nat Commun (2021) 12(1):1–9. doi: 10.1038/s41467-021-20935-9
282. Ziegler-Heitbrock L, Ancuta P, Crowe S, Dalod M, Grau V, Hart DN, et al. Nomenclature of Monocytes and Dendritic Cells in Blood. Blood J Am Soc Hematol (2010) 116(16):e74–80. doi: 10.1182/blood-2010-02-258558
283. Mildner A, Jung S. Development and Function of Dendritic Cell Subsets. Immunity (2014) 40(5):642–56. doi: 10.1016/j.immuni.2014.04.016
284. Conti L, Casetti R, Cardone M, Varano B, Martino A, Belardelli F, et al. Reciprocal Activating Interaction Between Dendritic Cells and Pamidronate-Stimulated γδ T Cells: Role of CD86 and Inflammatory Cytokines. J Immunol (2005) 174(1):252–60. doi: 10.4049/jimmunol.174.1.252
285. Collins C, Wolfe J, Roessner K, Shi C, Sigal LH, Budd RC. Lyme Arthritis Synovial γδ T Cells Instruct Dendritic Cells via Fas Ligand. J Immunol (2005) 175(9):5656–65. doi: 10.4049/jimmunol.175.9.5656
286. Martino A, Casetti R, Poccia F. Enhancement of BCG-Induced Th1 Immune Response Through Vγ9vδ2 T Cell Activation With non-Peptidic Drugs. Vaccine (2007) 25(6):1023–9. doi: 10.1016/j.vaccine.2006.09.070
287. Devilder M-C, Allain S, Dousset C, Bonneville M, Scotet E. Early Triggering of Exclusive IFN-γ Responses of Human Vγ9vδ2 T Cells by TLR-Activated Myeloid and Plasmacytoid Dendritic Cells. J Immunol (2009) 183(6):3625–33. doi: 10.4049/jimmunol.0901571
288. Eberl M, Moser B. Monocytes and γδ T Cells: Close Encounters in Microbial Infection. Trends Immunol (2009) 30(12):562–8. doi: 10.1016/j.it.2009.09.001
289. Shrestha N, Ida JA, Lubinski AS, Pallin M, Kaplan G, Haslett PA. Regulation of Acquired Immunity by γδ T-Cell/Dendritic-Cell Interactions. Ann New York Acad Sci (2005) 1062(1):79–94. doi: 10.1196/annals.1358.011
290. Martino A, Casetti R, D’Alessandri A, Sacchi A, Poccia F. Complementary Function of γδ T-Lymphocytes and Dendritic Cells in the Response to Isopentenyl-Pyrophosphate and Lipopolysaccharide Antigens. J Clin Immunol (2005) 25(3):230–7. doi: 10.1007/s10875-005-4080-8
291. Kunzmann V, Kretzschmar E, Herrmann T, Wilhelm M. Polyinosinic-Polycytidylic Acid-Mediated Stimulation of Human γδ T Cells via CD11c+ Dendritic Cell-Derived Type I Interferons. Immunology (2004) 112(3):369–77. doi: 10.1111/j.1365-2567.2004.01908.x
292. von Lilienfeld-Toal M, Sievers E, Bodemüller V, Mihailescu C, Märten A, Gorschlüter M, et al. Coculture With Dendritic Cells Promotes Proliferation But Not Cytotoxic Activity of γ/δ T Cells. Immunol Lett (2005) 99(1):103–8. doi: 10.1016/j.imlet.2005.02.001
293. Gruenbacher G, Gander H, Rahm A, Nussbaumer W, Romani N, Thurnher M. CD56+ Human Blood Dendritic Cells Effectively Promote TH1-Type γδ T-Cell Responses. Blood J Am Soc Hematol (2009) 114(20):4422–31. doi: 10.1182/blood-2009-06-227256
294. Li W, Kubo S, Okuda A, Yamamoto H, Ueda H, Tanaka T, et al. Effect of IL-18 on Expansion of γδ T Cells Stimulated by Zoledronate and IL-2. J Immunother (2010) 33(3):287–96. doi: 10.1097/CJI.0b013e3181c80ffa
295. Li H, Pauza CD. Interplay of T-Cell Receptor and Interleukin-2 Signalling in Vγ2vδ2 T-Cell Cytotoxicity. Immunology (2011) 132(1):96–103. doi: 10.1111/j.1365-2567.2010.03343.x
296. Fowler DW, Copier J, Wilson N, Dalgleish AG, Bodman-Smith MD. Mycobacteria Activate γδ T-Cell Anti-Tumour Responses via Cytokines From Type 1 Myeloid Dendritic Cells: A Mechanism of Action for Cancer Immunotherapy. Cancer Immunol Immunother (2012) 61(4):535–47. doi: 10.1007/s00262-011-1121-4
297. Cui Y, Kang L, Cui L, He W. Human γδ T Cell Recognition of Lipid A is Predominately Presented by CD1b or CD1c on Dendritic Cells. Biol Direct (2009) 4(1):1–12. doi: 10.1186/1745-6150-4-47
298. Van Acker HH, Anguille S, De Reu H, Berneman ZN, Smits EL, Van Tendeloo VF. Interleukin-15-Cultured Dendritic Cells Enhance Anti-Tumor Gamma Delta T Cell Functions Through IL-15 Secretion. Front Immunol (2018) 658. doi: 10.3389/fimmu.2018.00658
299. Fiore F, Castella B, Nuschak B, Bertieri R, Mariani S, Bruno B, et al. Enhanced Ability of Dendritic Cells to Stimulate Innate and Adaptive Immunity on Short-Term Incubation With Zoledronic Acid. Blood J Am Soc Hematol (2007) 110(3):921–7. doi: 10.1182/blood-2006-09-044321
300. Takahara M, Miyai M, Tomiyama M, Mutou M, Nicol AJ, Nieda M. Copulsing Tumor Antigen-Pulsed Dendritic Cells With Zoledronate Efficiently Enhance the Expansion of Tumor Antigen-Specific CD8+ T Cells via Vgamma9gammadelta T Cell Activation. J Leukoc Biol (2008) 83(3):742–54. doi: 10.1189/jlb.0307185
301. Su X, Zhang L, Jin L, Ye J, Guan Z, Chen R. Coculturing Dendritic Cells With Zoledronate Acid Efficiently Enhance the Anti-Tumor Effects of Cytokine-Induced Killer Cells. J Clin Immunol (2010) 30(5):766–74. doi: 10.1007/s10875-010-9434-1
302. Cabillic F, Toutirais O, Lavoué V, de la Pintiere CT, Daniel P, Rioux-Leclerc N, et al. Aminobisphosphonate-Pretreated Dendritic Cells Trigger Successful Vγ9vδ2 T Cell Amplification for Immunotherapy in Advanced Cancer Patients. Cancer Immunol Immunother (2010) 59(11):1611–9. doi: 10.1007/s00262-010-0887-0
303. Castella B, Riganti C, Fiore F, Pantaleoni F, Canepari ME, Peola S, et al. Immune Modulation by Zoledronic Acid in Human Myeloma: An Advantageous Cross-Talk Between Vγ9vδ2 T Cells, αβ CD8+ T Cells, Regulatory T Cells, and Dendritic Cells. J Immunol (2011) 187(4):1578–90. doi: 10.4049/jimmunol.1002514
304. Scotet E, Nedellec S, Devilder M-C, Allain S, Bonneville M. Bridging Innate and Adaptive Immunity Through Gammadelta T-Dendritic Cell Crosstalk. Front Biosci (2008) 13(5):6872–85. doi: 10.2741/3195
305. Galati D, Zanotta S, Bocchino M, De Filippi R, Pinto A. The Subtle Interplay Between Gamma Delta T Lymphocytes and Dendritic Cells: Is There a Role for a Therapeutic Cancer Vaccine in the Era of Combinatorial Strategies? Cancer Immunol Immunother (2021) 70(7):1797–809. doi: 10.1007/s00262-020-02805-3
306. Moser B, Eberl M. γδ T-APCs: A Novel Tool for Immunotherapy? Cell Mol Life Sci (2011) 68(14):2443–52. doi: 10.1007/s00018-011-0706-6
307. Van Acker HH, Anguille S, Van Tendeloo VF, Lion E. Empowering Gamma Delta T Cells With Antitumor Immunity by Dendritic Cell-Based Immunotherapy. Oncoimmunology (2015) 4(8):e1021538. doi: 10.1080/2162402X.2015.1021538
308. Boltjes A, Van Wijk F. Human Dendritic Cell Functional Specialization in Steady-State and Inflammation. Front Immunol (2014) 5:131. doi: 10.3389/fimmu.2014.00131
309. Sugie T, Murata-Hirai K, Iwasaki M, Morita CT, Li W, Okamura H, et al. Zoledronic Acid-Induced Expansion of γδ T Cells From Early-Stage Breast Cancer Patients: Effect of IL-18 on Helper NK Cells. Cancer Immunol Immunother (2013) 62(4):677–87. doi: 10.1007/s00262-012-1368-4
310. Fournié J-J, Sicard H, Poupot M, Bezombes C, Blanc A, Romagné F, et al. What Lessons can be Learned From γδ T Cell-Based Cancer Immunotherapy Trials? Cell Mol Immunol (2013) 10(1):35–41. doi: 10.1038/cmi.2012.39
311. Deniger DC, Moyes JS, Cooper LJ. Clinical Applications of Gamma Delta T Cells With Multivalent Immunity. Front Immunol (2014) 5:636. doi: 10.3389/fimmu.2014.00636
312. Barros MS, de Araújo ND, Magalhães-Gama F, Pereira Ribeiro TL, Alves Hanna FS, Tarragô AM, et al. γδ T Cells for Leukemia Immunotherapy: New and Expanding Trends. Front Immunol (2021) 3770. doi: 10.3389/fimmu.2021.729085
313. Le Floch A-C, Orlanducci F, Briantais A, Le Roy A, Chretien A-S, Vey N, et al. Anti-BTN3A 20.1 Agonist Monoclonal Antibody Enhances Autologous Vγ9vδ2 T Cells Cytotoxicity Against Primary Acute Myeloid Blasts. Blood (2019) 134:5153. doi: 10.1182/blood-2019-125541
314. Herrmann T, Fichtner AS, Karunakaran MM. An Update on the Molecular Basis of Phosphoantigen Recognition by Vγ9vδ2 T Cells. Cells (2020) 9(6):1433. doi: 10.3390/cells9061433
Keywords: γδ T cells, αβ T cells, B cells, dendritic cells, macrophages, monocytes, natural killer cells, neutrophils
Citation: Chan KF, Duarte JDG, Ostrouska S and Behren A (2022) γδ T Cells in the Tumor Microenvironment—Interactions With Other Immune Cells. Front. Immunol. 13:894315. doi: 10.3389/fimmu.2022.894315
Received: 11 March 2022; Accepted: 15 June 2022;
Published: 11 July 2022.
Edited by:
Dieter Kabelitz, University of Kiel, GermanyReviewed by:
Kenth Gustafsson, Great Ormond Street Institute of Child Health, University College London, United KingdomMassimo Massaia, University of Turin, Italy
Copyright © 2022 Chan, Duarte, Ostrouska and Behren. This is an open-access article distributed under the terms of the Creative Commons Attribution License (CC BY). The use, distribution or reproduction in other forums is permitted, provided the original author(s) and the copyright owner(s) are credited and that the original publication in this journal is cited, in accordance with accepted academic practice. No use, distribution or reproduction is permitted which does not comply with these terms.
*Correspondence: Andreas Behren, andreas.behren@onjcri.org.au