- Department of Medical Microbiology, School of Medicine, Nanchang University, Nanchang, China
Adjuvants have been of great interest to vaccine formulation as immune-stimulators. Prior to the recent research in the field of immune stimulation, conventional adjuvants utilized for aluminum-based vaccinations dominated the adjuvant market. However, these conventional adjuvants have demonstrated obvious defects, including poor protective efficiency and potential side effects, which hindered their widespread circulation. Outer membrane vesicles (OMVs) naturally exist in gram-negative bacteria and are capable of engaging innate and adaptive immunity and possess intrinsic adjuvant capacity. They have shown tremendous potential for adjuvant application and have recently been successfully applied in various vaccine platforms. Adjuvants could be highly effective with the introduction of OMVs, providing complete immunity and with the benefits of low toxicity; further, OMVs might also be designed as an advanced mucosal delivery vehicle for use as a vaccine carrier. In this review, we discuss adjuvant development, and provide an overview of novel OMV adjuvants and delivery vehicles. We also suggest future directions for adjuvant research. Overall, we believe that OMV adjuvants would find high value in vaccine formulation in the future.
Introduction
Vaccine adjuvants, functionally defined as non-specific immune-potentiators that provide signals or activate immune-recognition pathways or both, are capable of sustaining robust immune responses to some bacteria and viruses for long duration (Zinkernagel et al., 1997; Steinman, 2008). Conventional vaccines consist of inactivated or attenuated pathogens, but owing to their potential health hazards and risks of reversion in immune-compromised individuals (Belshe et al., 2004), adjuvants have been widely used in vaccine development (Marrack et al., 2009). Recent directions in sub-unit vaccines have also contributed to weak immunity consistent with a failure to induce an effective immune response (Skeiky et al., 2002). Therefore, adjuvant “help” for vaccine formulation is essential to overcome these weaknesses and generate strong immune protection.
Based on their mechanism of action or physicochemical properties, adjuvants could be divided into three subgroups (Allison and Byars, 1991): (I) active immune-stimulants; (II) carriers, and (III) vehicle adjuvants. For instance, Freund's complete adjuvant (FCA) and Freund's incomplete adjuvant (FIA), which mediate “a depot effect” at the injection site and increase the propagation of immune cells, could enhance the specific immune response (Lascelles et al., 1989; Jones et al., 1990); lipopolysaccharide (LPS) of Gram-negative bacteria, being an immunogenic molecule and a stimulator of Toll-like receptors (TLRs), could activate the host immune system against bacterial carriers (Audibert and Lise, 1993); and the liposome adjuvant could extend the half-life of antigens to ensure a significant antigen uptake ratio to APCs after vaccination, inclusive of adjuvant vehicles (Nakanishi et al., 1997).
Adjuvants play key roles in disease prevention and treatment. First, adjuvants influence the immune-phenotype, allowing the vaccine to produce the most effective modes of immunity for each specific pathogen (Edelman and Tacket, 2009; Schijns and Lavelle, 2011). This included potential Th1-promoting adjuvants in treating cancer since Th1 serotype immunity is critical for controlling viruses (Kennedy and Celis, 2008). Second, adjuvants could prolong the antibody response and reduce the antigen dose of immunization, thereby affecting the duration of immune responses (Gołoś and Lutynska, 2015). Third, many adjuvants have effectively facilitated the uptake by the mucosal epithelia against several infectious agents (Srivastava et al., 2015). Fourth, adjuvants could improve immune efficacy in various populations, especially neonates and geriatrics, owing to increased seroconversion and sero-protection rates (Petrovsky and Aguilar, 2004). These abilities of adjuvants are the reason for the continued interest in adjuvant development for a wide array of vaccine designs.
Limitation of Conventional Adjuvants
Incorporation of adjuvants into vaccine formulations have helped in overcoming some of the most pronounced limitations of immunization. However, the potential toxicity and adverse reactions associated with adjuvants have not been completely eliminated (Table 1).
Aluminum-based adjuvants are the most widely used for both human and veterinary vaccines. As the first excipient that had been approved in the vaccine market (Vogel and Powell, 1995), aluminum has been confirmed safe and is an effective Th2 immunity stimulator for preventing infections, such as HIV and malaria (De Milito et al., 2004; Lindblad, 2004). Nevertheless, there are several important limitations to their induction of Th1 immunity, since Th1 cells also have a critical impact on controlling infections. Further, aluminum is a toxic metal that is utilized in a liquid form in vaccine formulations, with unacceptable side effect when used in very high does (Eldred et al., 2006), including adjuvant arthritis, eosinophilia, sterile abscesses, eosinophilia, neurotoxicity, allergenicity, and myofascial pain (Allison and Byars, 1991; Exley, 2014). Finally, poor induction of mucosal immunity also limited its development (Gupta et al., 1995).
CpG adjuvants have been the subject of similar concerns, which led to investigations involving animal models and human subjects. The focus of these studies was safety based on Th1 responses (Bode et al., 2003). However, CpG motifs might reduce the apoptosis of activated lymphocytes, increase the production of auto-antibodies and pro-inflammatory cytokines, and induce TNF-α when administered in a host repeatedly or in conjunction with sub-lethal doses of LPS (Cowdery et al., 1996; Bode et al., 2003; Opal, 2010). This could result in increased host susceptibility to autoimmune disease or a predisposition to toxic shock. Several studies also indicated higher levels of pain, swelling, induration, pruritus, erythema, and systemic symptoms induced by CpG-adjuvanted vaccines (Bode et al., 2003). All these effects elevated uncertainties of potential safety profile of CpG adjuvants.
In general, an ideal adjuvant should be free from unacceptable side effects, and be safe and stable. Its manufacture should be easy, cost effective, and compatible with a wide range of vaccine components (Edelman, 1980; Alaniz et al., 2007). The potential toxicity was the most critical restraining factor to conventional adjuvant development. Additionally, the limited value regarding the minimal stimulation of immunity, led to an understanding and expectation of novel OMV adjuvants, which are safe, induce multifaceted immunity, and suitable for both animals and humans.
A Novel Vaccine Adjuvant: OMVs
Structure and Function of OMVs
OMVs are ubiquitous in Gram-negative bacteria consisting of proteins; an asymmetric distribution of lipids, mainly as LPS; and periplasmic contents (Kuehn and Kesty, 2005; Ellis and Kuehn, 2010). When bacteria encountered environmental stress, they established a colonization niche that could transport virulent factors and other materials into host cells (McBroom and Kuehn, 2007). Their reaction was an organic defense mechanism that helped pathogens create a suitable micro-environment for biofilm formation, and thus survival in hosts (Schooling and Beveridge, 2006). The effectiveness of OMVs adherence, entry, and content delivery into a host cell cytoplasm were based on the ability of the vesicles to fuse with bacterial membranes (Kulp and Kuehn, 2010). This was supported by endotoxins and lipoproteins in OMVs as conduits for the characteristic transfer of external agents at a sub-cellular level (Aliprantis et al., 2001). The application of vesicle transport has become an engineering tool for the manufacture of vaccines intended for effective antigen delivery.
Additionally, the function of OMVs, which could be used as specific toxin transporters, has proven potency is promoting the immune response, especially in T cell immunity. OMVs contain complex compounds that can be recognized by the innate and acquired immune response pathways through presentation of pathogen-associated molecular patterns (PAMPs) on OMVs. PAMPs could bind to pattern recognition receptors (PRRs) of the antigen presenting cells (ACPs) and activate naïve T cells, thereby activating the immune system (Miyaji et al., 2011). OMVs also possess intrinsic adjuvant properties, which are dependent on their natural composition, including various Toll-like receptor (TLR) antagonists, such as LPS, flagellin, peptidoglycans, lipoproteins, and other outer membrane proteins (OMPs) (Gnopo et al., 2017). Consequently, OMVs have been notable vaccine and vaccine adjuvant candidates for the application of vaccine formulations (Yi et al., 2016).
The Mechanism of OMVs Adjuvants
Since self-adjuvant properties of OMVs have been independently verifiable, the mechanism of their adjuvant activity could be explained as follows. The basis of OMV adjuvant activity includes the presence of PAMPs, induction of danger molecules, and the “geographic concept” (Figure 1) (Sanders and Feavers, 2011). PAMPs present in OMVs have been recognized by PRRs, mainly TLRs. The activation of TLRs resulted in the recruitment of cells into the immune system to stimulate APCs. This inferred that OMVs could enhance antigen uptake and induce the expression of cell surface molecules, receptors, and co-stimulatory molecules; thus, resulting in enhanced T cells production (Shen et al., 2012). Massari et al. (2006) reported that the binding of meningococcal PorB from OMVs to TLR-2 could activate human embryonic kidney cells via theTLR-2/TLR-1 complex, thereby enhancing the serum IgG titer (Massari et al., 2006). Also, some findings suggested that the induction of a danger molecule mediated by damaging host cells could enhance some signal molecules and their causal effect; these activities engaged specific mature T cells resulting in an enhanced immune responses (Siegemund et al., 2007). Furthermore, the “geographic concept” postulates increased uptake and translocation of antigens from the injection site to the tissue-draining lymph node by dendritic cells (DCs). This observation supported the hypothesis that OMVs had great potential for a vaccine platform, since they were immunogenic proteins, delivered carriers, and showed an inherent adjuvant effect.
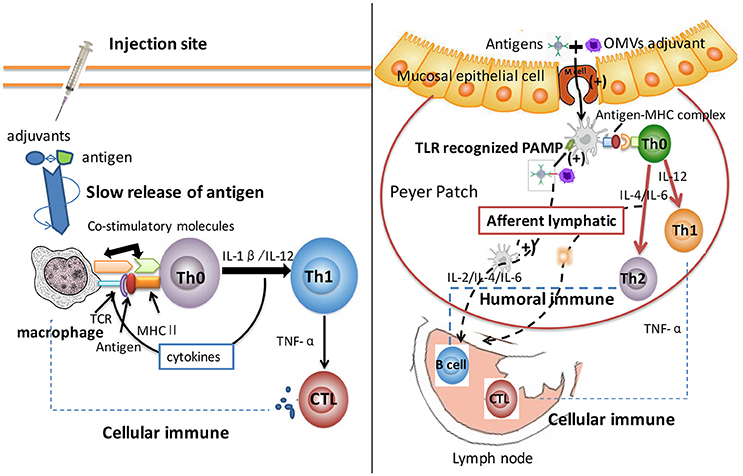
Figure 1. Comparison of aluminum and OMV adjuvants mechanism. After intake by APCs, antigen proteins were processed into smaller components and then loaded onto MHC class II molecules to formulate peptide-MHC complex. Aluminum adjuvant has been proven to induce a “depot effect” with slowly released antigens at the injection site or local lymph node; thus resulting in a prolonged immune response. Aluminum targeted the antigen to APC, and was subsequently recognized by Th0 cell, which had the same peptide-MHC complex recognized receptor. Adjuvants stabilized epitope conformation and stimulated the macrophages to induce retention and activation of Th1 immunity. This progress activated cellular immunity significantly. OMV adjuvant delivered antigen across mucosal barriers, and consequentially enhanced antigen uptake and translocation from the injection site to the tissue-draining lymph node. DCs recognized PAMPs of OMVs that led to the recruitment of immune cells and stimulated APCs through the up-regulated expression of receptors and co-stimulatory molecules. This process enhanced T helper cells production (including Th1 and Th2), and fully amplified cellular and humoral immune systems. The differences of adjuvant mechanisms between aluminum and OMVs caused different types of immune systems. Thus, OMV adjuvant triggered more comprehensive immune response and could serve as novel adjuvants for applications in vaccine development.
Development and Advantage of OMVs
OMVs have been tested on animals and humans as vaccine contents for decades, particularly for applications against the disease caused by Neisseria meningitis (N. meningitis) (Holst et al., 2009). The necessity of this vaccine formulation resulted in a demand of ideal adjuvants for vaccine use. Thus far, many successful experiments have led to the development of OMVs with proven safety and immune stimulating activities that could be developed as adjuvant tools in therapeutic applications. OMVs predisposed as a vehicle and adjuvant for nasal vaccines against meningococcal disease were firstly proposed in 1998 (Haneberg et al., 1998). Also, OMVs have been demonstrated compatible with different vaccine platforms (Katial et al., 2002), and were found to stimulate both cellular immunity and humoral immune response, thus possessing comprehensive immune-reactivity (Bottero et al., 2016). OMVs could even function as mucosal transporters to carry antigens to mucosal barriers (Pizza et al., 2001). Hence, OMVs could be an ideal vaccine adjuvant with the capacity for eliciting comprehensive immune responses, superior safety, and formulation of various mucosal vaccines.
Safety is the main concern regarding OMVs in adjuvant development. LPS, as the main structure of OMV, induced immune activity, but also become the main factor affecting the safety; therefore, a low-toxicity OMV structure designed to reduce LPS contents is necessary. LPS naturally resulted in excessive secretion of pro-inflammatory cytokines in organisms (Raetz and Whitfield, 2002); thus, ongoing investigations aimed to discover their compositions and alter their contents to improve OMV safety. Genetic engineering of OMV-producing bacteria has been a valid method to decrease toxicity and facilitate antibody response, and could effectively expand OMV safety in vaccine platforms (Leo et al., 2015). For example, gene knockout experiments conducted by Lee J. B. et al. (2009) depleted the msbB gene of OMVs in Salmonella typhimurium to yield low-endotoxic OMVs. This was then fused to the bacterial OmpA protein and constructed into the Salmonella mutation, resulting in a significant enhancement of antibody titers in mice serum (Lee S. R. et al., 2009). Also, Kim et al. (2009) established a platform technology by inactivating the MsbB (LpxM) lipid A acyltransferase; thus, generating low toxicity OMVs of Escherichia coli (E. coli) (Kim et al., 2009). These modified OMVs had both low toxicity and a foreign epitope tag that were suitable for development of multifunctional vaccine delivery vehicles. Additionally, another conservative strategy involved treating OMVs with detergent or detergent-free cell disruption techniques, which was most commonly used with sodium deoxycholate in conjunction with EDTA. The physical or chemical extraction of OMVs could selectively reduce the LPS content and also improving OMV yields (Quakyi et al., 1997).
Mucosal vaccines have been a highly beneficial strategy for preventing a majority of infectious pathogens, since mucosal surfaces are a major entry portal of many pathogenic microorganisms (Neutra et al., 1996; Sardiñas et al., 2006). Adjuvants or delivery carriers for developing an effective mucosal vaccine are essential, since pathogenic antigens alone have not been sufficient for the optimal mucosal delivery of antigens (Srivastava et al., 2015). Nevertheless, most existing adjuvants failed to deliver antigens or effectively activate mucosal immune cells, whereas OMVs-based adjuvants overcame these limitations akin to their complex compositions and functions. Casella and Mitchell (2008) reported that monophosphoryl lipid A derived from Salmonella R595, might be a promising mucosal adjuvant (Casella and Mitchell, 2008). Subsequently, Nakao et al. (2011) demonstrated that Porphyromonas gingivalis OMVs combined with Poly (I:C) could elicit enhanced secretory IgA (s-IgA) production in mucosal immune response (Nakao et al., 2011). The mechanism of OMV adjuvant processes could describe as follows: OMV is internalized into epithelial cells, when mediated though a lipid-raft-dependent endocytic pathway, and could be directed to early endosome for sorting into lysosomal compartments (Furuta et al., 2009). Effective OMVs could then release antigens recognized and processed by APCs (Ip et al., 2004). PAMPs could also attach to this conditional process for inherent presentation in the outer membrane for interaction with receptors existing in ACPs. These multi-level processes became synergistic and stimulated the production of multiple T cells. As a consensus, the induction of optimal mucosal s-IgA responses to major implements correlated with the presence of CD4 cells secreting IL-4 and IL-5 (Xu-Amano et al., 1993). Furthermore, Bergqvist et al. (2010) proposed that LPS of OMVs could also directly activate B lymphocytes and result in T cell—independent antibody production, which was thought to be a sign of mucosal immune response (Bergqvist et al., 2010). Therefore, OMVs were considered to be attractive mucosal adjuvant by inducing the immune response and transporter activity.
Application of OMVs Adjuvant
Although OMVs were discovered more than 50 years ago, together with the licensed OMV vaccine against N. meningitidis for humans, relevant research correlated to its adjuvants properties are still new and insufficient (Acevedo et al., 2014). These limited studied encouraged existing studies (Table 2) that helped in the conclusion of the current investigative finding. These conclusive data could support vaccine engineering with the use of OMV adjuvants in animal and human trials in an attempt to control the spread of bacterial and viral infections.
Neissria meningitidis as an invasive human pathogen can progress to sepsis, meningitis, and death. Owing to the significant epidemic harm that led to the progress in updated generation of meningococcal OMV vaccines, a consequential experiment associated with the adjuvants properties for N. meningitidis OMV-derived particles was also carried out (Stephens and Zimmer, 2002). In a previous report, N. meningitidis MenB OMVs were used as an adjuvant with group A meningococcal capsular polysaccharide, then this recombinant vaccine was administrated to New Zealand white rabbits to evaluate bactericidal antibody response and opsonophagocytosis activity against serogroup A meningococci (Siadat et al., 2011). Unlike most of the classic and introduced adjuvants causing local and systemic hypersensitivity reactions, OMV was a low-toxicity structure and reliable adjuvant with a high potency to induce a typical T cell response (Tavano et al., 2009). Similarly to N. meningitidis, OMVs derived from E. coli have also been engineered as adjuvants that when fused with a vesicle-localizing protein and immunogenic antigen, could strongly stimulate both cell and humoral immunity, especially mediated IFN-g and IL-17 T cell dependent response production (SciBX, 2010). Hence, OMV-based adjuvants were comparatively superior to aluminum adjuvants only for triggered B cell immunity.
Some valid strategies already existed for the effectiveness of OMV adjuvants formulated with virus or tumor vaccines. Among them, studies involved in HIV were the most comprehensive. OMV-based adjuvants could effectively enhance induction of IFN-γand IL-4 and further promote Th1-oriented responses (Alatrakchi et al., 2002). Further, OMVs combined with Virus-like particles (VLP) as an immune complex significantly induced high anti-HIV IgG production, particularly with IgG2a dominancy. In addition, a study reported that N. meningitidis MenB OMV as an adjuvant was promising in AIDs vaccine development (Aghasadeghi et al., 2011). Furthermore, a recent experiment decked OMVs with tumor antigens that elicited protective anti-tumor responses in immune-competent mice. The engineered OMVs offered synergistic protective activity, resulting in OMV platform that was particularly attractive for cancer immunotherapy (Grandi et al., 2017).
Noticeable advances in the process of proteomic and genetic engineering have led to a concern about the development of recombinant vaccines. OMVs have been attractive candidates for recombinant vaccines, since they are novel vaccine delivery platforms that enhanced recombinant engineering (Mohan et al., 2013; Afrough et al., 2017). Since the initial procedure of most biosynthesized bacterial glycans is similar, it was practical to design glycoengineered OMVs (geOMVs) as bacterial vaccine platforms. GeOMVs could display the O-antigen and surface glycans from different bacteria; thus, they could be effectively formulated with vaccines to prevent a wide array of bacterial infections (Szymanski et al., 2016; Valguarnera and Feldman, 2017). Another genetic engineering strategy involved gene-targeting technology. Plasmids could be transported into OMVs and further modify OMV lumen content, including both LPS functions and attenuated toxicity. The newly designed rOMVs could be used as a more skillful immune modulatory system directed to various vaccine platforms, and have been identified as effective in pandemic H1N1 influenza vaccines (Shim et al., 2017; Watkins et al., 2017). Overall, these findings represented a new direction in tailored vaccine design (Turner and Walper, 2017).
Concerns Associated With OMV Adjuvants
Recently, both the medical and research communities raised concerns about OMVs as novel adjuvants in controlling diseases, and it would normally led to greater investigative work. However, OMV adjuvant and related vaccine designs nevertheless raised some questions, and research activities in this potential and important field still remain nascent. OMV adjuvant properties should be at the forefront of therapeutic studies in light of their potential to prevent bacterial and viral infections. Research conclusions would possibly support the findings that OMV adjuvants needed a rational design to trigger optimal immune responses. However, owing to the toxicity of wild-type LPS, OMVs needed to be reformulated, together with some TLR antagonists occurring in OMVs, such as flagellin, lipoproteins, and other OMPs, which might cause uncontrolled responses, such as excess inflammation (Arigita et al., 2005; Thompson et al., 2005). Thus, OMV endotoxins should be removed artificially post-production. One example was the removal of Factor H binding protein from the OMPs of Neisseria (Jay Lucidarme et al., 2009). Another concern was that LPS-deficient OMVs commonly showed less effective immunogenicity than wild-type OMVs containing wild-type LPS (Gnopo et al., 2017). Therefore, an optimal balance of the appropriate modification of LPS, containing both of low-toxicity and high-immunogenicity, deserved much deeper research.
If OMVs are commercialized as adjuvants, major problems regarding mass production should be resolved. As we know, the formation mechanisms of OMVs are not explicit, and thus consistent production would be difficult (Vipond et al., 2006). For example, during the upstream process (USP) of bacterial pre-culture, an alternative to antifoam was required to scale up the USP fermentation process. Although most antifoams were not compatible with OMV production processes, and their surfactants could affect OMV integrity or interfere with the OMV purification, antifoam was still the standard method to prevent excessive foaming owing to required aeration at higher cell densities. Consequently, alternative techniques for mechanical foam breaking were be considered as part of the scale-up of the fermentation process (Baart et al., 2007; Leo et al., 2015). Moreover, external components, such as temperature, and the absorption of phages, also impacted OMV production (McBroom and Kuehn, 2007; Eddy et al., 2014). Further, oxidative stress caused by cysteine depletion in N. meningitides, or sodium carbonate in Vibiro cholera directly influenced the recombinant OMVs yield volume (van de Waterbeemd et al., 2013; Altindis et al., 2014). Therefore, it was found to be crucial to improve related production technology and internal environment conditions.
Up to now, few adjuvant candidates have been licensed for human trials as a direct result of the cost-prohibiting large-scale trials (Christensen et al., 2010). Moreover, some findings reported that LPS derivatives had adjuvant activity similar to that of wild-type LPS in mice model, but acquired no efficiency in humans; these species-specific responses were interpreted as differences in the activation and signaling of TLR complexes (Steeghs et al., 2008). These phenomena highlighted the difficulty of using animal models to evaluate the safety platform and protection of OMV candidate adjuvants in human vaccines. Therefore, to solve the challenge of OMVs-based adjuvants for human applications, more human and comparative animal trials are needed. The issue of high cost could be configured under the importance of scientific development and the likelihood of further therapeutic benefits.
The Orientation of OMVs Adjuvants
The continuous development of genomics, biochemistry, and nano-biotechnology, as well as the given advantages, have allowed for the successful development of OMV adjuvants as vaccine platforms. Nevertheless, several focuses have converged to engineer adjuvants with enhanced immunogenicity, greater safety, and wider coverage in the future.
First, current adjuvant engineering focused on higher immune potency. This included the incorporation of two or more adjuvants with different mechanisms of action to enhance the potency and type of the immune response to the vaccine antigen (Cooper and Steele, 1991). Added benefits were pursued with a synergistic effect to kill pathogens or deliver antigens. Therefore, future adjuvants offering comprehensive protection should possibly consist of multiple components. The level of protective efficiency also had a direct impact on the cost and utility of the vaccine platform.
Second, mucosal vaccines were geared toward multiple advantages, including convenient administration, non-invasiveness, high-patient compromise, and suitability for large-scale immunization (Srivastava et al., 2015). However, there are still a few traditional mucosal vaccines ready for use in their present form, owing to limitations in engineering newer, safer and more effective mucosal adjuvants (Lycke, 2012; Pritsch et al., 2016). Adjuvant binding with specific ligands could deliver antigens to specialized epithelial micro-fold cells (M cells), which are the most accessible targets for antigen delivered in mucosal lumen. OMVs could capture, adhere and transport microorganisms by endocytosis to underlying lymphoid immune cells, resulting in OMVs a promising mucosal adjuvant directed to mucosal epithelia (Jang et al., 2004). However, most adjuvant designs to date have been directed toward mucosa, without consideration of the particular role of M cell or the differences between various epithelia. Hence, more subtle designs should take into consideration that OMV adjuvants should be specifically tailored to the target mucosal epithelia (Woodrow et al., 2012).
The physicochemical characteristics of carrier components included size, surface chemistry, and hydrophobicity, also had a fatal effect on antigen crossing mucosal barriers (Rajapaksa et al., 2010; Woodrow et al., 2012). These biochemical activities were influenced by OMV types and administration routes. Studies on the transport of synthetic carriers with controlled size and surface chemistry have provided useful insight into the design criteria of mucosal delivery (Lai et al., 2007). Shakweh et al. (2005) reported that rhodamine 6G-labeled PLGA particles ranging in size from 0.3 to 1 μm were applicable when internalized by mucosal Peyer's patches (Shakweh et al., 2005). The differences in various types of OMV and immune routes have been appropriately reviewed by Gnopo et al. (2017). Accordingly, the synthetic stimuli of biochemical factors and their related sizes influenced the subsequent process of antigen delivery. Therefore, appropriate designs of OMVs played an important function in the progress of their transportation.
Review and Conclusion
As a significant component of the constitution of vaccines, adjuvants have been in development for over 80 years (Marrack et al., 2009). Nevertheless, more than a decade ago, it was necessary to modify the formulation and production of adjuvants based on queries about future direction. For example, the cost of developing new adjuvants was considered prohibitive. The needed investment of millions of US dollars for new vaccines was considered a positive return on investment owing to an expanding market for the sale of vaccines. Unfortunately, the same did not hold true for a niche product development, such as adjuvant. This limitation was contrasted to the continuing deficiencies of conventional adjuvants considering their unacceptable side-effects and incomplete immune-reactivity; inclusive of wholesale preclinical experiments that precluded the large-scale use of adjuvants.
However, new discoveries of antigen delivery through the use of OMVs have created a revolution in understanding OMV mechanisms as adjuvants. This was particularly true when OMVs were mediated in the innate immune system. The development of mucosal vaccine delivery systems has engendered new adjuvant research, which was encouraged by new knowledge driving the development of optimal structure—function relationships to produce more effective vaccine adjuvants. Genetic engineering was another fascinating development to modify OMVs for effective future use in vaccine. Although there are still several barriers to the development of OMV adjuvants, such as large-scale clinical and pre-clinical assessments, limited knowledge of OMV manufacturing process, and insufficient investments, there are many noticeable advantages to warrant detailed investigations for the development of OMV adjuvants that have exhibited comprehensive immune potency, especially in T cell immunity, higher safety, wider coverage, and a considerably robust mucosal delivery carrier. This research has also shown the potential of this recombinant vaccine for HIV-1. Compared to conventional adjuvants, OMVs showed evident superiority for future development. The advent of nano-biotechnology, progress of genomics, immunology, microbiology, and vaccine requirements, individually and combined, make it was possible to overcome the aforementioned challenges. Therefore, we consider that the newly discovered form of OMV adjuvants would progressively serve as delivery carrier platform and could be efficiently applied to efficient vaccine development.
Author Contributions
KT and RL wrote the manuscript. QL and XH revised for its integrity and accuracy. QL approved the final version of this manuscript and takes responsibility for its contents.
Funding
This study was supported by the National Natural Science Foundation of China (31760261) and the Science and Technology Research project of Jiangxi Provincial Education Department (60224).
Conflict of Interest Statement
The authors declare that the research was conducted in the absence of any commercial or financial relationships that could be construed as a potential conflict of interest.
Abbreviations
OMVs, outer membrane vesicles; FCA, Freund's complete adjuvant; FIA, Freund's incomplete adjuvant; APC, antigen-presenting cells; PRR, pattern recognition receptors; TLR, toll-like receptor; PAMP, pathogen-associated molecular patterns; LPS, lipopolysaccharides; OMPs, outer membrane vesicles; N. meningtidis; Neisseria meningitides, E. coli, Escherichia coli; geOMVs, glycoengineered OMVs; M cell, microfold cells; DCs, dendritic cells; USP, upstream process; rOMVs, recombinant outer membrane vesicles; KLH, keyhole limpet hemocyanin; PLS, Proteoliposomes; PsA, polysaccharide A; N. lactamica, Neisseria lactamica; P. aeruginosa, Pseudomonas aeruginosa; M. tuberculosis, Mycobacterium tuberculosis; HBsAg, Hepatitis B surface antigen; S. pneumonia, Streptococcus pneumonia; C. jejuni, Campylobacter jejuni; rPoA, recombinant Porin A protein; SC routes, Subcutaneous routes; IN routes, intranasal routes; IP routes, Intraperitoneal routes; IM routes, Intramuscularly routes; VLPs, virus-like particles.
References
Acevedo, R., Fernández, S., Zayas, C., Acosta, A., Sarmiento, M. E., Ferro, V. A., et al. (2014). Bacterial outer membrane vesicles and vaccine applications. Front. Immunol. 5:121. doi: 10.3389/fimmu.2014.00121
Afrough, P., Bouzari, S., Mousavi, S. F., Asadi, M. K., Vaziri, F., Fateh, A., et al. (2017). Evaluation of immunological responses to recombinant Porin A protein (rPoA) from native strains of Neisseria meningitidis serogroups A and B using OMV as an adjuvant in BALB/c mice. Microb. Pathog. 12, 209–214. doi: 10.1016/j.micpath.2017.09.038
Aghasadeghi, M. R., Salmani, A. S., Sadat, S. M., Javadi, F., Memarnejadian, A., Vahabpour, R., et al. (2011). Application of outer membrane vesicle of Neisseria meningitidis serogroup B as a new adjuvant to induce strongly Th1-oriented responses against HIV-1. Curr. HIV Res. 9, 630–635. doi: 10.2174/157016211798998772
Alaniz, R. C., Deatherage, B. L., Lara, J. C., and Cookson, B. T. (2007). Membrane vesicles are immunogenic facsimiles of Salmonella typhimurium that potently activate dendritic cells, prime B and T cell responses, and stimulate protective immunity in vivo. J. Immunol. 179, 7692–7701. doi: 10.4049/jimmunol.179.11.7692
Alatrakchi, N., Di, M. V., Thibault, V., and Autran, B. (2002). Strong CD4 Th1 responses to HIV and hepatitis C virus in HIV-infected long-term non-progressors co-infected with hepatitis C virus. AIDS 16, 713–717. doi: 10.1097/00002030-200203290-00006
Aliprantis, A. O., Weiss, D. S., Radolf, J. D., and Zychlinsky, A. (2001). Release of Toll-like receptor-2-activating bacterial lipoproteins in Shigella flexneri culture supernatants. Infect. Immun. 69, 6248–6255. doi: 10.1128/IAI.69.10.6248-6255.2001
Allison, A. C., and Byars, N. E. (1991). Immunological adjuvants: desirable properties and side-effects. Mol. Immunol. 28, 279–284. doi: 10.1016/0161-5890(91)90074-T
Altindis, E., Fu, Y., and Mekalanos, J. J. (2014). Proteomic analysis of Vibrio cholerae outer membrane vesicles. Proc. Natl. Acad. Sci. U.S.A. 111, E1548–E1556. doi: 10.1073/pnas.1403683111.
Arigita, C., Luijkx, T., Jiskoot, W., Poelen, M., Hennink, W. E., Crommelin, D. J., et al. (2005). Well-defined and potent liposomal meningococcal B vaccines adjuvated with LPS derivatives. Vaccine 23, 5091–5098. doi: 10.1016/j.vaccine.2005.06.001
Audibert, F. M., and Lise, L. D. (1993). Adjuvants: current status, clinical perspectives and future prospects. Trends Pharmacol. Sci. 14, 281–284. doi: 10.1016/0165-6147(93)90204-W
Baart, G. J., De, J. G., Philippi, M., La, V. D. P., Beuvery, E. C., et al. (2007). Scale-up for bulk production of vaccine against meningococcal disease. Vaccine 25, 6399–6408. doi: 10.1016/j.vaccine.2007.06.008
Belshe, R., Lee, M. S., Walker, R. E., Stoddard, J., and Mendelman, P. M. (2004). Safety, immunogenicity and efficacy of intranasal, live attenuated influenza vaccine. Expert Rev. Vaccines 3, 643–654. doi: 10.1586/14760584.3.6.643
Bergqvist, P., Stensson, A., Lycke, N. Y., and Bemark, M. (2010). T cell-independent IgA class switch recombination is restricted to the GALT and occurs prior to manifest germinal center formation. J. Immunol. 184, 3545–3553. doi: 10.4049/jimmunol.0901895
Bessler, W. G., Baier, W., Vd, E. U., Hoffmann, P., Heinevetter, L., Wiesmüller, K. H., et al. (1997). Bacterial lipopeptides constitute efficient novel immunogens and adjuvants in parenteral and oral immunization. Behring Inst. Mitt. 98, 390–399.
Bode, C., Zhao, G., Steinhagen, F., Kinjo, T., and Klinman, D. M. (2003). CpG DNA as a vaccine adjuvant. Expert Rev. Vaccines 2, 305–315. doi: 10.1586/14760584.2.2.305
Bottero, D., Gaillard, M. E., Zurita, E., Moreno, G., Martinez, D. S., Bartel, E., et al. (2016). Characterization of the immune response induced by pertussis OMVs-based vaccine. Vaccine 34, 3303–3309. doi: 10.1016/j.vaccine.2016.04.079
Casella, C. R., and Mitchell, T. C. (2008). Putting endotoxin to work for us: monophosphoryl lipid A as a safe and effective vaccine adjuvant. Cell. Mol. Life Sci. 65, 3231–3240. doi: 10.1007/s00018-008-8228-6
Christensen, H., Trotter, C., Hickman, M., and Edmunds, W. (2010). Modelling the cost-effectiveness of new meningococcal vaccines in England. Banff, AB: International pathogenic Neisseria conference.
Cooper, P. D., and Steele, E. J. (1991). Algammulin, a new vaccine adjuvant comprising gamma inulin particles containing alum: preparation and in vitro properties. Vaccine 9, 351–357. doi: 10.1016/0264-410X(91)90063-C
Cowdery, J. S., Chace, J. H., Yi, A. K., and Krieg, A. M. (1996). Bacterial DNA induces NK cells to produce IFN-gamma in vivo and increases the toxicity of lipopolysaccharides. J. Immunol. 156, 4570–4575.
Daliri, S. S., Kafil, H. S., Aghazadeh, M., Fateh, A., Yousefi, M., and Siadat, S. D. (2016). Extraction and biological evaluation of the membrane vesicles of Mycobacterium tuberculosis (CRBIP7.11) as an adjuvant and vaccine candidate. Jundishapur J. Microbiol. 2017:e39953. doi: 10.5812/jjm.39953
De Milito, A., Nilsson, A., Titanji, K., Thorstensson, R., Reizenstein, E., Narita, M., et al. (2004). Mechanisms of hypergammaglobulinemia and impaired antigen-specific humoral immunity in HIV-1 infection. Blood 103, 2180–2186. doi: 10.1182/blood-2003-07-2375
Dong, H. L., Kim, S. H., Kang, W., Choi, Y. S., Lee, S. H., Lee, S. R., et al. (2011). Adjuvant effect of bacterial outer membrane vesicles with penta-acylated lipopolysaccharide on antigen-specific T cell priming. Vaccine 29, 8293–8301. doi: 10.1016/j.vaccine.2011.08.102
Eddy, J. L., Gielda, L. M., Caulfield, A. J., Rangel, S. M., and Lathem, W. W. (2014). Production of outer membrane vesicles by the plague pathogen Yersinia pestis. PLoS ONE 9:e107002. doi: 10.1371/journal.pone.0107002
Edelman, R., and Tacket, C. O. (2009). Adjuvants for the future. Int. Rev. Immunol. 7, 51–66. doi: 10.3109/08830189009061764
Eldred, B. E., Dean, A. J., Mcguire, T. M., and Nash, A. L. (2006). Vaccine components and constituents: responding to consumer concerns. Med. J. Aust. 184, 170–175.
Ellis, T. N., and Kuehn, M. J. (2010). Virulence and immunomodulatory roles of bacterial outer membrane vesicles. Microbiol. Mol. Biol.Rev. Mmbr. 74, 81–94. doi: 10.1128/MMBR.00031-09
Ellis, T. N., Leiman, S. A., and Kuehn, M. J. (2010). Naturally produced outer membrane vesicles from Pseudomonas aeruginosa elicit a potent innate immune response via combined sensing of both lipopolysaccharide and protein components. Infect. Immun. 78, 3822–3831. doi: 10.1128/IAI.00433-10
Exley, C. (2014). Aluminium adjuvants and adverse events in sub-cutaneous allergy immunotherapy. Allergy Asthma Clin. Immunol. 10, 1–5. doi: 10.1186/1710-1492-10-4
Furuta, N., Tsuda, K., Omori, H., Yoshimori, T., Yoshimura, F., and Amano, A. (2009). Porphyromonas gingivalis outer membrane vesicles enter human epithelial cells via an endocytic pathway and are sorted to lysosomal compartments. Infect. Immun. 77:4187. doi: 10.1128/IAI.00009-09
Gnopo, Y. M. D., Watkins, H. C., Stevenson, T. C., DeLisa, M. P., and Putnam, D. (2017). Designer outer membrane vesicles as immunomodulatory systems - Reprogramming bacteria for vaccine delivery. Adv. Drug Deliv. Rev. 14, 132–142. doi: 10.1016/j.addr.2017.05.003
Gołoś, A., and Lutynska, A. (2015). Aluminium-adjuvanted vaccines - a review of the current state of knowledge. Przegl. Epidemiol. 69, 731–734.
Grandi, A., Tomasi, M., Zanella, I., Ganfini, L., Caproni, E., Fantappiè, L., et al. (2017). Synergistic protective activity of tumor-specific epitopes engineered in bacterial outer membrane vesicles. Front. Oncol. 7:253. doi: 10.3389/fonc.2017.00253
Gupta, R. K., Rost, B. E., Relyveld, E., and Siber, G. R. (1995). Adjuvant properties of aluminum and calcium compounds. Pharm. Biotechnol. 6, 229–248. doi: 10.1007/978-1-4615-1823-5_8
Haneberg, B., Dalseg, R., Oftung, F., Wedege, E., Høiby, E. A., Haugen, I. L., et al. (1998). Towards a nasal vaccine against meningococcal disease, and prospects for its use as a mucosal adjuvant. Dev. Biol. Stand. 92, 127–133.
Holst, J., Martin, D., Arnold, R., Huergo, C. C., Oster, P, O'Hallahan, J., et al. (2009). Properties and clinical performance of vaccines containing outer membrane vesicles from Neisseria meningitidis. Vaccine 27, B3–B12. doi: 10.1016/j.vaccine.2009.04.071
Ip, W. K. E., Charriere, G., Cho, J. H., and Ezekowitz, R. A. B. (2004). Receptors and signaling in the innate immune system. Curr. Opin. Organ Transplant. 10, 326–333. doi: 10.1097/01.mot.0000186198.62727.4b
Jackson, L. R., and Fox, J. G. (1995). Institutional policies and guidelines on adjuvants and antibody production. ILAR J. 37, 141–152. doi: 10.1093/ilar.37.3.141
Jang, M. H., Kweon, M. N., Iwatani, K., Yamamoto, M., Terahara, K., Sasakawa, C., et al. (2004). Intestinal villous M cells: an antigen entry site in the mucosal epithelium. Proc. Natl. Acad. Sci. U.S.A. 101, 6110–6115. doi: 10.1073/pnas.0400969101
Jay Lucidarme, M. C., Jamie, F., Stephen, J., Gray, E., Kaczmarski, B., Malcolm, G., et al. (2009). Characterization of fHbp, nhba (gna2132), nadA, porA, sequence type (ST), and genomic presence of IS1301 in Group B meningococcal ST269 clonal complex isolates from England and Wales. J. Clin. Microbiol. 47, 3577–3585. doi: 10.1128/JCM.00936-09
Jones, S. L., Cox, J. C., and Pearson, J. E. (1990). Increased monoclonal antibody ascites production in mice primed with Freund's incomplete adjuvant. J. Immunol. Methods 129, 227–231. doi: 10.1016/0022-1759(90)90443-Y
Katial, R. K., Brandt, B. L., Moran, E. E., Marks, S., Agnello, V., and Zollinger, W. D. (2002). Immunogenicity and safety testing of a Group B intranasal meningococcal native outer membrane vesicle vaccine. Infect. Immun. 70, 702–707. doi: 10.1128/IAI.70.2.702-707.2002
Kennedy, R., and Celis, E. (2008). Multiple roles for CD4+ T cells in anti-tumor immune responses. Immunol. Rev. 222, 129–144. doi: 10.1111/j.1600-065X.2008.00616.x
Kim, S. H., Kim, K. S., Lee, S. R., Kim, E., Kim, M. S., Lee, E. Y., et al. (2009). Structural modifications of outer membrane vesicles to refine them as vaccine delivery vehicles. Biochim. Biophys. Acta 1788, 2150–2159. doi: 10.1016/j.bbamem.2009.08.001
Kuehn, M. J., and Kesty, N. C. (2005). Bacterial outer membrane vesicles and the host–pathogen interaction. Genes Dev. 19, 2645–2655. doi: 10.1101/gad.1299905
Kulp, A., and Kuehn, M. J. (2010). Biological functions and biogenesis of secreted bacterial outer membrane vesicles. Annu. Rev. Microbiol. 64, 163–184. doi: 10.1146/annurev.micro.091208.073413
Lai, S. K., O'Hanlon, D. E., Harrold, S., Man, S. T., Wang, Y. Y., Cone, R., et al. (2007). Rapid transport of large polymeric nanoparticles in fresh undiluted human mucus. Proc. Natl. Acad. Sci. U.S.A. 104:1482. doi: 10.1073/pnas.0608611104
Lascelles, A. K., Eagleson, G., Beh, K. J., and Watson, D. L. (1989). Significance of Freund's adjuvant/antigen injection granuloma in the maintenance of serum antibody response. Vet. Immunol. Immunopathol. 22, 15–27. doi: 10.1016/0165-2427(89)90160-8
Lee, J. B., Jang, J. E., Song, M. K., and Chang, J. (2009). Intranasal delivery of cholera toxin induces th17-dominated T-cell response to bystander antigens. PLoS ONE 4:e5190. doi: 10.1371/journal.pone.0005190
Lee, S. R., Kim, S. H., Jeong, K. J., Kim, K. S., Kim, Y. H., Kim, S. J., et al. (2009). Multi-immunogenic outer membrane vesicles derived from an MsbB-deficient Salmonella enterica serovar typhimurium mutant. J. Microbiol. Biotechnol. 19, 1271–1279.
Leo, V. D. P., Michiel, S., and Peter, V. D. L. (2015). Outer membrane vesicles as platform vaccine technology. Biotechnol. J. 10, 1689–1706. doi: 10.1002/biot.201400395
Lindblad, E. B. (2004). Aluminium compounds for use in vaccines. Immunol. Cell Biol. 82, 497–505. doi: 10.1111/j.0818-9641.2004.01286.x
Lycke, N. (2012). Recent progress in mucosal vaccine development: potential and limitations. Nat. Rev. Immunol. 12, 592–605. doi: 10.1038/nri3251
Manocha, M., Pal, P. C., Chitralekha, K. T., Thomas, B. E, Tripathi, V, Gupta, S. D., et al. (2005). Enhanced mucosal and systemic immune response with intranasal immunization of mice with HIV peptides entrapped in PLG microparticles in combination with Ulex Europaeus-I lectin as M cell target. Vaccine 23, 5599–5617. doi: 10.1016/j.vaccine.2005.06.031
Marrack, P., Mckee, A. S., and Munks, M. W. (2009). Towards an understanding of the adjuvant action of aluminium. Nat. Rev. Immunol. 9, 287–293. doi: 10.1038/nri2510
Massari, P., Visintin, A., Gunawardana, J., Halmen, K. A., King, C. A., Golenbock, D. T., et al. (2006). Meningococcal porin PorB binds to TLR2 and requires TLR1 for signaling. J. Immunol. 176, 2373–2380. doi: 10.4049/jimmunol.176.4.2373
McBroom, A. J., and Kuehn, M. J. (2007). Release of outer membrane vesicles by Gram-negative bacteria is a novel envelope stress response. Mol. Microbiol. 63:545. doi: 10.1111/j.1365-2958.2006.05522.x
Miyaji, E. N., Carvalho, E., Oliveira, M. L., Raw, I., and Ho, P. L. (2011). Trends in adjuvant development for vaccines: DAMPs and PAMPs as potential new adjuvants. Braz. J. Med. Biol. Res. 44, 500–513. doi: 10.1590/S0100-879X2011000600003
Mohan, T., Verma, P., and Rao, D. N. (2013). Novel adjuvants & delivery vehicles for vaccines development: a road ahead. Ind. J. Med. Res. 138, 779–795.
Nakanishi, T., Kunisawa, J., Hayashi, A., Tsutsumi, Y., Kubo, K., Nakagawa, S., et al. (1997). Positively charged liposome functions as an efficient immunoadjuvant in inducing immune responses to soluble proteins. Biochem. Biophys. Res. Commun. 240, 793–797. doi: 10.1006/bbrc.1997.7749
Nakao, R., Hasegawa, H., Ochiai, K., Takashiba, S., Ainai, A., Ohnishi, M., et al. (2011). Outer membrane vesicles of Porphyromonas gingivalis elicit a mucosal immune response. PLoS ONE 6:e26163. doi: 10.1371/journal.pone.0026163
Neutra, M. R., Pringault, E., and Kraehenbuhl, J. P. (1996). Antigen sampling across epithelial barriers and induction of mucosal immune responses. Annu. Rev. Immunol. 14:275. doi: 10.1146/annurev.immunol.14.1.275
Opal, S. M. (2010). Endotoxins and other sepsis triggers. Contrib. Nephrol. 167, 14–24. doi: 10.1159/000315915
Orozco-Morales, M., Sánchez-García, F. J., Guevara-Salazar, P., Arrieta, O., Hernández-Pedro, N. Y., Sánchez-García, A., et al. (2012). Adjuvant immunotherapy of C6 glioma in rats with pertussis toxin. J. Cancer Res. Clin. Oncol. 138, 23–33. doi: 10.1007/s00432-011-1069-y
Petrovsky, N., and Aguilar, J. C. (2004). Vaccine adjuvants: current state and future trends. Immunol. Cell Biol. 82, 488–498. doi: 10.1111/j.0818-9641.2004.01272.x
Pizza, M., Giuliani, M. M., Fontana, M. R., Monaci, E., Douce, G., Dougan, G., et al. (2001). Mucosal vaccines: non toxic derivatives of LT and CT as mucosal adjuvants. Vaccine 19, 2534–2541. doi: 10.1016/S0264-410X(00)00553-3
Pritsch, M., Ben-Khaled, N., Chaloupka, M., Kobold, S., Berens-Riha, N., Peter, A., et al. (2016). Comparison of intranasal outer membrane vesicles with cholera toxin and injected MF59C.1 as adjuvants for malaria transmission blocking antigens AnAPN1 and Pfs48/45. J. Immunol. Res. 2016:3576028. doi: 10.1155/2016/3576028
Quakyi, E. K., Carter, P. H., Tsai, C. M., and Marti, G. E. (1997). Immunization with meningococcal membrane-bound lipooligosaccharide accelerates granulocyte recovery and enhances lymphocyte proliferation in myelosuppressed mice. Pathobiol. J. Immunopathol. Mol. Cell. Biol. 65, 26. doi: 10.1159/000164100
Raetz, C. R., and Whitfield, C. (2002). Lipopolysaccharide endotoxins. Annu. Rev. Biochem. 71, 635–700. doi: 10.1146/annurev.biochem.71.110601.135414
Rajapaksa, T. E., Bennett, K. M., Hamer, M., Lytle, C., Rodgers, V. G. J., and Lo, D. D. (2010). Intranasal M cell uptake of nanoparticles is independently influenced by targeting ligands and buffer ionic strength*. J. Biol. Chem. 285, 23739–23746. doi: 10.1074/jbc.M110.126359
Rimmelzwaan, G. F., Baars, M. A. G., Van, B. R., and Adme, O. (2001). A single dose of an ISCOM influenza vaccine induces long-lasting protective immunity against homologous challenge infection but fails to protect Cynomolgus macaques against distant drift variants of influenza A (H3N2) viruses. Vaccine 20, 158–163. doi: 10.1016/S0264-410X(01)00262-6
Romeu, B., Lastre, M., García, L., Cedré, B., Mandariote, A., Fariñas, M., et al. (2014). Combined meningococcal serogroup A and W135 outer-membrane vesicles activate cell-mediated immunity and long-term memory responses against non-covalent capsular polysaccharide A. Immunol. Res. 58, 75–85. doi: 10.1007/s12026-013-8427-6
Sanders, H., and Feavers, I. M. (2011). Adjuvant properties of meningococcal outer membrane vesicles and the use of adjuvants in Neisseria meningitidis protein vaccines. Expert Rev. Vaccines 10, 323–334. doi: 10.1586/erv.11.10
Sardiñas, G., Reddin, K., Pajon, R., and Gorringe, A. (2006). Outer membrane vesicles of Neisseria lactamica as a potential mucosal adjuvant. Vaccine 24, 206–214. doi: 10.1016/j.vaccine.2005.07.064
Schijns, V. E., and Lavelle, E. C. (2011). Trends in vaccine adjuvants. Expert Rev. Vaccines 10, 539–550. doi: 10.1586/erv.11.21
SciBX (2010). Engineered Bacterial Outer Membrane Vesicles (OMVs) as Vaccines and Vaccine Adjuvants. SciBX. doi: 10.1038/scibx.2010.134. [Epub ahead of print].
Schooling, S. R., and Beveridge, T. J. (2006). Membrane vesicles: an overlooked component of the matrices of biofilms. J. Bacteriol. 188, 5945–5957. doi: 10.1128/JB.00257-06
Shakweh, M., Besnard, M., Nicolas, V., and Fattal, E. (2005). Poly (lactide-co-glycolide) particles of different physicochemical properties and their uptake by peyer's patches in mice. Eur. J. Pharm. Biopharm. 61, 1–13. doi: 10.1016/j.ejpb.2005.04.006
Shen, Y., Giardino Torchia, M. L., Lawson, G. W., Karp, C. L., Ashwell, J. D., and Mazmanian, S. K. (2012). Outer membrane vesicles of a human commensal mediate immune regulation and disease protection. Cell Host Microbe 12, 509–520. doi: 10.1016/j.chom.2012.08.004
Shim, S. M., Song, E. J., Song, D., Lee, T. Y., Kim, D. J., Nam, J. H., et al. (2017). Nontoxic outer membrane vesicles efficiently increase the efficacy of an influenza vaccine in mice and ferrets. Vaccine 35, 3741–3748. doi: 10.1016/j.vaccine.2017.05.053
Siadat, S. D., Dezfuli, S. R. N., Zangeneh, M., Moshiri, A., Sadat, S. M., Ardestani, M. S., et al. (2011). Outer membrane vesicle of Neisseria meningitidis serogroup B as an adjuvant in immunization of rabbit against Neisseria meningitidis serogroup A. Afr. J. Microbiol. Res. 15, 3090–3095.
Siegemund, S., Schütze, N., Freudenberg, M. A., Lutz, M. B., Straubinger, R. K., and Alber, G. (2007). Production of IL-12, IL-23 and IL-27p28 by bone marrow-derived conventional dendritic cells rather than macrophages after LPS/TLR4-dependent induction by Salmonella Enteritidis. Immunobiology 212, 739–750. doi: 10.1016/j.imbio.2007.09.004
Skeiky, Y. A., Coler, R. N., Brannon, M., Stromberg, E., Greeson, K., Crane, R. T., et al. (2002). Protective efficacy of a tandemly linked, multi-subunit recombinant leishmanial vaccine (Leish-111f) formulated in MPL adjuvant. Vaccine 20, 3292–3303. doi: 10.1016/S0264-410X(02)00302-X
Srivastava, A., Gowda, D. V., Madhunapantula, S. R. V., Shinde, C. G., and Iyer, M. (2015). Mucosal vaccines: a paradigm shift in the development of mucosal adjuvants and delivery vehicles. APMIS 123:275. doi: 10.1111/apm.12351
Steeghs, L., Keestra, A. M., Van, M. A., Uronenhansson, H., Van, D. L. P., Callard, R., et al. (2008). Differential activation of human and mouse toll-like receptor 4 by the adjuvant candidate LpxL1 of Neisseria meningitidis. Infect. Immun. 76, 3801–3807. doi: 10.1128/IAI.00005-08
Steinman, R. M. (2008). Dendritic cells in vivo: a key target for a new vaccine science. Immunity 29, 319–324. doi: 10.1016/j.immuni.2008.08.001
Stephens, D. S., and Zimmer, S. M. (2002). Pathogenesis, therapy, and prevention of meningococcal sepsis. Curr. Infect. Dis. Rep. 4, 377–386. doi: 10.1007/s11908-002-0004-4
Szymanski, C. M., Valguarnera, E., Goyettedesjardins, G., Nothaft, H., Segura, M., Feldman, M. F., et al. (2016). Glycoengineered outer membrane vesicles: a novel platform for bacterial vaccines. Sci. Rep. 6:24931. doi: 10.1038/srep24931
Tavano, R., Franzoso, S., Cecchini, P., Cartocci, E., Oriente, F., Capecchi, B., et al. (2009). Self-adjuvant and immune-stimulating activity of the anti-meningococcus B vaccine candidate Neisseria meningitidis adhesin A as a soluble recombinant antigen (NadA Δ 351–405) or as part of bacterial outer membrane vesicles. New Biotechnol. 25:S6. doi: 10.1016/j.nbt.2009.06.017
Taylor, C. E. (1995). Cytokines as adjuvants for vaccines: antigen-specific responses differ from polyclonal responses. Infect. Immun. 63, 3241–3244.
Thompson, B. S., Chilton, P. M., Ward, J. R., Evans, J. T., and Mitchell, T. C. (2005). The low-toxicity versions of LPS, MPL adjuvant and RC529, are efficient adjuvants for CD4+ T cells. J. Leukoc. Biol. 78, 1273–1280. doi: 10.1189/jlb.0305172
Turner, K. B., and Walper, S. A. (2017). Bacterial outer membrane vesicles: an emerging tool in vaccine development, as adjuvants, and for therapeutic delivery. Drug Deliv. Lett. 7, 83–91. doi: 10.2174/2210303107666170725163826
Valguarnera, E., and Feldman, M. F. (2017). Glycoengineered outer membrane vesicles as a platform for vaccine development. Methods Enzymol. 597, 285–310. doi: 10.1016/bs.mie.2017.06.032
van de Waterbeemd, B., Zomer, G., van den Ijssel, J., van Keulen, L., Eppink, M. H., van der Ley, P., et al. (2013). Cysteine depletion causes oxidative stress and triggers outer membrane vesicle release by Neisseria meningitidis; implications for vaccine development. PLoS ONE 8:e54314. doi: 10.1371/journal.pone.0054314
Vipond, C., Suker, J., Jones, C., Tang, C., Feavers, I., and Wheeler, J. (2006). Proteomic analysis of a meningococcal outer membrane vesicle vaccine prepared from the group B strain NZ98/254. Proteomics 6, 3400–3413. doi: 10.1002/pmic.200500821
Vogel, F. R., and Powell, M. F. (1995). A compendium of vaccine adjuvants and excipients. Pharm. Biotechnol. 6, 141–228. doi: 10.1007/978-1-4615-1823-5_7
Watkins, H. C., Rappazzo, C. G., Higgins, J. S., Sun, X., Brock, N., Chau, A., et al. (2017). Safe recombinant outer membrane vesicles that display M2e elicit heterologous influenza protection. Mol. Ther. 25, 989–1002. doi: 10.1016/j.ymthe.2017.01.010
Woodrow, K. A., Bennett, K. M., and Lo, D. D. (2012). Mucosal vaccine design and delivery. Annu. Rev. Biomed. Eng. 14, 17–48. doi: 10.1146/annurev-bioeng-071811-150054
Xu-Amano, J., Kiyono, H., Jackson, R. J., Staats, H. F., Fujihashi, K., Burrows, P. D., et al. (1993). Helper T cell subsets for immunoglobulin A responses: oral immunization with tetanus toxoid and cholera toxin as adjuvant selectively induces Th2 cells in mucosa associated tissues. J. Exp. Med. 178, 1309–1320. doi: 10.1084/jem.178.4.1309
Yi, J., Liu, Q., and Kong, Q. (2016). Advances in outer membrane vesicles of gram-negative bacteria as sub-unit vaccines-A review. Acta Microbiol. Sin. 6, 213–220.
Keywords: outer membrane vesicles (OMVs), adjuvants, immunostimulator, vaccine, mucosal delivery carrier
Citation: Tan K, Li R, Huang X and Liu Q (2018) Outer Membrane Vesicles: Current Status and Future Direction of These Novel Vaccine Adjuvants. Front. Microbiol. 9:783. doi: 10.3389/fmicb.2018.00783
Received: 22 December 2017; Accepted: 06 April 2018;
Published: 26 April 2018.
Edited by:
José Roberto Mineo, Federal University of Uberlandia, BrazilReviewed by:
Maryam Dadar, Razi Vaccine and Serum Research Institute, IranSandip Chakraborty, College of Veterinary Sciences and Animal Husbandry, India
Copyright © 2018 Tan, Li, Huang and Liu. This is an open-access article distributed under the terms of the Creative Commons Attribution License (CC BY). The use, distribution or reproduction in other forums is permitted, provided the original author(s) and the copyright owner are credited and that the original publication in this journal is cited, in accordance with accepted academic practice. No use, distribution or reproduction is permitted which does not comply with these terms.
*Correspondence: Qiong Liu, p19890528@126.com