- 1School of Psychology, Army Medical University, Chongqing, China
- 2Department of Microbiology, College of Basic Medical Sciences, Army Medical University, Chongqing, China
Background: Microstructural alterations in the hippocampus may underlie stress-related disorders and stress susceptibility. However, whether these alterations are pre-existing stress vulnerability biomarkers or accumulative results of chronic stress remain unclear. Moreover, examining the whole hippocampus as one unit and ignoring the possibility of a lateralized effect of stress may mask some stress effects and contribute to the heterogeneity of previous findings.
Methods: After C57BL/6 mice were exposed to a 10-day chronic social defeat stress (CSDS) paradigm, different stress phenotypes, i.e., susceptible (n = 10) and resilient (n = 7) mice, were discriminated by the behavior of the mice in a social interaction test. With in vivo diffusion tensor imaging (DTI) scans that were conducted both before and after the stress paradigm, we evaluated diffusion properties in the left and right, dorsal (dHi) and ventral hippocampus (vHi) of experimental mice.
Results: A significantly lower fractional anisotropy (FA) was found in the right vHi of the susceptible mice prior to the CSDS paradigm than that found in the resilient mice, suggesting that pre-existing microstructural abnormalities may result in stress susceptibility. However, no significant group differences were found in the post-stress FA values of any of the hippocampal regions of interest (ROIs). In addition, mean diffusivity (MD) and radial diffusivity (RD) values were found to be significantly greater only in the right dHi of the resilient group compared to those of the susceptible mice. Furthermore, a significant longitudinal decrease was only observed in the right dHi RD value of the susceptible mice. Moreover, the social interaction (SI) ratio was positively related to post-stress left MD, right dHi MD, and right dHi RD values and the longitudinal right dHi MD percent change. Meanwhile, a negative relationship was detected between the SI ratio and bilateral mean of the post-stress left relative to right vHi FA value, highlighting the important role of right hippocampus in stress-resilience phenotype.
Conclusion: Our findings demonstrated different longitudinal microstructural alterations in the bilateral dHi and vHi between stress-susceptible and resilient subgroups and indicated a right-sided lateralized stress effect, which may be useful in the diagnosis and prevention of stress-related disorders as well as their intervention.
Introduction
Chronic stress contributes to several serious psychiatric disorders, including depression and post-traumatic stress disorder (PTSD) (Heim et al., 2008; Muscatell et al., 2009; Hunter et al., 2010; Pizzagalli, 2014), in which the hippocampus plays a critical regulatory role and its structure and function are affected (Smith, 2005; Amico et al., 2011; Delgado y Palacios et al., 2011; Zannas et al., 2013; Tse et al., 2014; O’Doherty et al., 2015; Otten and Meeter, 2015; Chan et al., 2016; Morey et al., 2016). The alterations in hippocampal morphology, such as changes in neuronal numbers, dendritic length and neurite density, are known to be associated with behavioral changes under stress (Czeh et al., 2006; Li et al., 2011, 2014; Yang et al., 2015; Gilabert-Juan et al., 2017). However, due to the paucity of longitudinal studies, whether the microstructural changes in the hippocampus pre-exist in stress-vulnerable individuals as a susceptible biomarker or are accumulative results caused by persisting stress exposure is still unclear (Sheline, 2011). Determining the direction of this confounding causality will be helpful for elaborating the mechanism that underlies stress-related disorders and for developing more customized interventions.
As a non-invasive imaging method, diffusion tensor imaging (DTI) can be repeatedly employed in longitudinal studies and is able to sensitively detect microstructural organization by measuring physical properties of water molecule diffusion in the tissue of interest (Alexander et al., 2007; Kumar et al., 2014). Among the most widely used diffusion indices, fractional anisotropy (FA) reflects the degree of tissue integrity and alignment of cellular structure (Beppu et al., 2005; Kinoshita et al., 2008; Anacker et al., 2016), while mean diffusivity (MD) is more related to cell density and size (Kumar et al., 2014). In addition, the radial diffusivity (RD) and axial diffusivity (AD) should be considered in the interpretation of the FA value, for they represent myelin and axonal integrity, respectively, and pathological alterations in either can lead to a decrease in FA. A combination of these four indices may represent a comprehensive understanding of the microstructural changes in the hippocampus under stress.
For longitudinal studies, given the limitations of clinical studies (Nestler and Hyman, 2010), various animal models of stress have been employed as substitutes to establish a better understanding of stress-related disorders. Among those, the chronic social defeat stress (CSDS) model mimics a psychosocial stressor in real life and not only is of great construct, face, discriminative and predictive validity but also consistently generates susceptible and resilient subgroups characterized by the presence or absence of social withdrawal in a post-stress social interaction test, respectively (Golden et al., 2011). Stress resilience, which describes when people “achieve a positive outcome in the face of adversity” (McEwen et al., 2015), has been demonstrated to be due to an active stress coping mechanism rather than simply a passive absence of stress-induced pathophysiological changes (Charney, 2004; Krishnan et al., 2007; Russo et al., 2012; Friedman et al., 2014). In addition, based on the CSDS model, previous studies have demonstrated distinctions between the two stress phenotypes from various perspectives, such as gene expression (Krishnan et al., 2007), protein expression (Carboni et al., 2006; Stelzhammer et al., 2015), and neurophysiological processes (Lagace et al., 2010; Friedman et al., 2014). An understanding of the individual differences in microstructural hippocampal changes between those susceptible and resilient to stress will be useful for elaborating the neurobiological mechanisms under stress and for developing more targeted interventions.
Moreover, structural and functional disparities have been demonstrated between the dorsal (dHi) and ventral hippocampus (vHi), in which the dHi receives input information from cortical regions and plays a role in learning and spatial memory, while the vHi projects to the prefrontal cortex and is highly connected to the amygdala, bed nucleus of stria terminalis (BNST), and hypothalamic–pituitary–adrenal (HPA) axis, leading to its involvement in emotional regulation and behavioral motivation (Fanselow and Dong, 2010; Kheirbek and Hen, 2011; Bannerman et al., 2014; O’Leary and Cryan, 2014). Moreover, there is growing evidence for hemispheric lateralization in the hippocampus (Hou et al., 2013), as well as for asymmetric effects imposed by stress (Zach et al., 2016). Therefore, studies of the whole hippocampus may mask regional-specific stress effects (Nasca et al., 2017) and contribute to the heterogeneities observed in previous studies. Accordingly, the bilateral dHi and vHi should be investigated as separate brain regions.
Therefore, in the present study, we used a repeated DTI method to explore longitudinal microstructural alterations in the bilateral dHi and vHi of CSDS-susceptible and resilient mice to test the following hypotheses: (1) basic DTI indices are capable of being sensitive to microstructural alterations in hippocampal subregions under stress; (2) a pre-existing difference in subregional hippocampal microstructure between stress-susceptible and resilient mice may exist; (3) stress may induce distinct microstructural alterations in the two hemispheres of the hippocampus and along its dorso-ventral axis.
Materials and Methods
Animals
Adult male C57BL/6 mice (C57; 6–7 weeks old; Vital River Laboratories, Beijing, China) were housed in a group of five with free access to food and water and were acclimated to an average room temperature of 22°C with 50–60% humidity and proper day and night cycle from 8 AM to 8 PM for 1 week prior to experiments. Male CD-1 mice (10 weeks old; Vital River Laboratories) were singly housed and kept under the same conditions. This study was carried out in accordance with the National Institute of Health Guide for the Care and Use of Laboratory Animals. The protocol was approved by the Ethics Committee of Third Military Medical University.
CSDS Protocol
The formal CSDS paradigm lasted for 10 days as described previously (Krishnan et al., 2007; Golden et al., 2011). Before the paradigm started, the CD-1 mice underwent a 3-day screening process. Once daily, a strange screener C57 mouse was placed directly into the home cage of the CD-1 mice for 3 min. A qualified CD-1 aggressor would attack in at least two consecutive sessions with a latency to initial aggression of less than 1 min. The chosen aggressors were then placed on the left side of adapted hamster cages (which were divided into two parts by a transparent perforated plexiglass) overnight before the formal initiation of the defeat sessions. At approximately 3:30 PM every afternoon for 10 consecutive days, each C57 mouse (n = 17) was first placed into a new aggressor’s home cage compartment for a 10-min social defeat session, followed by placement in the right side of the same cage for the remainder of the 24 h for continuous non-physical social stress exposure. On the other hand, the control C57 mice (n = 7) were housed with other C57 partners in the same adapted hamster cage, with one mouse on each side. The control mice were rotated to a new cage at the same time on a daily basis without any physical contact. After the last defeat session, all C57 mice (n = 24) were singly housed in standard mouse cages for at least 24 h before receiving the following behavior tests.
Social Interaction Test (SIT)
Before the SIT was conducted, all experimental mice were placed in the laboratory room for acclimation for at least an hour. Then, according to the protocol (Golden et al., 2011), each C57 mouse was carefully placed in the center of the social field (Figure 1A) twice, either with an empty transparent perforated enclosure (first 150-s trial) or with a completely novel CD-1 mouse contained in the enclosure (second 150-s trial). Between the two trials, the C57 mice were placed back in their own cages for a 30-s rest period. The exploratory activity of the C57 mice in the two trials was recorded and analyzed by a video-tracking apparatus and software (Xinruan Information Technology Co., Shanghai, China). For each mouse, the social interaction (SI) ratio was calculated as (interaction time, CD-1 present)/(interaction time, CD-1 absent) × 100%, and the corner ratio was calculated as (corner time, CD-1 present)/(corner time, CD-1 absent) × 100%.
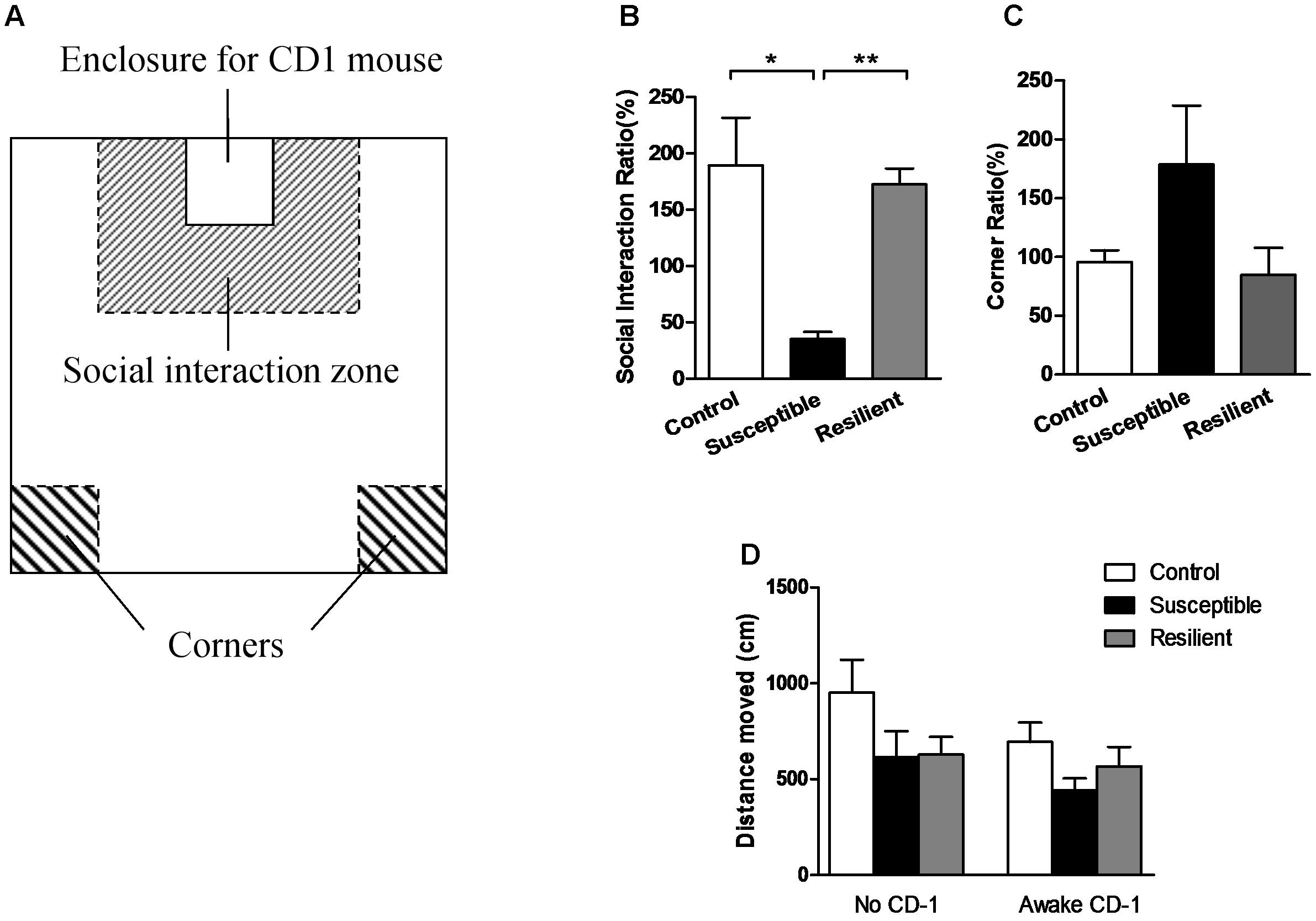
FIGURE 1. Social interaction test. (A) Schematic diagram of the social interaction arena. (B) Susceptible mice had a significantly lower SI ratio. (C) Susceptible mice had a non-significantly higher corner ratio. (D) Three groups displayed a comparable distance moved in the two separate trials. Data were presented as mean ± SEM; ∗P < 0.05, ∗∗P < 0.01.
In vivo DTI Data Acquisition and Processing
To obtain longitudinal data, we conducted in vivo DTI scans twice, both before and after the CSDS paradigm, with a 7T magnetic resonance imaging (MRI) scanner (Bruker Bio Spec 70/20 USR). Experimental mice were initially anesthetized with 5% isoflurane, then placed on the scanning bed, and maintained with 1–2% isoflurane, and their respiration rate and body temperature were monitored throughout the DTI procedures. The portion of the brain between the olfactory bulb and the anterior cerebellum was scanned with the following parameters: TE = 25 ms, TR = 3000 ms, band width = 20000 Hz, slices = 10, slice thickness = 1 mm, b-value = 1000 s/mm2, diffusion directions = 30, gradient duration = 4.5 ms, gradient separation = 10.5 ms, image size = 128 × 128, FOV = 20 mm × 20 mm, and segments = 4. The data were processed using DTI-Studio software1. Motion correction and eddy current correction was performed by an automated image and registration package. The data were then interpolated to attain isotropic voxels and decoded to obtain the tensor field data, which were used to obtain the eigenvalues (λ1, λ2, λ3) and orthonormal eigenvectors (e1, e2, e3) and to compute the DTI indices with the following equations: ; MD = ⅓ Trace = ⅓ (λ1 + λ2 + λ3); RD = ½ (λ2 + λ3); AD = λ1. Regions of interest (ROIs), including bilateral dHi and vHi, were manually drawn on the B0 images and the generated FA maps according to the mouse atlas (Paxinos and Franklin, 2001), Allen Mouse Brain Atlas2 (Lein et al., 2007) and previous studies (Kleinknecht et al., 2012; Reichel et al., 2017) by experimenters who were blind to group assignments (Figure 2). DTI indices were generated based on each ROI. Longitudinal percent change of DTI data was calculated as 100% × (post–pre)/pre. The degree of hemispheric asymmetry in hippocampal microstructure was also considered, which was calculated as the percentage difference between left and right ROIs’ diffusivity values: 100% × 2 × (left - right)/(left + right) (Madsen et al., 2012).
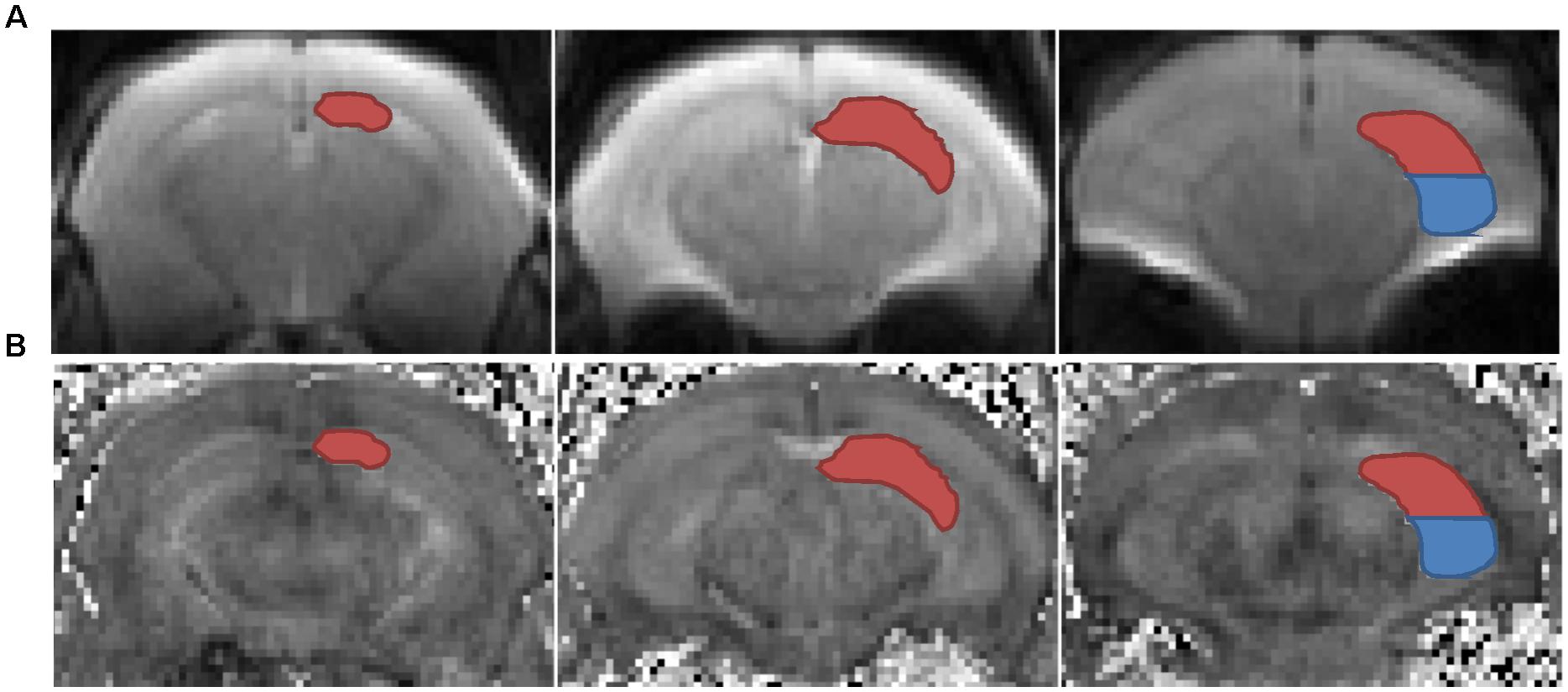
FIGURE 2. Left dorsal (red) and ventral (blue) hippocampus were delineated in representative coronal B0 maps (A) and FA maps (B). ROIs, regions of interest.
Statistical Analyses
The statistical analysis was carried out using SPSS 18 (SPSS, Chicago, IL, United States) and illustrated with GraphPad Prism 5 (GraphPad Software, San Diego, CA, United States). Data were expressed as the mean ± SEM values. For the comparisons of the three groups (i.e., the susceptible, resilient and control groups), one-way analysis of variance (ANOVA) and two-sided Bonferroni post hoc test were used. Spearman’s correlation analysis was performed to examine the relationship between diffusivity values and SI ratios. The statistical significance level was set at P < 0.05.
Results
Social Interaction Test
The stressed mice were divided into two subgroups, with the susceptible mice (n = 10) defined as those with an SI ratio less than 100%, and the resilient mice (n = 7) defined as those with an SI ratio greater than 100%. The susceptible mice spent less time in the interaction zone or even hid in the corners when there was an aggressive CD-1 mouse present in the perforated enclosure, even though these mice had never previously interacted with this particular CD-1 mouse, giving them a significantly lower SI ratio [Figure 1B; F(2, 21) = 15.000, P = 0.000; post hoc comparison: control vs. susceptible, P = 0.028; control vs. resilient, P = 0.973; susceptible vs. resilient, P = 0.000] and non-significantly higher corner ratio [Figure 1C; F(2,21) = 1.897, P = 0.175] than the other two groups. Moreover, the three groups displayed a comparable distance moved in the two separate trials [Figure 1D; F(2,21) = 0.545, P = 0.588], suggesting that the differences in social interaction had no correlation with the locomotory activity of the mice.
Diffusion Properties of the Examined ROIs
Prior to the CSDS paradigm, a significantly lower FA value was found in the right vHi of the susceptible mice than in that of the resilient group [Table 1; F(2, 21) = 3.896, P = 0.039; post hoc comparison: control vs. susceptible, P = 0.165; control vs. resilient, P = 0.998; susceptible vs. resilient, P = 0.047], suggesting that a pre-existing deficiency in the tissue integrity of the right vHi may be present in susceptible mice. However, there was no significant group difference in the FA values of any of the ROIs after stress exposure. In contrast, the MD (Table 2) and RD values (Table 3) presented a similar trend to one another; there was no significant difference in the MD or RD values of any ROI prior to stress, but after stress exposure, the resilient mice presented a higher MD and RD value in the right dHi than susceptible mice [MD: F(2, 21) = 3.800, P = 0.045; post hoc comparison: control vs. susceptible, P = 0.999, control vs. resilient, P = 0.805, susceptible vs. resilient, P = 0.042, RD: F(2,21) = 7.164, P = 0.006; post hoc comparison: control vs. susceptible, P = 0.618, control vs. resilient, P = 0.434, susceptible vs. resilient, P = 0.005], suggesting that a unique alteration may occur in the right dHi of resilient mice. However, there was no significant difference in AD values in either the pre- or post-stress comparisons (Table 4).
In the longitudinal comparisons (Supplementary Table S1), a significant difference was only found in the RD value of the right dHi, which decreased in the susceptible group more than in the resilient group [control = −47.67 ± 0.58%, susceptible = −50.45 ± 1.42%, resilient = −41.01 ± 3.32%; F(2, 21) = 5.225, P = 0.018; post hoc comparison: control vs. susceptible, P = 0.999, control vs. resilient, P = 0.349, susceptible vs. resilient, P = 0. 016].
Associations Between the SI Ratio and Diffusion Properties
Correlation analysis (Supplementary Table S2) revealed a significant positive relationship between the SI ratio and post-stress left MD (r24 = 0.497, P = 0.031), right dHi MD (r24 = 0.507, P = 0.027), and right dHi RD values (r24 = 0.594, P = 0.007), as well as the percent change in the longitudinal right dHi MD (r24 = 0.504, P = 0.028), suggesting that the microstructural alterations reflected by these regional DTI indices may contribute some protection associated with stress resilience. However, no significant correlations were found between the SI ratio and any pre-stress DTI indices.
To test whether the stress paradigm imposed an asymmetric effect on the hippocampus, the associations between the SI ratio and the hippocampal bilateral mean of the four DTI indices were analyzed. A negative relationship was found between the SI ratio and post-stress left relative to right vHi FA (Supplementary Table S2; r24 = −0.570, P = 0.011). That is, the higher the post-stress right relative to left vHi FA, the higher the SI ratio and the greater the stress resilience, suggesting a protective role of the right ventral hippocampal tissue integrity under stress.
Discussion
To examine whether there are hippocampal microstructural alterations underlying chronic social stress susceptibility, we used a longitudinal in vivo DTI method to document the changes in hippocampal diffusion pattern in a CSDS model using basic DTI indices, aiming to contribute to the understanding of the involved causality. Meanwhile, we also investigated whether there are asymmetric effects imposed by stress between the left and right as well as the dorsal and ventral portions of the hippocampus, which may be of therapeutic and preventive value for stress-related psychiatric disorders.
Consistent with our hypothesis, prior to CSDS, a significantly lower FA value was found in the right vHi of the susceptible mice compared to that of the resilient group, which may have contributed to the division of the following behavioral response to stress. The FA value reflects cell number, tissue density, and cell structure alignment; therefore, this pre-existing lower FA value may result from an inconsistent orientation, reduced cell structure alignment, decreased myelination or increased interference from neuroinflammation, gliosis or other factors that limit the diffusion of water molecules in the right vHi of the susceptible mice (Beppu et al., 2005; Alexander et al., 2007; Kinoshita et al., 2008; Evans, 2013; Kumar et al., 2014; Anacker et al., 2016). Concerning cellular changes have been demonstrated postmortem in the hippocampus of major depressive disorder (MDD) (Stockmeier et al., 2004) and PTSD patients (Krystal and Duman, 2004). However, due to the paucity of longitudinal studies, including pre-stress hippocampal DTI data, these assumptions still need to be verified with further research combined with other methods.
No significant group differences were found in the post-stress FA values of any of the hippocampal ROIs examined, nor were any significant correlations between SI ratios and pre-stress, post-stress or longitudinal changes in FA values observed, which is consistent with previous negative results (Delgado y Palacios et al., 2011; Kumar et al., 2014; Khan et al., 2016). However, Anacker et al. (2016), with an ex vivo MRI method, revealed that the hippocampal FA value of CSDS mice were positively correlated with their social avoidance index, suggesting that water diffusion is more anisotropic in stress-susceptible mice. This disparity may be due to the differences between the in vivo and ex vivo experimental conditions, making the results difficult to compare directly (Khan et al., 2016). Moreover, the correlation was not significant in the independently analyzed control group in the ex vivo study; therefore, an analysis including all three groups may reveal that the mild difference observed in the stressed groups is no longer significant. On the other hand, in a recent clinical study (Srivastava et al., 2016), a significantly lower FA value was found in the right hippocampus of patients with first-episode, treatment-naive MDD than that found in the right hippocampus of healthy controls and was believed to potentially reflect the decrease in hippocampal volume of MDD patients found in previous studies (Frodl et al., 2002; Malykhin et al., 2010). Therefore, macrostructural volume changes in the hippocampus may be reflected by alterations in its microstructure.
In addition, according to the mathematical definition of the FA value, its interpretation needs to be combined with MD, RD and AD values. No significant differences were observed in pre-stress MD, RD, or AD values in any of the hippocampal ROIs examined among the three groups. After exposure to the CSDS paradigm, a significant increase in MD and RD values was only observed in the right dHi of the resilient group, indicating that a unique change may occur in this region of the resilient brain. The MD value in the hippocampus is generally considered to be closely related to hippocampal function. For example, in healthy individuals, people with higher education (Piras et al., 2011) or better memory function (Carlesimo et al., 2010) have been demonstrated to have a lower hippocampal MD value. Higher MD values may result from reduced membrane density and may also reflect neuronal arborization or increased extracellular space (Londono et al., 2003; Kumar et al., 2014). Meanwhile, a higher RD value in white matter is usually associated with demyelination or dysmyelination (Song et al., 2002). In the hippocampus, however, there are two other possible explanations for an increase in RD: dendritic atrophy and loss of neurites may lead to a decrease in directional diffusion of water molecules, or augmented arborization may increase the diffusion directions along dendrites (Delgado y Palacios et al., 2014). Both the concerning postmortem examinations (Stockmeier et al., 2004) and studies conducted in various animal models of stress seem to support the latter possibility. For example, reduced interneuronal dendritic arborization in the hippocampal CA1 region was found in chronic mild restraint-stressed mice (Gilabert-Juan et al., 2017). Furthermore, chronic psychosocial stress was shown to result in decreased astroglial plasticity in the hippocampus (Czeh et al., 2006), and the density of dendritic spines in the hippocampus of susceptible mice was found to be significantly lower than in the hippocampus of control and stress-resilient mice in the learned helplessness (Yang et al., 2015) and chronic mild stress (CMS) (Li et al., 2011, 2014) paradigms. In addition, direct evidence of plastic changes has been shown to occur in the hippocampus of stress-resilient subgroups, including neurogenesis in the dentate gyrus and dendrite and synapse remodeling in major neurons of Ammon’s horn (Li et al., 2014; McEwen et al., 2015). Moreover, from the perspective of treatment, anti-depressants, such as imipramine, have been shown to prevent depressive-like behaviors through increasing dendritic branching of immature neurons (Fenton et al., 2015) and contribute to resilience to CMS re-exposure by re-establishing hippocampal neurogenesis and neuronal dendritic arborization (Alves et al., 2017). Although further studies are needed to shed light on the underlying mechanisms of the diffusion changes observed in the right hippocampus of resilient individuals, the increased MD and RD values observed in the resilient mice in the present study may result from augmented dendritic arborization and neuronal plasticity.
In the examination of the AD value, no significant group differences or longitudinal changes were found in the hippocampus, which was also negative in another study of CMS paradigm (Delgado y Palacios et al., 2011). However, a decreased AD value, not FA, MD or RD, was detected in the left hippocampus of mice exposed to CMS compared to that in the left hippocampus of controls, which indicated a destruction of neurofibrils (Kumar et al., 2014). The inconsistency of these results could be attributed to the different stress paradigms that were applied. The lack of a differentiation between susceptible animals and those that are resilient may have also masked subtle hippocampal changes in stress-induced subgroups. Moreover, in a recent study of veterans (Waltzman et al., 2017), the right hippocampal AD value was significantly higher in the PTSD group than in the traumatic brain injury group; however, compared to that of the control group, there were no significant differences in the bilateral hippocampal DTI indices of those individuals with PTSD. This finding demonstrated that no significant axonal injury in the hippocampus of PTSD patients was detected by DTI, which is consistent with our findings.
Our findings also revealed an asymmetric effect induced by stress on microstructural changes in hippocampal subregions, emphasizing the role of the right hippocampus in the underlying mechanisms of stress-related disorders. Glucocorticoids may cause the lateral effect of stress on the hippocampus, which has been predominantly observed in the right side (Tang et al., 2008; Zach et al., 2010, 2016; Frodl and O’Keane, 2012). However, higher HPA axis activation (basal cortisol levels) has been found to be associated with higher left relative to right hippocampal MD, suggesting that either the left hippocampus plays a lateralized regulatory role in HPA axis function or the neuroendocrine response under stress imposes left-sided effects on hippocampal microstructure (Madsen et al., 2012). This asymmetric role of the bilateral hippocampus in stress may also be due to asymmetries in neuronal numbers and neurogenesis, as well as proteomics and genomics (Hou et al., 2013). Further studies are required to examine the molecular mechanisms underlying such asymmetry.
Our investigation of the dorsal and ventral portions of the hippocampus suggested that the difference in ventral hippocampal microstructure seemed to act as a pre-existing factor in the following behavioral phenotypes, whereas the dHi was more influenced by the stress paradigm and demonstrated adaptive changes. Structural and functional differences in the dHi and vHi have been put forward previously (Fanselow and Dong, 2010), and their specific role under stress has drawn an increasing amount of attention. For example, after chronic unpredictable stress (CUS), a volumetric reduction and increase was revealed in the dHi and vHi, respectively, concurrent with dendritic atrophy and enrichment in the respective hippocampal subregions (Pinto et al., 2015). The vHi is usually believed to be more involved in emotional regulation and the stress response (Trivedi and Coover, 2004; Adami et al., 2006; Pentkowski et al., 2006; Kenworthy et al., 2014) through neurogenesis (O’Leary et al., 2012; Tanti et al., 2012) and epigenetics (Nasca et al., 2017). In the present study, the significantly lower pre-stress FA value also exhibited in the vHi. However, after stress, a greater microstructural alteration was demonstrated in the dHi than in the vHi, especially in the resilient group. Consistent with our findings, an analysis of the change in the number of hippocampal interneurons of specific subtypes after CMS revealed a more pronounced effect on the dHi than vHi (Czeh et al., 2015). Moreover, CUS selectively decreased cell survival in the vHi, while it induced adaptive neuroplasticity primarily in the dHi, which was closely related to the following behavioral responses, such as avoidance or amelioration of the stressor (Hawley and Leasure, 2012). But whether the pre-existed FA differences in the right vHi drive the changes in dHi MD/RD values in response to stress is still need further longitudinal studies based on the specific role of the vHi and dHi both before and after stress.
The present study had some limitations. First, our study lacked a direct observation of neuron and dendrite density in the bilateral hippocampus of stress-susceptible and resilient mice using immunohistological methods, which would be necessary for a more in-depth explanation of the longitudinal changes of diffusion properties. Second, this longitudinal work did not examine other stress-related brain regions. Covariant microstructural changes might be more closely related to the mechanisms underlying stress-related psychiatry or stress resilience. In addition, since the main focus of the current study was to investigate the longitudinal microstructural changes in the bilateral hippocampus in different stress phenotypes, only an ROI-based method was used rather than a voxel-based analysis or tract-based spatial statistics. Third, more hippocampal-dependent function tasks might be added to test whether there is a corresponding change in memory and learning, contextual fear conditioning, or other hippocampus-dependent cognitional or emotional regulations associated with the detected microstructural changes. Moreover, echo-planar imaging (EPI) sequence was chosen over turbo spin echo (TSE) in this study for its common use, less imaging time and motion artifact (Poustchi-Amin et al., 2001), however, taking the image quality into consideration, TSE could be a good alternative (Hirata et al., 2018). Finally, due to the limited sample size, some more subtle differences may have not reached a significant level. In conclusion, this non-invasive in vivo DTI method allowed us to detect subtle microstructural alterations in the bilateral dHi and vHi of different stress phenotypes in a CSDS mouse model. Our data revealed a lower FA value in the right vHi of the susceptible mice than in the right vHi of resilient mice that was present prior to stress, suggesting that pre-existing microstructural abnormalities may result in stress susceptibility. Meanwhile, increases in MD and RD values were found in the right dHi of the resilient subgroup after stress compared to those before stress, which may indicate that plastic changes that prevent individuals from exhibiting social avoidance behavior occur in response to stress. Furthermore, the asymmetric effect imposed by stress highlights the important role of the right hippocampus, which may be the key to understanding the mechanism underlying stress-related disorders as well as become a potential target for resilience training or more effective interventions.
Author Contributions
XL conducted the experiments, analyzed the data, and drafted the manuscript. ZF designed the experiments. JY and YG performed some behavioral tests. YG, XW, and ZF revised the paper.
Funding
This work was supported by grants from the National Natural Science Foundation of China (NSFC 31640036) and Key Project of the Applied Basic Research Programs for Military Mental Health (BWS14J029).
Conflict of Interest Statement
The authors declare that the research was conducted in the absence of any commercial or financial relationships that could be construed as a potential conflict of interest.
Acknowledgments
We gratefully acknowledge the invaluable assistance of Yu Guo (Daping Hospital, China) in MRI scanning, Zhi Liu (Department of Histology and Embryology, Army Medical University, China) in behavioral tests, and Chen Bian (School of Psychology, Army Medical University, China) in mouse hippocampus separation.
Supplementary Material
The Supplementary Material for this article can be found online at: https://www.frontiersin.org/articles/10.3389/fnins.2018.00613/full#supplementary-material
Footnotes
References
Adami, P., Konig, P., Vetter, Z., Hausmann, A., and Conca, A. (2006). Post-traumatic stress disorder and amygdala-hippocampectomy. Acta Psychiatr. Scand. 113, 360–363. doi: 10.1111/j.1600-0447.2005.00737.x
Alexander, A. L., Lee, J. E., Lazar, M., and Field, A. S. (2007). Diffusion tensor imaging of the brain. Neurotherapeutics 4, 316–329. doi: 10.1016/j.nurt.2007.05.011
Alves, N. D., Correia, J. S., Patricio, P., Mateus-Pinheiro, A., Machado-Santos, A. R., Loureiro-Campos, E., et al. (2017). Adult hippocampal neuroplasticity triggers susceptibility to recurrent depression. Transl. Psychiatry 7:e1058. doi: 10.1038/tp.2017.29
Amico, F., Meisenzahl, E., Koutsouleris, N., Reiser, M., Moller, H. J., and Frodl, T. (2011). Structural MRI correlates for vulnerability and resilience to major depressive disorder. J. Psychiatry Neurosci. 36, 15–22. doi: 10.1503/jpn.090186
Anacker, C., Scholz, J., O’Donnell, K. J., Allemang-Grand, R., Diorio, J., Bagot, R. C., et al. (2016). Neuroanatomic Differences Associated With Stress Susceptibility and Resilience. Biol. Psychiatry 79, 840–849. doi: 10.1016/j.biopsych.2015.08.009
Bannerman, D. M., Sprengel, R., Sanderson, D. J., McHugh, S. B., Rawlins, J. N., Monyer, H., et al. (2014). Hippocampal synaptic plasticity, spatial memory and anxiety. Nat. Rev. Neurosci. 15, 181–192. doi: 10.1038/nrn3677
Beppu, T., Inoue, T., Shibata, Y., Yamada, N., Kurose, A., Ogasawara, K., et al. (2005). Fractional anisotropy value by diffusion tensor magnetic resonance imaging as a predictor of cell density and proliferation activity of glioblastomas. Surg. Neurol. 63, 56–61;discussion61. doi: 10.1016/j.surneu.2004.02.034
Carboni, L., Piubelli, C., Pozzato, C., Astner, H., Arban, R., Righetti, P. G., et al. (2006). Proteomic analysis of rat hippocampus after repeated psychosocial stress. Neuroscience 137, 1237–1246. doi: 10.1016/j.neuroscience.2005.10.045
Carlesimo, G. A., Cherubini, A., Caltagirone, C., and Spalletta, G. (2010). Hippocampal mean diffusivity and memory in healthy elderly individuals: a cross-sectional study. Neurology 74, 194–200. doi: 10.1212/WNL.0b013e3181cb3e39
Chan, S. W., Harmer, C. J., Norbury, R., O’Sullivan, U., Goodwin, G. M., and Portella, M. J. (2016). Hippocampal volume in vulnerability and resilience to depression. J. Affect. Disord. 189, 199–202. doi: 10.1016/j.jad.2015.09.021
Charney, D. S. (2004). Psychobiological mechanisms of resilience and vulnerability: implications for successful adaptation to extreme stress. Am. J. Psychiatry 161, 195–216. doi: 10.1176/appi.ajp.161.2.195
Czeh, B., Simon, M., Schmelting, B., Hiemke, C., and Fuchs, E. (2006). Astroglial plasticity in the hippocampus is affected by chronic psychosocial stress and concomitant fluoxetine treatment. Neuropsychopharmacology 31, 1616–1626. doi: 10.1038/sj.npp.1300982
Czeh, B., Varga, Z. K., Henningsen, K., Kovacs, G. L., Miseta, A., and Wiborg, O. (2015). Chronic stress reduces the number of GABAergic interneurons in the adult rat hippocampus, dorsal-ventral and region-specific differences. Hippocampus 25, 393–405. doi: 10.1002/hipo.22382
Delgado y Palacios, R., Campo, A., Henningsen, K., Verhoye, M., Poot, D., Dijkstra, J., et al. (2011). Magnetic resonance imaging and spectroscopy reveal differential hippocampal changes in anhedonic and resilient subtypes of the chronic mild stress rat model. Biol. Psychiatry 70, 449–457. doi: 10.1016/j.biopsych.2011.05.014
Delgado y Palacios, R., Verhoye, M., Henningsen, K., Wiborg, O., and Van der Linden, A. (2014). Diffusion kurtosis imaging and high-resolution MRI demonstrate structural aberrations of Caudate Putamen and Amygdala after chronic mild stress. PLoS One 9:e95077. doi: 10.1371/journal.pone.0095077
Evans, A. C. (2013). Networks of anatomical covariance. Neuroimage 80, 489–504. doi: 10.1016/j.neuroimage.2013.05.054
Fanselow, M. S., and Dong, H. W. (2010). Are the dorsal and ventral hippocampus functionally distinct structures? Neuron 65, 7–19. doi: 10.1016/j.neuron.2009.11.031
Fenton, E. Y., Fournier, N. M., Lussier, A. L., Romay-Tallon, R., Caruncho, H. J., and Kalynchuk, L. E. (2015). Imipramine protects against the deleterious effects of chronic corticosterone on depression-like behavior, hippocampal reelin expression, and neuronal maturation. Prog. Neuropsychopharmacol. Biol. Psychiatry 60, 52–59. doi: 10.1016/j.pnpbp.2015.02.001
Friedman, A. K., Walsh, J. J., Juarez, B., Ku, S. M., Chaudhury, D., Wang, J., et al. (2014). Enhancing depression mechanisms in midbrain dopamine neurons achieves homeostatic resilience. Science 344, 313–319. doi: 10.1126/science.1249240
Frodl, T., Meisenzahl, E. M., Zetzsche, T., Born, C., Groll, C., Jager, M., et al. (2002). Hippocampal changes in patients with a first episode of major depression. Am. J. Psychiatry 159, 1112–1118. doi: 10.1176/appi.ajp.159.7.1112
Frodl, T., and O’Keane, V. (2012). How does the brain deal with cumulative stress? A review with focus on developmental stress, HPA axis function and hippocampal structure in humans. Neurobiol. Dis. 52, 24–37. doi: 10.1016/j.nbd.2012.03.012
Gilabert-Juan, J., Bueno-Fernandez, C., Castillo-Gomez, E., and Nacher, J. (2017). Reduced interneuronal dendritic arborization in CA1 but not in CA3 region of mice subjected to chronic mild stress. Brain Behav. 7:e00534. doi: 10.1002/brb3.534
Golden, S. A., Covington, H. E. III, Berton, O., and Russo, S. J. (2011). A standardized protocol for repeated social defeat stress in mice. Nat. Protoc. 6, 1183–1191. doi: 10.1038/nprot.2011.361
Hawley, D. F., and Leasure, J. L. (2012). Region-specific response of the hippocampus to chronic unpredictable stress. Hippocampus 22, 1338–1349. doi: 10.1002/hipo.20970
Heim, C., Newport, D. J., Mletzko, T., Miller, A. H., and Nemeroff, C. B. (2008). The link between childhood trauma and depression: insights from HPA axis studies in humans. Psychoneuroendocrinology 33, 693–710. doi: 10.1016/j.psyneuen.2008.03.008
Hirata, K., Nakaura, T., Okuaki, T., Kidoh, M., Oda, S., Utsunomiya, D., et al. (2018). Comparison of the image quality of turbo spin echo- and echo-planar diffusion-weighted images of the oral cavity. Medicine 97:e0447. doi: 10.1097/MD.0000000000010447
Hou, G., Yang, X., and Yuan, T. F. (2013). Hippocampal asymmetry: differences in structures and functions. Neurochem. Res. 38, 453–460. doi: 10.1007/s11064-012-0954-3
Hunter, S. C., Durkin, K., Heim, D., Howe, C., and Bergin, D. (2010). Psychosocial mediators and moderators of the effect of peer-victimization upon depressive symptomatology. J. Child Psychol. Psychiatry 51, 1141–1149. doi: 10.1111/j.1469-7610.2010.02253.x
Kenworthy, C. A., Sengupta, A., Luz, S. M., Ver Hoeve, E. S., Meda, K., Bhatnagar, S., et al. (2014). Social defeat induces changes in histone acetylation and expression of histone modifying enzymes in the ventral hippocampus, prefrontal cortex, and dorsal raphe nucleus. Neuroscience 264, 88–98. doi: 10.1016/j.neuroscience.2013.01.024
Khan, A. R., Chuhutin, A., Wiborg, O., Kroenke, C. D., Nyengaard, J. R., Hansen, B., et al. (2016). Biophysical modeling of high field diffusion MRI demonstrates micro-structural aberration in chronic mild stress rat brain. Neuroimage 142, 421–430. doi: 10.1016/j.neuroimage.2016.07.001
Kheirbek, M. A., and Hen, R. (2011). Dorsal vs ventral hippocampal neurogenesis: implications for cognition and mood. Neuropsychopharmacology 36, 373–374. doi: 10.1038/npp.2010.148
Kinoshita, M., Hashimoto, N., Goto, T., Kagawa, N., Kishima, H., Izumoto, S., et al. (2008). Fractional anisotropy and tumor cell density of the tumor core show positive correlation in diffusion tensor magnetic resonance imaging of malignant brain tumors. Neuroimage 43, 29–35. doi: 10.1016/j.neuroimage.2008.06.041
Kleinknecht, K. R., Bedenk, B. T., Kaltwasser, S. F., Grunecker, B., Yen, Y. C., Czisch, M., et al. (2012). Hippocampus-dependent place learning enables spatial flexibility in C57BL6/N mice. Front. Behav. Neurosci. 6:87. doi: 10.3389/fnbeh.2012.00087
Krishnan, V., Han, M.-H., Graham, D. L., Berton, O., Renthal, W., Russo, S. J., et al. (2007). Molecular adaptations underlying susceptibility and resistance to social defeat in brain reward regions. Cell 131, 391–404. doi: 10.1016/j.cell.2007.09.018
Krystal, J. H., and Duman, R. (2004). What’s missing in posttraumatic stress disorder research? Studies of human postmortem tissue. Psychiatry 67, 398–403. doi: 10.1521/psyc.67.4.398.56567
Kumar, B. S. H., Mishra, S. K., Trivedi, R., Singh, S., Rana, P., and Khushu, S. (2014). Demyelinating evidences in CMS rat model of depression: a DTI study at 7 T. Neuroscience. 275, 12–21. doi: 10.1016/j.neuroscience.2014.05.037
Lagace, D. C., Donovan, M. H., DeCarolis, N. A., Farnbauch, L. A., Malhotra, S., Berton, O., et al. (2010). Adult hippocampal neurogenesis is functionally important for stress-induced social avoidance. Proc. Natl. Acad. Sci. U.S.A. 107, 4436–4441. doi: 10.1073/pnas.0910072107
Lein, E. S., Hawrylycz, M. J., Ao, N., Ayres, M., Bensinger, A., Bernard, A., et al. (2007). Genome-wide atlas of gene expression in the adult mouse brain. Nature 445, 168–176. doi: 10.1038/nature05453
Li, N., Liu, R. J., Dwyer, J. M., Banasr, M., Lee, B., Son, H., et al. (2011). Glutamate N-methyl-D-aspartate receptor antagonists rapidly reverse behavioral and synaptic deficits caused by chronic stress exposure. Biol. Psychiatry 69, 754–761. doi: 10.1016/j.biopsych.2010.12.015
Li, Y., Wang, H., Wang, X., Liu, Z., Wan, Q., and Wang, G. (2014). Differential expression of hippocampal EphA4 and ephrinA3 in anhedonic-like behavior, stress resilience, and antidepressant drug treatment after chronic unpredicted mild stress. Neurosci. Lett. 566, 292–297. doi: 10.1016/j.neulet.2014.03.008
Londono, A., Castillo, M., Lee, Y. Z., and Smith, J. K. (2003). Apparent diffusion coefficient measurements in the hippocampi in patients with temporal lobe seizures. AJNR Am. J. Neuroradiol. 24, 1582–1586.
Madsen, K. S., Jernigan, T. L., Iversen, P., Frokjaer, V. G., Knudsen, G. M., Siebner, H. R., et al. (2012). Hypothalamic–pituitary–adrenal axis tonus is associated with hippocampal microstructural asymmetry. Neuroimage 63, 95–103. doi: 10.1016/j.neuroimage.2012.06.071
Malykhin, N. V., Carter, R., Seres, P., and Coupland, N. J. (2010). Structural changes in the hippocampus in major depressive disorder: contributions of disease and treatment. J. Psychiatry Neurosci. 35, 337–343. doi: 10.1503/jpn.100002
McEwen, B. S., Gray, J., and Nasca, C. (2015). Recognizing resilience: learning from the Effects of Stress on the Brain. Neurobiol. Stress 1, 1–11. doi: 10.1016/j.ynstr.2014.09.001
Morey, R. A., Haswell, C. C., Hooper, S. R., and De Bellis, M. D. (2016). Amygdala, hippocampus, and ventral medial prefrontal cortex volumes differ in maltreated youth with and without chronic posttraumatic stress disorder. Neuropsychopharmacology 41, 791–801. doi: 10.1038/npp.2015.205
Muscatell, K. A., Slavich, G. M., Monroe, S. M., and Gotlib, I. H. (2009). Stressful life events, chronic difficulties, and the symptoms of clinical depression. J. Nerv. Ment. Dis. 197, 154–160. doi: 10.1097/NMD.0b013e318199f77b
Nasca, C., Bigio, B., Zelli, D., de Angelis, P., Lau, T., Okamoto, M., et al. (2017). Role of the astroglial glutamate exchanger xCT in ventral hippocampus in resilience to stress. Neuron 96:e405. doi: 10.1016/j.neuron.2017.09.020
Nestler, E. J., and Hyman, S. E. (2010). Animal models of neuropsychiatric disorders. Nat. Neurosci. 13, 1161–1169. doi: 10.1038/nn.2647
O’Doherty, D. C., Chitty, K. M., Saddiqui, S., Bennett, M. R., and Lagopoulos, J. (2015). A systematic review and meta-analysis of magnetic resonance imaging measurement of structural volumes in posttraumatic stress disorder. Psychiatry Res. 232, 1–33. doi: 10.1016/j.pscychresns.2015.01.002
O’Leary, O. F., and Cryan, J. F. (2014). A ventral view on antidepressant action: roles for adult hippocampal neurogenesis along the dorsoventral axis. Trends Pharmacol Sci. 35, 675–687. doi: 10.1016/j.tips.2014.09.011
O’Leary, O. F., O’Connor, R. M., and Cryan, J. F. (2012). Lithium-induced effects on adult hippocampal neurogenesis are topographically segregated along the dorso-ventral axis of stressed mice. Neuropharmacology 62, 247–255. doi: 10.1016/j.neuropharm.2011.07.015
Otten, M., and Meeter, M. (2015). Hippocampal structure and function in individuals with bipolar disorder: a systematic review. J. Affect. Disord. 174, 113–125. doi: 10.1016/j.jad.2014.11.001
Paxinos, G., and Franklin, K. (2001). The Mouse Brain in Stereotaxic Coordinates. San Diego, CA: Acadamic Press.
Pentkowski, N. S., Blanchard, D. C., Lever, C., Litvin, Y., and Blanchard, R. J. (2006). Effects of lesions to the dorsal and ventral hippocampus on defensive behaviors in rats. Eur. J. Neurosci. 23, 2185–2196. doi: 10.1111/j.1460-9568.2006.04754.x
Pinto, V., Costa, J. C., Morgado, P., Mota, C., Miranda, A., Bravo, F. V., et al. (2015). Differential impact of chronic stress along the hippocampal dorsal-ventral axis. Brain Struct. Funct. 220, 1205–1212. doi: 10.1007/s00429-014-0713-0
Piras, F., Cherubini, A., Caltagirone, C., and Spalletta, G. (2011). Education mediates microstructural changes in bilateral hippocampus. Hum. Brain Mapp. 32, 282–289. doi: 10.1002/hbm.21018
Pizzagalli, D. A. (2014). Depression, stress, and anhedonia: toward a synthesis and integrated model. Annu. Rev. Clin. Psychol. 10, 393–423. doi: 10.1146/annurev-clinpsy-050212-185606
Poustchi-Amin, M., Mirowitz, S. A., Brown, J. J., McKinstry, R. C., and Li, T. (2001). Principles and applications of echo-planar imaging: a review for the general radiologist. Radiographics. 21, 767–779. doi: 10.1148/radiographics.21.3.g01ma23767
Reichel, J. M., Bedenk, B. T., Czisch, M., and Wotjak, C. T. (2017). Age-related cognitive decline coincides with accelerated volume loss of the dorsal but not ventral hippocampus in mice. Hippocampus 27, 28–35. doi: 10.1002/hipo.22668
Russo, S. J., Murrough, J. W., Han, M. H., Charney, D. S., and Nestler, E. J. (2012). Neurobiology of resilience. Nat. Neurosci. 15, 1475–1484. doi: 10.1038/nn.3234
Sheline, Y. I. (2011). Depression and the hippocampus: cause or effect? Biol Psychiatry 70, 308–309. doi: 10.1016/j.biopsych.2011.06.006
Smith, M. E. (2005). Bilateral hippocampal volume reduction in adults with post-traumatic stress disorder: a meta-analysis of structural MRI studies. Hippocampus 15, 798–807. doi: 10.1002/hipo.20102
Song, S.-K., Sun, S.-W., Ramsbottom, M. J., Chang, C., Russell, J., and Cross, A. H. (2002). Dysmyelination Revealed through MRI as Increased Radial (but Unchanged Axial) Diffusion of Water. Neuroimage 17, 1429–1436. doi: 10.1006/nimg.2002.1267
Srivastava, S., Bhatia, M. S., Bhargava, S. K., Kumari, R., and Chandra, S. (2016). A Diffusion Tensor Imaging Study Using a Voxel-Based Analysis, Region-of-Interest Method to Analyze White Matter Abnormalities in First-Episode, Treatment-Naive Major Depressive Disorder. J. Neuropsychiatry Clin. Neurosci. 28, 131–137. doi: 10.1176/appi.neuropsych.15050120
Stelzhammer, V., Ozcan, S., Gottschalk, M. G., Steeb, H., Hodes, G. E., Guest, P. C., et al. (2015). Central and peripheral changes underlying susceptibility and resistance to social defeat stress – A proteomic profiling study. Diag. Neuropsychiatry 1, 1–7. doi: 10.1016/j.dineu.2015.08.001
Stockmeier, C. A., Mahajan, G. J., Konick, L. C., Overholser, J. C., Jurjus, G. J., Meltzer, H. Y., et al. (2004). Cellular changes in the postmortem hippocampus in major depression. Biol. Psychiatry 56, 640–650. doi: 10.1016/j.biopsych.2004.08.022
Tang, A. C., Zou, B., Reeb, B. C., and Connor, J. A. (2008). An epigenetic induction of a right-shift in hippocampal asymmetry: selectivity for short- and long-term potentiation but not post-tetanic potentiation. Hippocampus 18, 5–10. doi: 10.1002/hipo.20370
Tanti, A., Rainer, Q., Minier, F., Surget, A., and Belzung, C. (2012). Differential environmental regulation of neurogenesis along the septo-temporal axis of the hippocampus. Neuropharmacology. 63, 374–384. doi: 10.1016/j.neuropharm.2012.04.022
Trivedi, M. A., and Coover, G. D. (2004). Lesions of the ventral hippocampus, but not the dorsal hippocampus, impair conditioned fear expression and inhibitory avoidance on the elevated T-maze. Neurobiol. Learn. Mem. 81, 172–184. doi: 10.1016/j.nlm.2004.02.005
Tse, Y. C., Montoya, I., Wong, A. S., Mathieu, A., Lissemore, J., Lagace, D. C., et al. (2014). A longitudinal study of stress-induced hippocampal volume changes in mice that are susceptible or resilient to chronic social defeat. Hippocampus 24, 1120–1128. doi: 10.1002/hipo.22296
Waltzman, D., Soman, S., Hantke, N. C., Fairchild, J. K., Kinoshita, L. M., Wintermark, M., et al. (2017). Altered microstructural caudate integrity in posttraumatic stress disorder but not traumatic brain injury. PLoS One 12:e0170564. doi: 10.1371/journal.pone.0170564
Yang, C., Shirayama, Y., Zhang, J. C., Ren, Q., and Hashimoto, K. (2015). Regional differences in brain-derived neurotrophic factor levels and dendritic spine density confer resilience to inescapable stress. Int. J. Neuropsychopharmacol. 18:yu121. doi: 10.1093/ijnp/pyu121
Zach, P., Mrzilkova, J., Rezacova, L., Stuchlik, A., and Valeš, K. (2010). Delayed Effects of Elevated Corticosterone Level on Volume of Hippocampal Formation in Laboratory Rat. Physiol. Res. 59, 985–996.
Zach, P., Vales, K., Stuchlik, A., Cermakova, P., Mrzilkova, J., Koutela, A., et al. (2016). Effect of stress on structural brain asymmetry. Neuro Endocrinol. Lett. 37, 253–264.
Keywords: chronic social defeat stress, susceptibility, resilience, dorsoventral hippocampus, diffusion tensor imaging (DTI), longitudinal
Citation: Liu X, Yuan J, Guang Y, Wang X and Feng Z (2018) Longitudinal in vivo Diffusion Tensor Imaging Detects Differential Microstructural Alterations in the Hippocampus of Chronic Social Defeat Stress-Susceptible and Resilient Mice. Front. Neurosci. 12:613. doi: 10.3389/fnins.2018.00613
Received: 07 March 2018; Accepted: 13 August 2018;
Published: 29 August 2018.
Edited by:
Pablo Blinder, Tel Aviv University, IsraelReviewed by:
Boldizsar Czeh, University of Pécs, HungaryJames P. Kesby, University of Queensland, Australia
Rafael Delgado Y. Palacios, Federal Agency for Medicines and Health Products (FAMHP), Belgium
Copyright © 2018 Liu, Yuan, Guang, Wang and Feng. This is an open-access article distributed under the terms of the Creative Commons Attribution License (CC BY). The use, distribution or reproduction in other forums is permitted, provided the original author(s) and the copyright owner(s) are credited and that the original publication in this journal is cited, in accordance with accepted academic practice. No use, distribution or reproduction is permitted which does not comply with these terms.
*Correspondence: Zhengzhi Feng, fzz@tmmu.edu.cn