Supplementing Ruminally Protected Lysine, Methionine, or Combination Improved Milk Production in Transition Dairy Cows
- 1State Key Laboratory of Animal Nutrition, Institute of Animal Sciences, Chinese Academy of Agricultural Sciences, Beijing, China
- 2Department of Dairy Science, Virginia Tech, Blacksburg, VA, United States
- 3Joint Laboratory on Integrated Crop-Tree-Livestock Systems of the Chinese Academy of Agricultural Sciences (CAAS), Ethiopian Institute of Agricultural Research (EIAR) and World Agroforestry Centre (ICRAF), Beijing, China
- 4Hunan Co-Innovation Center of Safety Animal Production, Changsha, China
The objectives of this study were to evaluate the effects of dietary supplementation of ruminally protected lysine (RPL), or methionine (RPM), and their combination (RPML) on the production efficiency of transition cows. A total of 120 pre-partum multiparous Holstein cows were assigned to four treatments based on previous lactation milk production, days (d) of pregnancy, lactation, and body condition score (BCS). Cows were fed a basal diet [pre-calving: 1.53 Mcal/kg dry matter (DM) and post-calving: 1.70 Mcal/kg DM] with or without supplemental ruminally protected amino acids (RPAA). Treatments were the basal diets without supplemental amino acids (CONTROL, n = 30), with supplemental methionine (RPM, pre-calving at 0.16% of DM and post-calving at 0.12% of DM, n = 30), with supplemental lysine (RPL, pre-calving at 0.33% of DM and post-calving at 0.24% DM, n = 30), and the combination (RPML, pre-calving at 0.16% RPM + 0.33% RPL of DM and post-calving at 0.12% RPM + 0.24 % RPL DM, n = 30). The dietary content of lysine was balanced to be within 6.157.2% metabolizable protein (MP)–lysine and that of methionine was balanced within 2.1–2.35% MP-methionine. Dry matter intake (DMI) was measured daily. Milk samples were taken on d 7, 14, and 21 days relative to calving (DRC), and milk yields were measured daily. Blood samples were taken on d −21, −14, −7 before expected calving and d 0, 7, 14, and 21 DRC. Data were analyzed using SAS software. There were significant Trt × time interactions (P < 0.01) for DMI pre- and post-calving period. The CON cows had lower DMI than RPM, RPL, and RPML, both pre-calving (P < 0.01) and post-calving periods (P < 0.01). Energy-corrected milk (P < 0.01), milk fat (P < 0.01), protein (P = 0.02), and lactose (P < 0.01) percentage levels were greater for RPM, RPL, and RPML cows compared to CON. Supplementing RPAA assisted in maintaining BCS post-calving than CON (P < 0.01). Blood concentrations of β-hydroxybutyrate decreased with RPM or RPL or the combination pre-calving (P < 0.01) and tended to decrease post-calving (P = 0.10). These results demonstrated that feeding RPL and RPM improved DMI and milk production efficiency, maintained BCS, and reduced β-hydroxybutyrate concentrations of transition cows.
Introduction
Dairy cows undergo significant metabolic, physiological, and immunological changes during the transition period, which, if not managed correctly, will increase the occurrence of metabolic disorders during early lactation (1). Peri-parturient cows mobilize body fat to meet their energy requirements (2), and to meet protein or amino acid (AA) requirements as well, because dry matter intake (DMI) is insufficient to meet the cow's requirements. The negative balance of energy and AAs is the main reason for many metabolic diseases and problems, and its consequences cause reduced milk production, lower fertility, increased health disorders, and decreased animal efficiency in the long term.
Improving the AA profile for milk protein synthesis via dietary AA supplementation can alleviate intensive AA mobilization in dairy cows after parturition, thus sparing body protein reserves. Methionine (Met), lysine (Lys), and histidine (His) are generally considered limiting AAs in diets for high-producing cows (3–7). Metabolism of Met in the liver is very important; for instance, one-carbon metabolism has many roles in controlling the immunometabolic and growth during pregnancy, lactation, and the neonatal period in dairy cattle (8). Met is a source of the methyl donor S-adenosyl Met (9). Probably, the most important metabolic role of Met is that of a hepatic lipotropic agent promoting synthesis of very low–density lipoproteins (VLDL), which minimizes the accumulation of triacylglycerol (TAG) in the liver (10, 11). Carnitine is synthesized from Lys (carbon backbone) and Met (methyl group donor) (12), which is required for the oxidation of long-chain fatty acids (LCFAs) and the regulation of ketosis.
Transition dairy cows fed ruminally protected Lys (RPL) and ruminally protected Met (RPM) during both the pre- and post-calving periods had increased milk and milk protein production (13, 14), but this response is not universal (15). Pre- and post-calving supplementation of only RPM may be sufficient to elicit the response (16, 17). The inclusion rate of AAs in diets is varied. As an example, Lee et al. (15) supplied a combination of RPL and RPM to transition dairy cows either before calving (0.14% RPM + 0.24% RPL of DM) or post-calving (0.22% RPM + 0.36% RPL of DM) or throughout the peri-parturient period (15). Others fed 15 g/d of RPM to post-calving cows (14). Fehlberg et al. (18) supplied 0.54% and 0.40% of DM RPL during pre- and post-calving, respectively, to transition cows (19). Studies on beef cows supplied RPM (10 g/d to provide a 3.7 g/d of absorbed Met) (20) or at a 9 g/d (18). Therefore, in the current study, the dietary supplementation of RPL was balanced to have Lys within 6.15–7.2% metabolizable protein (MP)–Lys and Met to within 2.1–2.35% MP-Met.
Previous studies have been conducted to examine supplementation of one ruminally protected AA (RPAA) (in most cases, but not all) to low or medium energy transition diets, generally either in the pre-or post-partum periods, with only a few studies supplementing throughout the peri-parturient period (14, 15, 19, 21, 22). To our knowledge, no studies have been conducted to examine the effects of supplying a combination of RPAA to high-energy diets of transition cows. It was hypothesized that continual supply of either RPM or RPL to high energy diets fed to transition dairy cows would stimulate DMI, driven by the improvement of AA balance, leading to improve energy and MP balance and increased milk production. We also hypothesized that the improvement of lactation performance and health would be greatest when cows have consumed the combination of RPL and RPM. The objectives of this study were to assess the effects of the supplementation of RPL or RPM and the combination of high energy density diets on production efficiency and BHB concentrations of transition dairy cows.
The results have been presented in partial form during the 2021 Annual Meeting of American Dairy Science Association (ADSA), Abstract No. 245 “Supplementing ruminally protected methionine or lysine improved milk production in transition cows” (23, 24).
Materials and Methods
Experimental Design and Animals
The trial was conducted from December 2019 to March 2020 at a mega-dairy with a total capacity of 10,000 milking cows, AustAsia Dairy, Shandong, China. A total of 120 Holstein dairy cows were randomly allocated to eight groups [replicates (15 cows per each replicate)], and two replicates were assigned to each of the four experimental treatments (n = 30 cows per treatment): (1) CON cows received the basal diet without supplemental RPAA; (2) RPM received CON plus RPM; (3) RPL received CON plus RPL; and (4) RPML received CON plus RPM and RPL. RPM, RPL, and RPML all received the same amount of supplemental RPAAs.
A statistical power analysis was conducted before the trial using a two-tailed α of 0.05, a power of 0.95, and an expected effect size of 0.35 using G–Power 3.1 software (25). At that power, 25 cows per treatment were required in order to detect a minimum of about 6% difference in post-calving DMI between treatment groups. The experiment was conducted as a completely randomized design with treatments arranged in a 2 × 2 factorial. Cows were selected and assigned to treatment based on days (d) pregnant (250 ± 2 d, P = 0.84), previous lactation milk production (11,512 ± 1,837 kg; 305-d milk production, P = 0.90), parity (3.09 ± 1.56, P = 0.94), and body condition score (BCS; 3.58 ± 0.26, P = 0.86).
The cows were fed their respective diets starting 3 weeks (wk) (25.0 ± 3.31 d) before the expected calving to 3 wk post-calving (24.0 ± 3.31 d). Cows were fed total mixed rations (TMR) ad libitum four times per day at 0600, 1,200, 1,800, and 2,400 h and milked in the rotary milking parlor four times per day just before being fed, at ~6-h intervals. Feed offered was managed to achieve a target of 5% refusals. The composition, chemical analyses, and AA profiles of the diets are presented in (Tables 1–3).
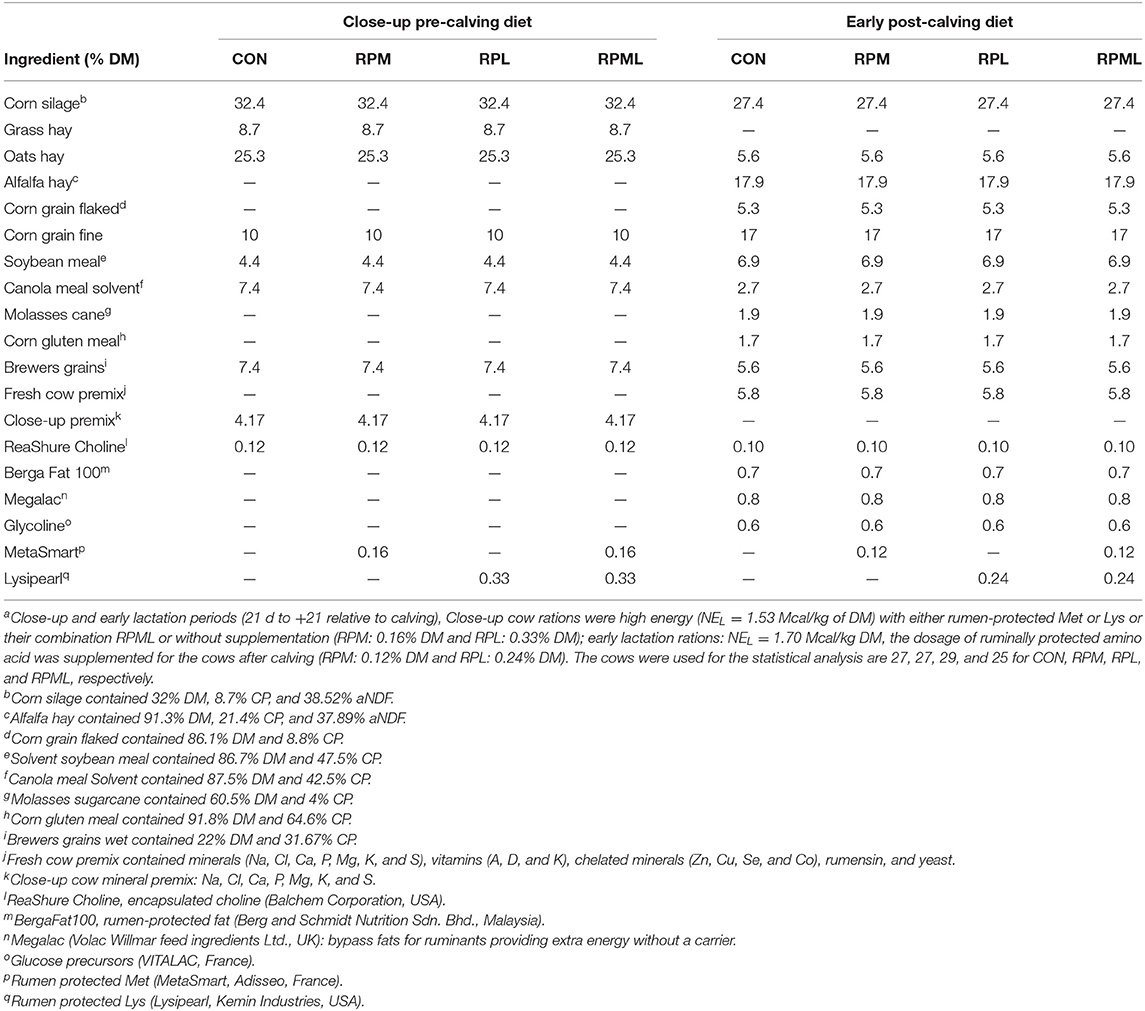
Table 1. Ingredient composition of diets fed to Holstein cows during the transition period [close-up pre-calving diets (the last 3 wk before calving) to the early post-calving (the first 3 wk after calving)]a.
During the close-up and post-calving periods, cows were housed in a ventilated, four-row, free-stall barn (center feed alley with two rows of stalls on each side). After calving, cows were moved to the colostrum barn for 1 to 3 d, followed by relocation to the milking barn. Manure was removed by mechanical scraper four times per day at 0600, 1,200, 1,800, and 2,400 h. Sand bedding was groomed four times/d, and new sand was added two times/d at 0600 and 1,200 h. Cows had access to ad libitum water during the trial.
Temperature and humidity were measured during the pre-calving and post-calving periods (Humidity Detector PCE-HT 112, PCE Instruments Ltd., UK), and the temperature–humidity index (THI) was calculated according to the National Research Council (26).
where Tdb-dry bulb temperature (°C) and RH - relative humidity(%).
Ration Formulation
The isopropyl ester of 2-hydroxy-4-(methylthio)-butanoic acid (HMBi) was supplied in a dry powder form (MetaSmart, Adisseo, France). According to the manufacturer, the product contains 57% HMBi, with a Met equivalence of 78% and a ruminal absorption of 50%. Thus, each gram of the product provided 0.22 g of metabolizable Met. The RPL was provided as a dry powder containing 47.5% L-Lys monohydrochloride (3.2.3) with 70% bioavailability, according to the manufacturer. Thus, each gram of the product provided 0.33 g of metabolizable Lys-HCl (LysiPEARL, Kemin Industries, USA).
Rations were formulated using Cornell Net Carbohydrate and Protein System (CNCPS v. 6.5.5) as executed by AMTS.Cattle.Professional v. 4.7.2 (2016, AMTS LLC, USA) to meet or exceed requirements (Table 1). The dietary content of Met and Lys were balanced according to recommendations of CNCPS v6.5; for Lys to be within 6.15 to 7.2% of MP and Met within 2.1 to 2.35% of dietary MP—to achieve a Lys-to-Met ratio in the range of 2.5:1–3:1 for dairy cows. To achieve that, RPL was provided at rates of 0.33 and 0.24% of DM during the pre- and post-calving, respectively. Met was supplied during the close-up and post-calving periods at rates of 0.16 and 0.12% of DM, respectively (Tables 1–3). The close-up diet was formulated for close-up cows weighing 680 kg [body weight (BW)], with BCS of 3.5 and a predicted intake of 12.8 kg DM/d. The milking cow diet was formulated for fresh cows at 15 d in milk (DIM), weighing 608 kg, with BCS of 3.5, producing 33 kg milk/d with 3.4% crude protein (CP) and 4.2% milk fat, using a predicted DMI of 17.2 kg/d, and using the nutrition evaluation programs CNCPS.
RPAA was mixed with the feed additive premix using a mixer and then top-dressed on the TMR and mixed into the TMR four times/d during the trial using a Vertical Feed Mixer (Supreme International Limited, USA). A Premix is a blend of micronutrients where each nutrient component is prescaled and precision blended into a premix. Premixes include ingredients like vitamins, minerals, nucleotides, AAs, and other functional ingredients. Premix composition is detailed in Table 1.
Sampling, Measurements, and Analysis
Feed and Physically Effective NDF
Feed offered and refused were measured daily for each treatment group. Diets and the major dietary ingredients (i.e., corn silage, alfalfa hay, corn grain, and soybean meal) were sampled and analyzed weekly and used to calculate nutrient concentrations. Feed samples were dried at 105°C for 4 h to determine the DM and stored at −20°C for further analysis. The dried feed samples were ground through a 1-mm screen before analysis using a Cyclotec 1093 Mill (Tecator 1093, Tecator AB, Höganäs, Sweden). Feed samples were further dried at 105°C for 2 h to determine the absolute DM. Chemical analyses of dry matter (DM), CP, ether extract (EE), neutral detergent fiber (aNDF), and acid detergent fiber (ADF) were performed using wet chemistry techniques at the State Key Laboratory of Animal Nutrition, Institute of Animal Sciences of Chinese Academy of Agricultural Sciences (CAAS), Beijing. China.
The content of CP (N × 6.25) in feed samples was analyzed using the macro-Kjeldahl nitrogen test (27) (method 984.13.4.09) with a Kjeltec digester 20 and a Kjeltec System 1026 distilling unit (Tecator AB). EE content was analyzed using a soxhlet HT6 apparatus (Tecator AB) according to method no. 920.39 (27). ADF and aNDF were analyzed according to (28) using alpha-amylase with the addition of sodium sulfite. Ash content was analyzed by incineration at 550°C, and the organic matter content was calculated by subtracting ash from 100. Non-fiber carbohydrates (NFCs) were calculated by difference according to the National Research Council (3).
Energy balance (EB), dietary energy density, and MP balance were calculated based on the actual consumed DMI per each treatment group of individual ingredients and their respective energy value and MP estimates from the CNCPS feed library.
Requirements for NE were calculated as follows:
The net energy of lactation (NEL, Mcal/d) was calculated according to the following:
The net energy requirement for pregnancy (NEY; Mcal/d) was calculated as
NEY = [(0.00318 × day of gestation – 0.0352) × (calf birth weight/45)]/0.218 for animals that were greater than 250 d pregnant, i.e., the pre-fresh group.
The sum of individual requirements was as follows:
EB (Mcal/d) was calculated as follows:
Energy-corrected milk (ECM; 3.5% fat) and fat corrected milk (FCM) were calculated according to (29):
Physically effective NDF (peNDF) of the TMR was determined weekly using a Penn State Particle Separator with three sieves: upper (19-mm pore size), middle (8-mm pore size), lower (4-mm pore size); it also has a pan. The peNDF content (DM bases) of the TMR was estimated by multiplying the NDF content of the feed by the percent of feed retained on each sieve (30).
Blood Samples and Analysis
Blood samples of ~5 ml were collected via coccygeal vessel from individual cows at 0700 h on d −21 (± 3.31 d), −14 (± 3.31 d), and −7 (± 3.31 d) before expected calving and d 0, 7 ± 3.31, 14 ± 3.31, and 21 ± 3.31 d post-calving. Blood β-hydroxybutyrate (BHBA)AQQ31 concentrations were measured immediately after collection of whole blood using the BHBCheck Plus (PortaCheck Inc., Moorestown, NJ, USA).
Milk Samples and Analysis
Milk samples were collected each milking session from individual cows on 7 ± 3.31, 14 ± 3.31, and 21 ± 3.31 DIM. Milk samples from each milking session from each cow were mixed and combined to make a representative milk sample (morning, afternoon, evening, and night; volume ratio of 3:3:2:2); each milk sample was preserved with bronopol-B2 preservative (D&F Control Systems Inc., Dublin, ON, Canada) and stored at 4°C with subsequent analysis for fat, protein, lactose, total solids (TSs), and milk urea nitrogen (MUN) by mid-infrared analysis (MilkoScan FT3; Foss-600, Foss Analytics, Hillerød, Denmark). Somatic cell count (SCC) was analyzed using a Somatic Cells Analyzer (Foss, Hillerød, Denmark). Daily milk production for individual cows was automatically recorded using parlor milk flow sensors, and data were transferred to the dairy herd management software [Delpro Software v5.4 (DeLaval Corporation, Sweden)]. Daily data were then summarized as average weekly data points for statistical analysis. Somatic cell score (SCS) was calculated by transforming SCC using the following equation (31):
Milk N: The Kjeldahl method was used to measures the amount of nitrogen (N) in a milk sample. Milk protein contains ~15.65% N. Therefore, the milk protein concentration is calculated by multiplying the Kjeldahl N concentration by 6.38 (100 ÷ 15.65).
Body Condition Scoring
BCSs were assessed for individual cows once per week by the same two trained professionals from d 21 before expected calving until 3 wk post-calving. BCS was assessed using a five-point scale: 1 = extremely thin and 5 = extremely fat (3). Body condition scoring is a practical method of evaluating body energy stores in dairy cows. The BCS system included a combination of both visual appraisal and manual palpation to score individual cows. BCS evaluation is based on the visual appraisal of many-body points, commonly, between pins and hooks, transverse process, hooks, between the hooks, and tailhead to pins (3).
Calculations and Statistical Analyses
One cow was excluded for calving too early at 265 d of pregnancy (RPML, n = 1). One cow was excluded from pre-calving due to vagal paralysis (RPML, n = 1). Three cows were excluded due to twins (CON, n = 1; PRM, n = 1; RPML, n = 1). The data were removed from 1 cow due to lameness problems (RPML, n = 1). Cows were excluded due to fracture (RPML, n = 1), milk fever (CON, n = 1), uterine prolapse (RPM, n = 1), or digestive problems (CON, n = 1; PRM, n = 1; RPL, n = 1). Therefore, the data for these cows were excluded from the statistical analysis. The cows were used for the statistical analysis are 27, 27, 29, and 25 for CON, RPM, RPL, and RPML, respectively.
Pre-calving and post-calving data were analyzed separately using a model containing the main effects of Met, Lys, and time and their interaction using PROC MIXED of SAS (v9.4, SAS Institute Inc., USA, 2013). The statistical model is as follows:
where Yijkl was the dependent, continuous variable; μ was the overall mean; Li was the fixed effect of Lys; Mj was the fixed effect of Met; LMij was the interaction effect of Lys and Met; Aijk was the random effect of the kth cow within the ijth treatment (combination of Lys and Met); Tl was the repeated, fixed effect of time (day); LTil was the interaction effect of time and Lys; MTjl was the interaction effect of time and Met; LMTijl was the interaction effect of Lys, Met, and time; and εijkl was the residual error. Concentrations of β-hydroxybutyrate, milk yield, and composition were analyzed at different time points, and the covariance structure for the repeated measurements was modeled using the spatial power option. Computing the denominator degrees of freedom using the Kenward–Roger method (32) assessed the distribution of the residuals to determine normality and homoscedasticity. A log-transformation was used for the milk SCC variable to enhance the homogeneity of the distribution of residuals. The least-squares means were compared using the least significant difference, and statistical differences were declared significant at P ≤ 0.05. A tendency was considered at P > 0.05 to P ≤ 0.10.
Results
Barn Characteristics, Feed Particle Size
The average THI was 54.0 ± 4.0 for the close-up barn (min 41.8 and max 69.8) and 57.2 ± 3.9 for the fresh cow barn (min 43.3 and max 70.8). Particle size was as follows: 11.6 ± 0.73% of the pre-calving TMR DM was retained on the upper sieve; 38.4 ± 1.39% on the middle sieve, 17.9 ± 1.3% on the lower sieve, and 33.2 ± 1.5% on the bottom pan were collected. A 9.7 ± 0.70% of the post-calving TMR DM was retained on the upper; 39.9 ±1.40% on the middle sieve, 19.5± 1.28% on the lower sieve, and 31.1± 1.6% on the bottom pan were collected.
Metabolizable Protein Balance
MP balance was positive in the pre-calving period for all treatments, but post-calving MP balances were negative across all dietary treatments. Diets supplied with RPM had greater MP supplies than other treatments during the close-up period supplying an average of 296, 79, and 47 g/d more MP than CON, RPL, and RPML diets, respectively (Table 2). Post-calving cows fed the CON diet had lower MP (656, 237, and 295 g/d) than RPML, RPM, and RPL cows, respectively (Table 2). The RPM and RPML diets provided ca. 0.20% units more Met to reach 2.59% of MP and provided 0.29% units more Lys to reach up to 7.0% of MP.
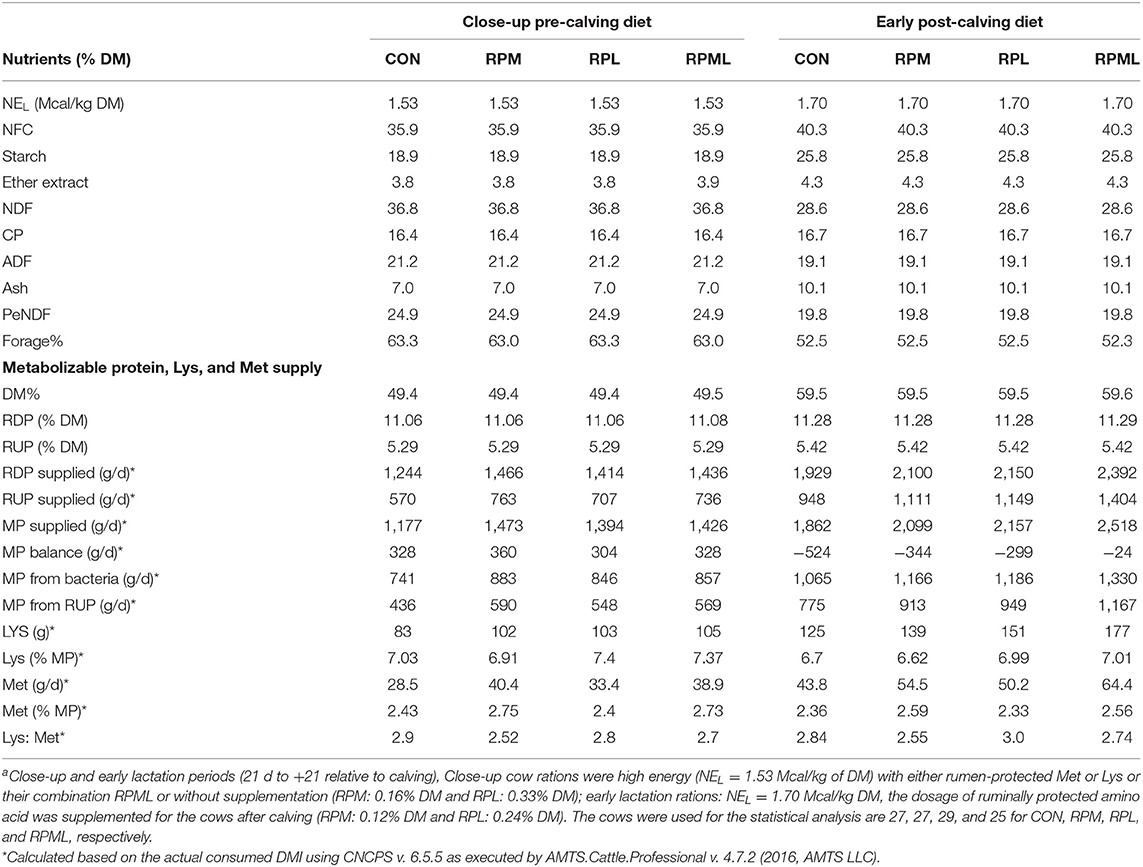
Table 2. Chemical composition and predicted metabolizable protein, Lys, and Met supplies of the diets fed to Holstein cows during the transition period [close-up pre-calving diets (the last 3 wk before calving) to the early post-calving (the first 3 wk after calving)]a.
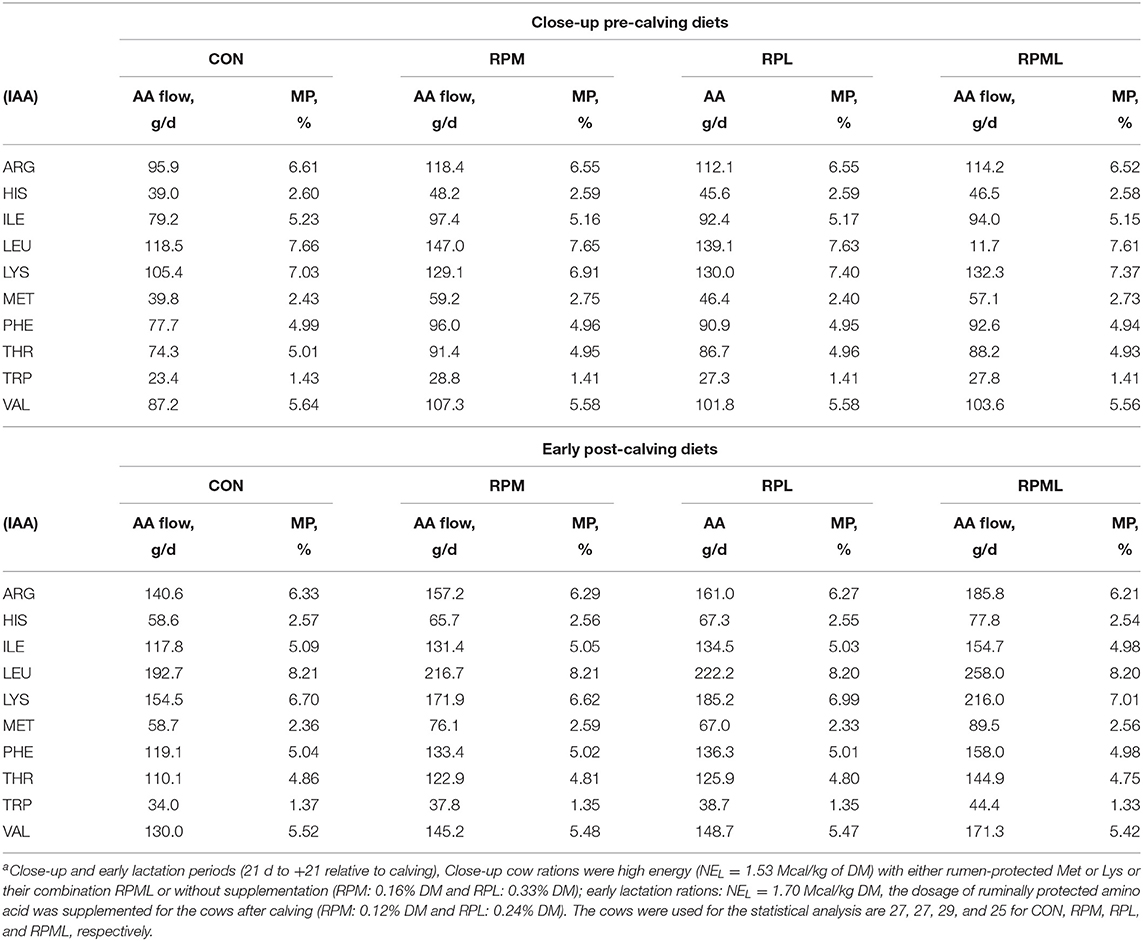
Table 3. Duodenal flows of the digestible indispensable amino acids (IAA) predicted for diets fed during the transition period by the Cornell Net Carbohydrate and Protein System [(CNCPS) v. 6.5.5]a.
Dry Matter Intake
There were significant Trt × Time interactions for DMI (P < 0.001), but DMI of the RPAA treatments was always greater (P < 0.001) than the CON group pre-calving (13.3 vs. 11.2 kg/d) and post-calving (20.6 vs. 17.4 kg/d) (Table 4, Figures 1A,B). DMI was higher for cows that consumed RPM than those cows receiving either RPL or RPML pre-calving (P < 0.001; 13.6 vs. 12.9, 13.2 kg/d, respectively). RPML cows had greater DMI compared with RPM or RPL post-calving (P < 0.001, 22.6 vs. 19.4, 19.8 kg/d, respectively). Cows fed the RPL consumed more DMI than cows fed RPM post-calving (P < 0.001, 19.8 vs. 19.4 kg/d, Table 4).
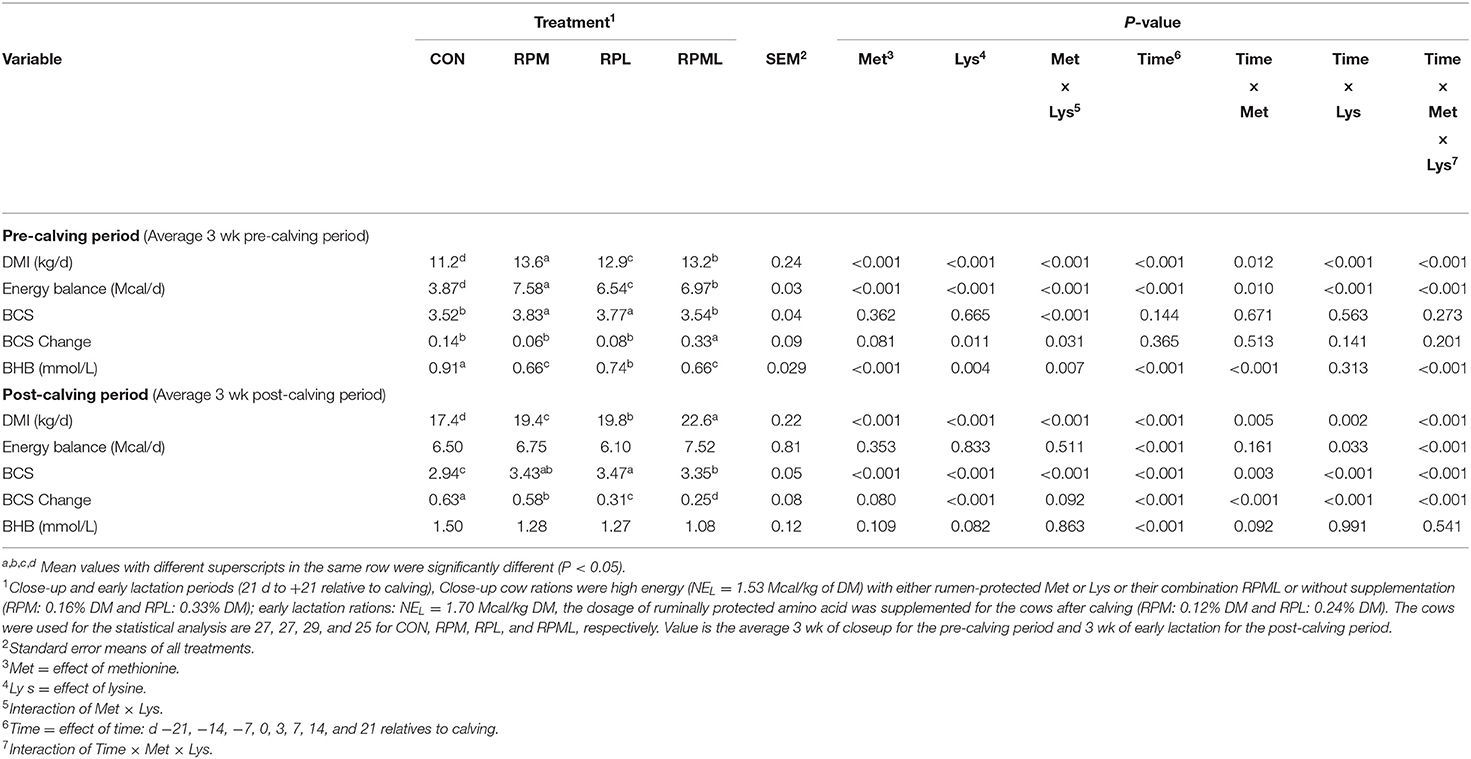
Table 4. Effect of supplementation of ruminally protected Met and Lys on DMI, BCS, Energy Balance, and BHB of Holstein cows during the transition period.
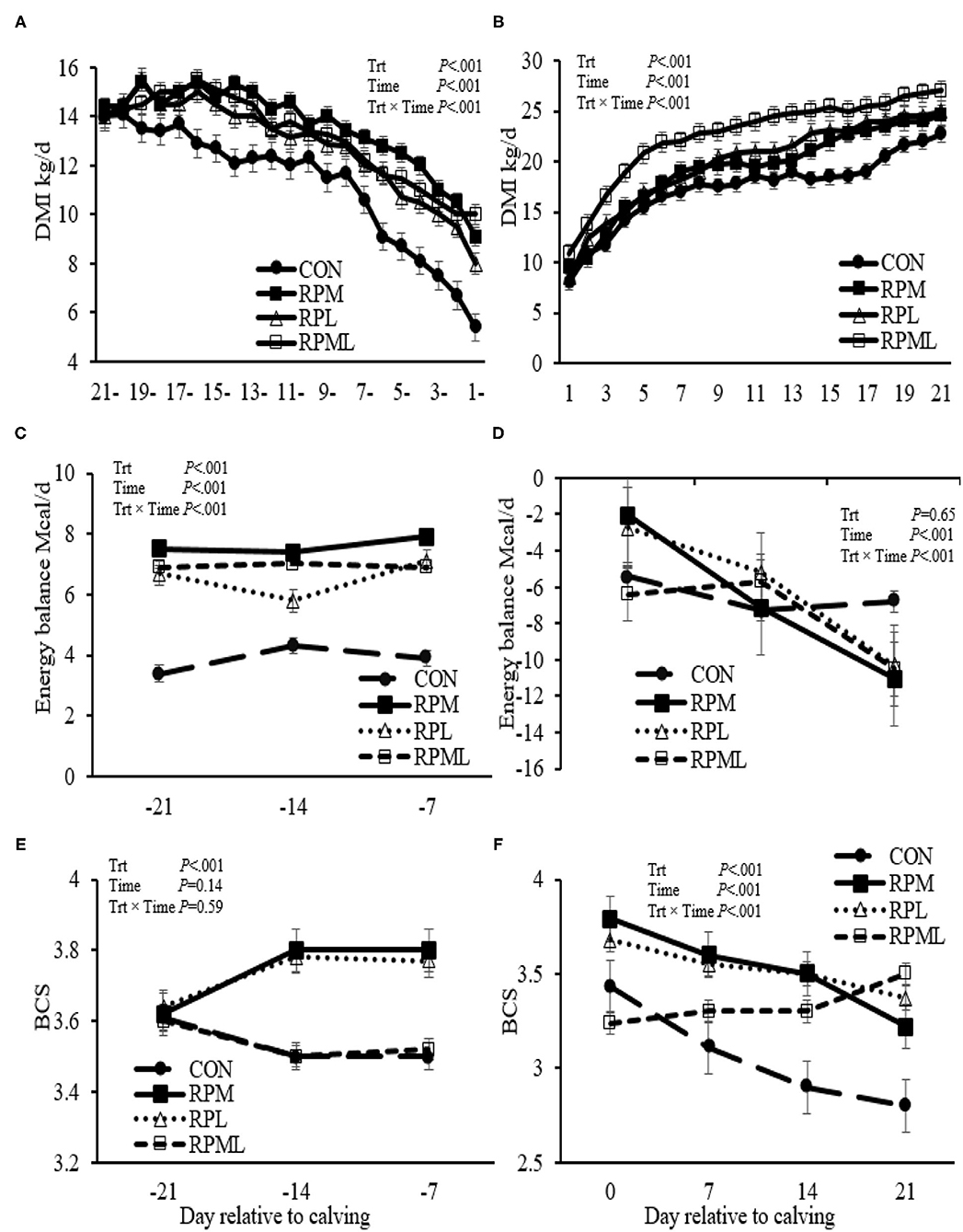
Figure 1. Effect of ruminally protected Lys and Met supplementation on dry matter intake, energy balance (EB; Mcal/d), and body condition score of Holstein dairy cows during the transition period. Values are means; standard errors are represented by vertical bars.
Effects on Energy Balance and Body Condition Score
There was an interaction of Trt × Time for EB, but the supplemental cows were always had greater EB than those cows fed an unsupplemented diet. EB was higher for RPAA cows than cows receiving the CON diet pre-calving (P <0.001; 7.03 vs. 3.87 Mcal). There was no difference between treatments in EB post-calving (P = 0.65). There was an interaction of Trt × Time for EB post-calving (P <0.01), with cows fed CON diet had higher EB at wk 3 (6.85 Mcal) compared with that fed RPM, RPL, and RPML of 11.07, 10.32, and 10.49 Mcal, respectively (P < 0.05) (Table 4, Figures 1C,D).
There was a Trt × Time interaction for BCS post-calving (P < 0.01); cows that received the RPML diet had greater BCS at wk 3 (3.49 units) compared with those in RPM and CON (3.22 and 2.79 units, respectively; P < 0.05) and a tendency for increase BCS compared with those cows in RPL (3.37 units; P = 0.12). BCS was improved by RPAA supply post-calving (P < 0.01, 3.42 vs. 2.94 units). Time had a strong tendency for increase BCS pre-calving (P = 0.14); time affected BCS post-calving (P < 0.001; Table 4, Figures 1E,F). In the contrast analysis conducted for comparing the supplemented AA to the CON, the results indicated that supplemental-AA cows had a smaller BCS change than cows fed CON diet post-calving (P < 0.01; −0.21 vs. −0.63 units). There was no difference between treatments in BCS change pre-calving (P > 0.10).
Effect on Blood β-Hydroxybutyrate Concentrations
Concentrations of blood BHBA decreased with RPM, RPL, or the combination pre-calving (0.69 vs. 0.91 mmol/L; P < 0.01), and there was a strong tendency for a decrease post-calving (1.21 vs. 1.50 mmol/L; P = 0.10) compared to unsupplemented cows (Table 4, Figures 2A,B). There was an interaction of Trt × Time for BHBA pre-calving (P < 0.01), in which CON cows had higher BHBA concentrations at wk −1 (1.04 mmol/L) compared with that fed RPM, PRL, and RPML (0.68, 0.77, and 0.72 mmol/L, respectively; P < 0.001). There was no Trt × Time interaction post-calving (P = 0.54) (Table 4, Figure 2A).
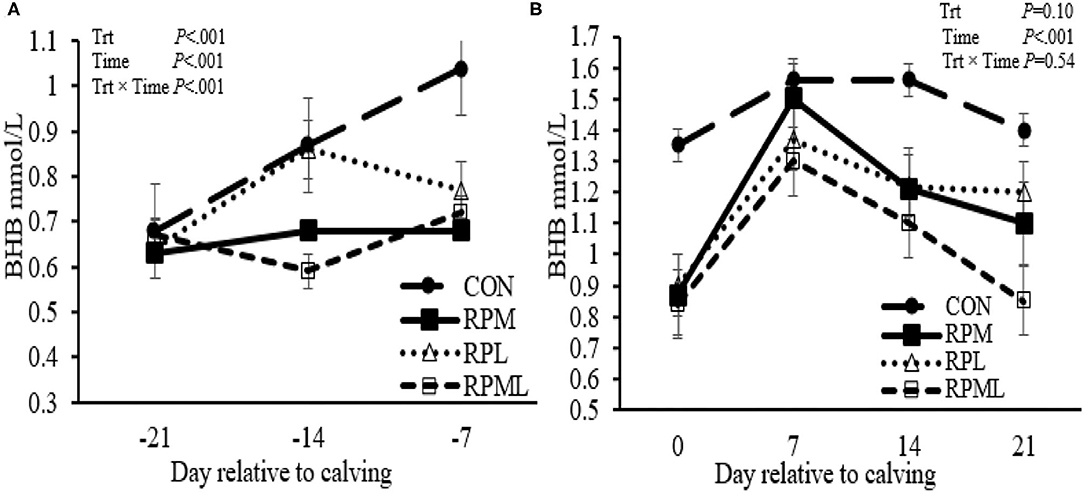
Figure 2. Effect of ruminally protected Lys and Met supplementation on blood β-hydroxybutyrate concentration of Holstein dairy cows during the transition period. Values are means; standard errors are represented by vertical bars.
Effects on Milk Production and Composition
Milk yield was greater (P < 0.05; 41.1 vs. 35.2 kg/d), ECM yield (P < 0.05; 44.4 vs. 36.5 kg/d) for cows fed RPAA was compared with the CON (Table 5, Figures 3A,B). Milk yield and ECM were not different between RPM and RPL treatment (P > 0.05). ECM yield was higher for cows that were fed RPML compared with those cows in RPM and RPL (P < 0.05; 50.2 vs. 41.5 kg/d). Milk fat (4.0 vs. 3.7%), crude protein (3.43 vs. 3.26%), lactose (5.4 vs. 4.8%), and TS (13.3 vs. 12.88%) contents and yield were greater for supplemented cows compared with CON (P < 0.05) (Table 5, Figures 4A–H). MUN of the CON group was always greater (P < 0.01) than RPAA treatments (9.7 vs. 13.74, 10.85, and 11.47 mg/dl) for CON, RPM, RPL, and RPML, respectively (Table 5, Figure 5A). There was a Time × Trt interaction for SCC (P = 0.008), with the CON group having greater SCC at wk 3 (3.47 log-transformed) compared with RPM, PRL, and RPML (3.25, 3.21, and 3.22 log-transformed, respectively; P < 0.05; Table 5, Figure 5B).
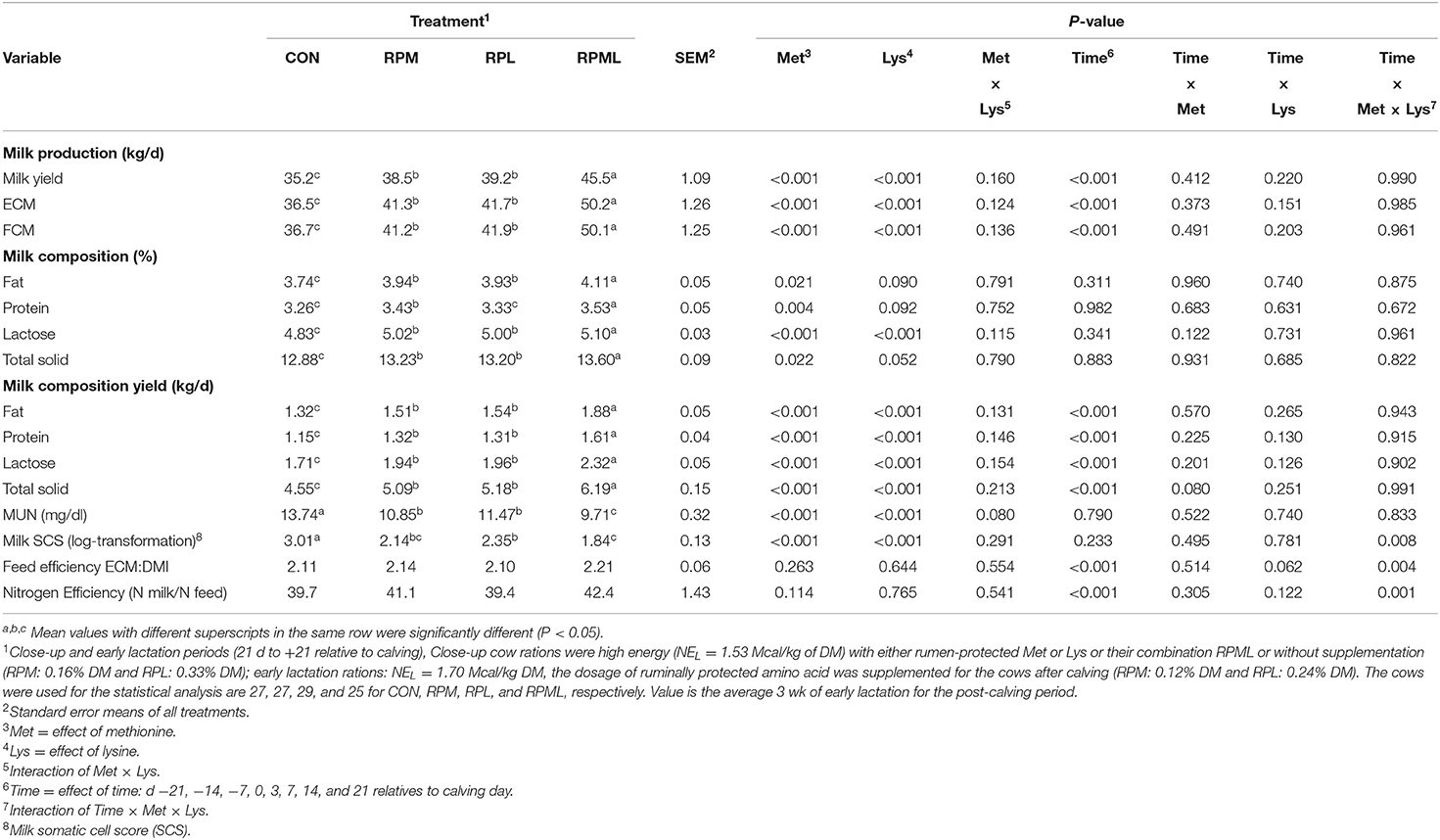
Table 5. Effect of supplementation of ruminally protected Met and Lys on milk yield and composition during 3 wk post-calving in Holstein dairy cows.
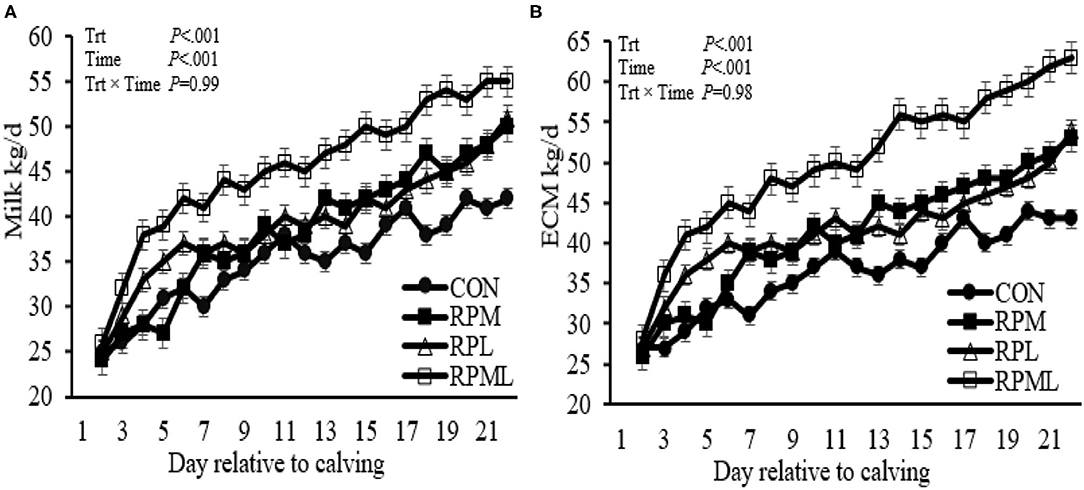
Figure 3. Effect of ruminally protected Lys and Met supplementation on milk production, energy-corrected milk yield of Holstein dairy cows during the transition period. Values are means; standard errors are represented by vertical bars.
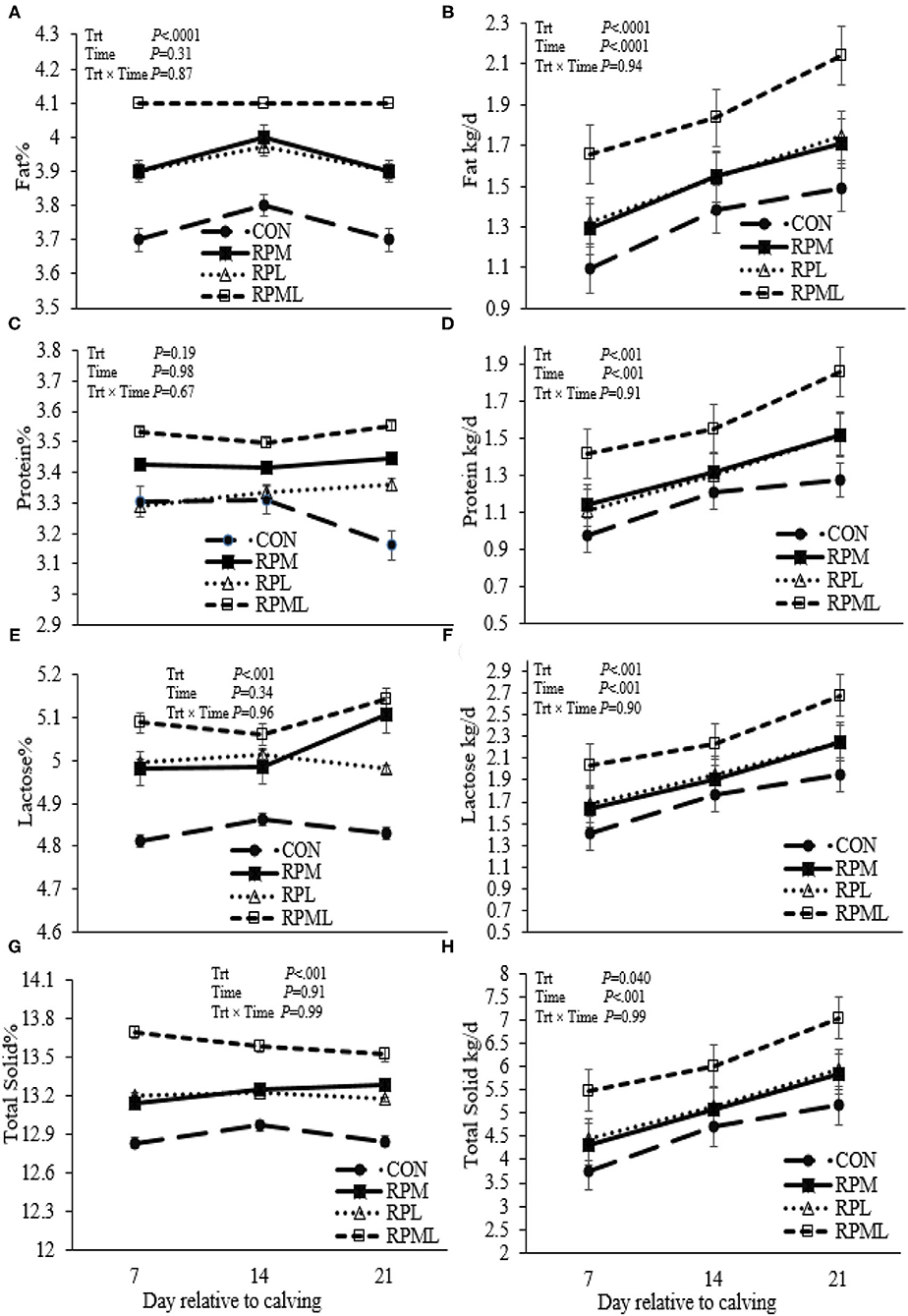
Figure 4. Effect of ruminally protected Lys and Met supplementation on some milk components and yields of Holstein dairy cows during the transition period. Values are means; standard errors are represented by vertical bars.
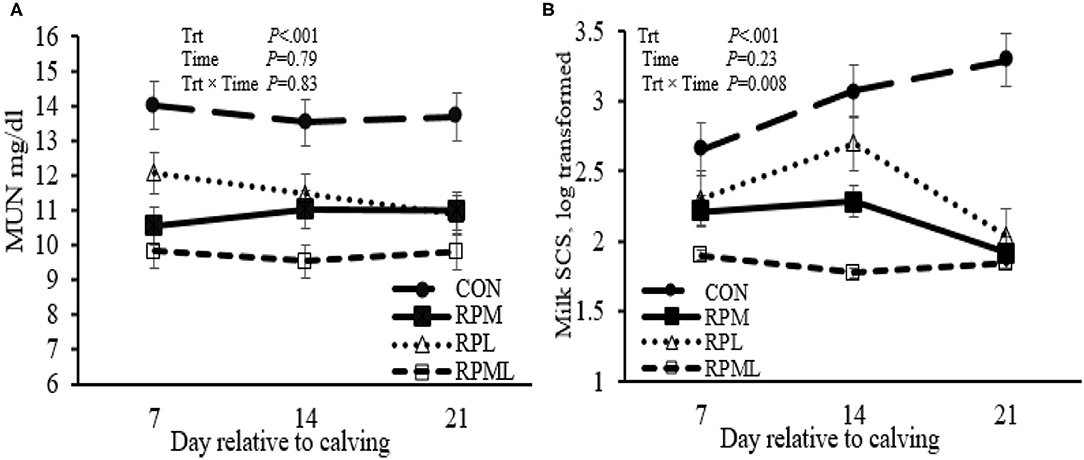
Figure 5. Effect of ruminally protected Lys and Met supplementation on MUN and somatic cell count of Holstein dairy cows during the transition period. Values are means; standard errors are represented by vertical bars.
Feed Efficiency, Nitrogen Efficiency
There were no effects of AA supply on feed efficiency (ECM/DMI; P = 0.63) or nitrogen efficiency (feed N/milk N; P = 0.42). There was a time effect for feed and nitrogen efficiency (P < 0.05; Table 5). There was a Time × Trt interaction for feed efficiency at wk3 (P = 0.04), in which cows fed RPM and RPL had higher feed efficiency (P = 0.05; 2.45 and 2.41 ECM/DMI, respectively).
Discussion
Metabolizable Protein Balance
In the current study, the greater MP was supplied from RPAA diets, which was driven by increased DMI to sustain greater milk yield, improved EB, and carried over to subsequent post-calving performance. This assisted in the replenishment of body condition for cows fed RPML and RPL but not cows fed RPM. Overall, our study's post-calving MP balance in RPM cows was (−344 g/d) compared with a −617 g/d (33). The differences between our study and the prior study could have been partially associated with the stimulation of DMI (4.3 kg/d) greater in the current study than others (33). In addition, in our study, pre-calving diets provided with HMBi, RPL, and RPML delivered an equal amount of MP-Lys and 11 g more of MP-Met than the CON diet; cows that received an AA supply had an extra ca. 30 g of MP-Lys and ca. 12 g of MP-Met post-calving. In the work of Osorio et al. (33), cows that received HMBi had similar amounts of MP-Lys and an additional 7 g of MP-Met than the CON pre-calving, HMBi cows received an extra ca. 15 g of MP-Lys and ca. 11 g of MP-Met during the post-calving period. Similar to our results, they achieved an estimated increase of 9 g/d absorbed Met over the CON diet by supplying a 40.8 g/d HMBi (34).
Dry Matter Intake
In the current study, stimulating DMI resulted in greater energy and total MP intake, which supported greater production and spared BW loss, attributed mainly to increased indispensable AAs (IAA) efficiency. In addition, stimulation of carnitine synthesis has been shown to increase DMI 1 to 3 g/d carnitine in lactating Holstein diets (35). Meyer et al. (36) showed that an L-carnitine supplementation of 25 g of rumen-protected L-carnitine per cow/d did not affect the DMI of transition dairy cows. However, the increased DMI in this study in response to Lys and Met was greater than reported by (35); thus, it is unlikely that carnitine is the sole contributor. Furthermore, other factors such as changes in metabolism, hormone concentrations, AA balance, and inflammatory and neural control may have affected DMI in the transition dairy cows.
The mechanism relating to AA and DMI has been shown in non-ruminants to impact food intake. There is considerable evidence for neural control of food intake in response to imbalanced-AA diets (37, 38). Our study found a 23% decrease in DMI for CON cows fed diets deficient in multiple IAA (−21.8 g MP-AA, equal to a 14.6% AA-deficient rate) as compared to cows fed balanced-IAA diets. Gietzen et al. (37) reported that food intake was decreased 55% for rats fed threonine (THR) deficient diets (12.5% THR deficient rate), similar to the current study. So, one might conclude that the mechanism may be contributing to decreasing DMI in cows fed imbalanced-IAA diets. In our study, a higher 23% DMI and 27.3% milk yield was observed in cows receiving a balanced-IAA diet than those cows that were fed multiple IAA-deficient diets (a 15.4% THR-deficient rate). In comparison, a 12.5% THR deficiency was required to cause a 55% decrease in food intake in rodents (37), which was similar to the AA deficiency in the prior study. It appears that IAA-deficiency in ruminants may have significant effects on DMI, and the reduction in DMI only appears when the IAA-deficiency is relatively large as compared to rodents. The mechanisms underlying the control of the feeding response to the dietary model remain not fully understood, and more research is needed.
The rate of free fatty acid oxidation in the liver also appears to regulate feed intake in ruminants. Allen and Piantoni (39) reported that hepatic oxidation of non-esterified fatty acid (NEFA) stimulated brain satiety centers via the hepatic vagal nerve to suppress intake. It is possible that liver concentrations of fatty acid or fatty acid–derived metabolites such as BHBA are being sensed rather than the oxidation rate (39); in either case, they were exporting more fatty acid as VLDL would lead to less oxidative stress in the liver and avoid intake suppression, which partially may explain the higher DMI for cows fed RPAA diets in the present study.
Increased DMI was generally (13, 40) but not universally (19) observed in response to the provision of RPL before calving. Supply RPL after calving also resulted in greater DMI when consumed pre-calving (13, 19, 40), in agreement with our results. DMI has also responded to RPL in some cases in mid-lactation (41), but not in others (42, 43). No such response was observed in lactating and dry cows (14, 44, 45). DMI has previously been reported to increase with supply RPM pre-calving (16, 46) and post-calving (33), similar to what was observed in our study. However, DMI responses to RPM were not detected pre-partum (47). In contrast to the present study, no response of DMI in transition cows continually consumed RPM and RPL before or after calving (15).
Effects on Energy Balance and Body Condition Score
In our study, the response for feeding RPAA was matched with improved EB that is driven by the increase of DMI leading to 5, 5, and 13 kg/d greater ECM for cows supplemented with RPM or RPL or RPML, respectively, and this maintained BCS post-calving. Osorio et al. (33) showed that RPM cows need greater nutrients, particularly during post-calving, to compensate for high milk yield, agreeing with this work. However, pre- and post-calving EB was not affected by the provision of RPL to transition cows (19). The changes in BCS reflect the EB change observed in cows (48). In the current study, feeding dairy cows RPAA resulted in increased DMI and improved BCS, which minimized the changes in BCS at d 21 post-calving compared to d 21 pre-calving in this study. Dissimilarly, providing RPM pre-calving (14, 33, 49), or RPL did not affect BCS of transition cows (40, 50).
Effect on β-Hydroxybutyrate Concentrations
In the current study, reduced BHBA concentrations with AAs supply are explained by the increased DMI, leading to improved EB, which could have reduced LCFA release from adipose tissue and hepatic conversation of FA to BHBA. During negative EB, there is a large uptake of adipose tissue–derived FA by the liver, which would have resulted in incomplete oxidation of NEFA and, consequently, elevated ketone bodies (10). Excessive adipose tissue mobilization results in incomplete hepatic oxidation and release of ketone bodies (10, 51), which was in the lower subclinical thresholds for cows fed RPML in the present study. Supply RPAA to cows during the transition period had varied effects on β-hydroxybutyrate. For instance, a previous study conducted by our laboratory (40), post-calving BHBA decreased when RPL was continually fed pre- and post-calving (40) or when RPM and RPL were supplemented in early lactation (15); however, post-calving BHBA was not affected by feeding RPL pre-calving (19). The decrease in pre-calving BHBA was only observed when RPL was provided pre-partum (19), in contrast to this experiment.
Another reason for the lower β-hydroxybutyrate with RPAA supply suggests that RPM and RPL might have improved hepatic lipid metabolism and increased carnitine bioavailability. Osorio et al. (33) found a tendency for decreasing the occurrence rate of clinical ketosis when RPM and HMBi were provided to transition cows suggesting that supplied Met might have improved hepatic lipid metabolism, similar to our findings. Lipotropic agents such as Met or choline assist in lipid export from the liver by stimulating VLDL formation (10). Consequently, that response may lead to a decrease in hepatic TAG accumulation and formation of ketone bodies (10, 52), which may assist in decreasing BHBA in the current study due to RPAA supply.
Effects on Milk Production and Composition
In the present study, adequate dietary MP and AA balance have essential roles in improving milk protein content and yield. Similarly, milk protein outputs have been reported to increase by supply RPM to transition and dairy cows (33, 53) or a tendency for an increase in beef cows (20); however, this has not always been observed (9, 15). Authors noted that milk protein yield improves with increased MP (19, 54), which was revealed in the current study, and milk protein is influenced in direct proportion to the adequacy of Met in MP (3). Balancing the AA profile will probably alter milk protein in comparison with milk production (3, 15, 55). The supply of the IAA during the far-off dry period before the supplementation of RPAA during the close-up and its relative to cow's requirements may also affect the degree of response. The deficiency in IAA may also influence animals' response, animals likely should respond even if they were not deficient, but it also depends on how deficient the other IAAs are.
Protein supply is considered the limiting factor for the absorption of AAs in the mammary gland, which will be determined the yield of protein, lactose, and consequently milk production, as milk yield is a derivative of these components (56, 57). In the current study, increase in the supply of protein would increase protein yield and increase milk production and the required amount for lactose synthesis. The most limiting IAA for milk protein yield is Met, Lys, and, probably, His. Milk protein responses to Met are properly documented, and responses to Lys and His are somewhat more changeable but have often been observed (58, 59). Besides Met, Lys, and His, it shows that other two IAA of leucine (Leu) and isoleucine (Ile) are also contributed to driving milk protein production (60, 61). In the current study, milk fat percentage and yield are likely increased due to more available energy. In contrast, when energy is limiting, milk fat yield is possible to decrease. Notably, cows in the CON group are the most affected by this due to low DMI, resulting in less available energy, which was resulted in the least yield of milk fat.
By meeting or exceeding MP pre-calving, cows in the current study could easily adapt to post-partum more efficiently, as indicated by increased DMI, the main driver of milk yield. Supporting this, dairy cows fed MP-insufficient diets supplied with RPM, Lys, and His had milk yields that did not differ from cows provided MP-adequate diets (44), suggesting that providing RPAA- to MP-insufficient diets could be corrected for the deficiency. Several studies have demonstrated increased milk yield with RPM or RPL supply during the transition period (16, 19, 33, 62), in agreement with this study. Response to RPL has also been observed in later lactation cows (53, 63), but this response is not universal (15, 64–66).
The greater milk yield response to supplemental RPAA in this study may also be due to increased efficiency of absorbed AA use (in addition to providing adequate MP). Milk lactose and protein increased with AA supply, resulting in the use of a greater proportion of the unsupplemented IAA for milk protein. Junior et al. (53) reported that increased efficiency of absorbed AA increases milk yield in high-producing dairy cows (53). There are several reasons for the variations in milk production and constituents in response to RPAA supplementation; it is explained by the variability of protection methods for RPAA, which would have resulted in differing bioavailability, which may have accounted for differences in results between studies; the differences in the basal diets feedstuff, the milk production level, and the metabolizable amount of Met and Lys g/MP. In addition, the experimental length and animal type, lactation stage, farm management, and cows' comfort may also affect animal response to RPAA supply.
In the current study, RPAA supply to transition cows may positively affect udder health, as indicated by the lowered milk SCC. This improvement may be explained by that the RPAA plays an important role in improving immune status in transition cows through enhanced phagocytosis and oxidative functions, as reported when RPM was fed to dairy cows pre-calving and post-calving continuously (62). Many reasons can cause an increase in milk SCC; one of these, the inflammation in the mammary gland tissues (67), cow productivity, health status, lactation, lactation phase, and animal breed (68). The decrease of MUN observed in this study by providing RPAA to transition cows is indicative of greater efficiency of nitrogen use and an improvement in AA efficiency due to increase milk protein output. MUN decreased by feeding RPAA of either Met, Lys, Leu, and the combination to mid-to-late lactation dairy cows (69), or RP Met, THR, Ile, and Leu to mid-lactation (70), similar to what observed in the present study, but not in others (19, 65).
Conclusions
In the current study, from the practical side, a continual supply of a combination of RPL and RPM throughout the transition period increased DMI and ECM production as well as improved EB. From the health side, the average β-hydroxybutyrate concentrations during the 3-wk post-calving period for cows that supplied either RPL or RPM were at the lower thresholds range of subclinical ketosis, whereas cows fed a combination was in the normal range of β-hydroxybutyrate concentrations. The results from the present study suggested that continual feeding RPL and RPM to transition cows is a practical strategy to improve milk production and performance efficiency and the health of perinatal dairy cows.
Data Availability Statement
The raw data supporting the conclusions of this article will be made available by the authors, without undue reservation.
Ethics Statement
The animal study was reviewed and approved by the Animal Care and Use Committee of the Institute of Animal Science, Chinese Academy of Agricultural Sciences, Beijing, China, approved the study protocol (No. IAS20180115).
Author Contributions
SE: conceptualization, conducted the experiment, laboratory analysis, writing—original draft, and formal analysis. HW: conducted the experiment. ZW: investigation. MH: review and editing. DB: conceptualization, review and editing, investigation, supervision, project administration, and funding acquisition. All authors contributed to the article and approved the submitted version.
Funding
This research was supported by the Key Research and Development Program of the Ningxia Hui Autonomous Region (2021BEF02018), the International Atomic Energy Agency Technical Co-operation and Assistance Programme (No. CPR5025), the Agriculture Science and Technology Innovation Program (ASTIP-IAS07-1), Chinese Academy of Agricultural Science and Technology Innovation Project (CAAS-XTCX2016011-01), and Beijing Dairy Industry Innovation Team (BAIC06-2021). SE was supported by a Ph.D. scholarship from the Graduate School of Chinese Academy of Agricultural Sciences (GSCAAS, Beijing, China).
Conflict of Interest
The authors declare that the research was conducted in the absence of any commercial or financial relationships that could be construed as a potential conflict of interest.
Publisher's Note
All claims expressed in this article are solely those of the authors and do not necessarily represent those of their affiliated organizations, or those of the publisher, the editors and the reviewers. Any product that may be evaluated in this article, or claim that may be made by its manufacturer, is not guaranteed or endorsed by the publisher.
Acknowledgments
We are very grateful to Prof. Richard Kellems from Brigham Young University (USA) for his contribution to manuscript revision. The authors gratefully acknowledge the staff of AustAsia dairy Group for their assistance in conducting the experiment and providing the cows that were used in this study.
References
1. Drackley JK. ADSA foundation scholar award: Biology of dairy cows during the transition period: The final frontier? J Dairy Sci. (1999) 82:2259–73. doi: 10.3168/jds.S0022-0302(99)75474-3
2. Grummer RR, Mashek DG, Hayirli A. Dry matter intake and energy balance in the transition period. Vet Clin North Am Food Anim Pract. (2004) 20:447–70. doi: 10.1016/j.cvfa.2004.06.013
3. NRC. Nutrient Requirements of Dairy Cattle. Nutrient Requirements of Dairy Cattle. 7th Revised Edition. National Research Council. Washington, DC (2001).
4. Vyas D, Erdman RA. Meta-analysis of milk protein yield responses to lysine and methionine supplementation. J Dairy Sci. (2009) 92:5011–8. doi: 10.3168/jds.2008-1769
5. Lean IJ, de Ondarza MB, Sniffen CJ, Santos JEP, Griswold KE. Meta-analysis to predict the effects of metabolizable amino acids on dairy cattle performance. J Dairy Sci. (2018) 101:340–64. doi: 10.3168/jds.2016-12493
6. Appuhamy JADRN, Knapp JR, Becvar O, Escobar J, Hanigan MD. Effects of jugular-infused lysine, methionine, and branched-chain amino acids on milk protein synthesis in high-producing dairy cows. J Dairy Sci. (2011) 94:1952–60. doi: 10.3168/jds.2010-3442
7. Schwab CG, Broderick GA. A 100-Year Review: Protein and amino acid nutrition in dairy cows. J Dairy Sci. (2017) 100:10094–112. doi: 10.3168/jds.2017-13320
8. Coleman DN, Alharthi AS, Liang Y, Lopes MG, Lopreiato V, Vailati-Riboni M, et al. Multifaceted role of one-carbon metabolism on immunometabolic control and growth during pregnancy, lactation and the neonatal period in dairy cattle. J Anim Sci Biotechnol. (2021) 12:27. doi: 10.1186/s40104-021-00547-5
9. Davidson S, Hopkins BA, Odle J, Brownie C, Fellner V, Whitlow LW. Supplementing Limited Methionine Diets with Rumen-Protected Methionine, Betaine, and Choline in Early Lactation Holstein Cows. J Dairy Sci. (2008) 91:1552–9. doi: 10.3168/jds.2007-0721
10. Bauchart D, Durand D, Gruffat D, Chilliard Y. Mechanisms of liver steatosis in early lactation cows - Effects of hepatoreceptor agents. In: Proceedings of the Cornell Nutrition Conference for Feed Manufactures. New York (1998). pp. 27–37.
11. Martinov MV, Vitvitsky VM, Banerjee R, Ataullakhanov FI. The logic of the hepatic methionine metabolic cycle. Biochim Biophys Acta. (2010) 1804:89–96. doi: 10.1016/j.bbapap.2009.10.004
12. Carlson DB, Litherland NB, Dann HM, Woodworth JC, Drackley JK. Metabolic effects of abomasal L-carnitine infusion and feed restriction in lactating holstein cows. J Dairy Sci. (2006) 89:4819–34. doi: 10.3168/jds.S0022-0302(06)72531-0
13. Xu S, Harrison JH, Chalupa W, Sniffen C, Julien W, Sato H, et al. The Effect of Ruminai Bypass Lysine and Methionine on Milk Yield and Composition of Lactating Cows. J Dairy Sci. (1998) 81:1062–77. doi: 10.3168/jds.S0022-0302(98)75668-1
14. Socha MT, Putnam DE, Garthwaite BD, Whitehouse NL, Kierstead NA, Schwab CG, et al. Improving intestinal amino acid supply of pre- and postpartum dairy cows with rumen-protected methionine and lysine. J Dairy Sci. (2005) 88:1113–26. doi: 10.3168/jds.S0022-0302(05)72778-8
15. Lee C, Lobos NE, Weiss WP. Effects of supplementing rumen-protected lysine and methionine during prepartum and postpartum periods on performance of dairy cows. J Dairy Sci. (2019) 102:11026–39. doi: 10.3168/jds.2019-17125
16. Batistel F, Arroyo JM, Bellingeri A, Wang L, Saremi B, Parys C, et al. Ethyl-cellulose rumen-protected methionine enhances performance during the periparturient period and early lactation in Holstein dairy cows. J Dairy Sci. (2017) 100:7455–67. doi: 10.3168/jds.2017-12689
17. Zhou Z, Vailati-Riboni M, Trevisi E, Drackley JK, Luchini DN, Loor JJ. Better postpartal performance in dairy cows supplemented with rumen-protected methionine compared with choline during the peripartal period. J Dairy Sci. (2016) 99:8716–32. doi: 10.3168/jds.2015-10525
18. Lievre M. Effects of Metabolizable Protein and Supplemental Methionine in Late Gestation on Colostrum Quality and Transfer of Passive Immunity in Beef Cattle (Doctoral dissertation) (2020).
19. Fehlberg LK, Guadagnin AR, Thomas BL, Sugimoto Y, Shinzato I, Cardoso FC. Feeding rumen-protected lysine prepartum increases energy-corrected milk and milk component yields in Holstein cows during early lactation. J Dairy Sci. (2020) 103:11386–400. doi: 10.3168/jds.2020-18542
20. Silva GM, Chalk CD, Ranches J, Schulmeister TM, Henry DD, DiLorenzo N, et al. Effect of rumen-protected methionine supplementation to beef cows during the periconception period on performance of cows, calves, and subsequent offspring. Animal. (2021) 15:100055. doi: 10.1016/j.animal.2020.100055
21. Deye N, Vincent F, Michel P, Ehrmann S, Da Silva D, Piagnerelli M, et al. Changes in cardiac arrest patients' temperature management after the 2013 “TTM” trial: Results from an international survey. Ann Intens. (2016) 6:1–9. doi: 10.1186/s13613-015-0104-6
22. Batistel F, Arroyo JM, Garces CIM, Trevisi E, Parys C, Ballou MA, et al. Ethyl-cellulose rumen-protected methionine alleviates inflammation and oxidative stress and improves neutrophil function during the periparturient period and early lactation in Holstein dairy cows. J Dairy Sci. (2018) 101:480–90. doi: 10.3168/jds.2017-13185
23. Elsaadawy S, Wang H, Wu Z, Bu D. Supplementing ruminally protected methionine or lysine improved milk production in transition cows. J Dairy Sci Abstr Annu Meet Am Dairy Sci Assoc. (2021) 104(Suppl. 1):97. Available online at: https://www.adsa.org/Portals/0/SiteContent/Docs/Meetings/2021ADSA/ADSA2021_Abstracts.pdf
24. Wang H, Elsaadawy SA, Wu Z, Bu DP. Maternal Supply of Ruminally-Protected Lysine and Methionine During Close-Up Period Enhances Immunity and Growth Rate of Neonatal Calves. Front Vet Sci. (2021) 8:780731. doi: 10.3389/fvets.2021.780731
25. Faul F, Erdfelder E, Buchner A. Lang AG. Statistical power analyses using G*Power 31: Tests for correlation and regression analyses. Behav Res Methods. (2009) 41:1149–60. doi: 10.3758/BRM.41.4.1149
26. NRC. Nutrient Requirements of Domestic Animals. Washington, DC: National Academy of Science, National Research Council (1956).
27. AOAC International. Official Methods of Analysis. 17th ed. Gaithersburg, MD: AOAC International (2000).
28. Van Soest PJ, Robertson JB, Lewis BA. Methods for Dietary Fiber, Neutral Detergent Fiber, and Nonstarch Polysaccharides in Relation to Animal Nutrition. J Dairy Sci. (1991) 74:3583–97. doi: 10.3168/jds.S0022-0302(91)78551-2
29. Tyrrell HF, Reid JT. Prediction of the Energy Value of Cow's Milk. J Dairy Sci. (1965) 48:1215–23. doi: 10.3168/jds.S0022-0302(65)88430-2
30. Kononoff PJ, Heinrichs AJ, Buckmaster DR. Modification of the Penn State Forage and Total Mixed Ration Particle Separator and the Effects of Moisture Content on its Measurements. J Dairy Sci. (2003) 86:1858–63. doi: 10.3168/jds.S0022-0302(03)73773-4
31. Shook GE. Major advances in determining appropriate selection goals. J Dairy Sci. (2006) 89:1349–61. doi: 10.3168/jds.S0022-0302(06)72202-0
32. Littell RC. Analysis of unbalanced mixed model data: a case study comparison of ANOVA versus REML/GLS. J Agric Biol Environ Stat. (2002) 7:472–90. doi: 10.1198/108571102816
33. Osorio JS, Ji P, Drackley JK, Luchini D, Loor JJ. Supplemental Smartamine M or MetaSmart during the transition period benefits postpartal cow performance and blood neutrophil function. J Dairy Sci. (2013) 96:6248–63. doi: 10.3168/jds.2012-5790
34. Chen ZH, Broderick GA, Luchini ND, Sloan BK, Devillard E. Effect of feeding different sources of rumen-protected methionine on milk production and N-utilization in lactating dairy cows. J Dairy Sci. (2011) 94:1978–88. doi: 10.3168/jds.2010-3578
35. Carlson DB, Woodworth JC, Drackley JK. Effect of l-Carnitine Infusion and Feed Restriction on Carnitine Status in Lactating Holstein Cows. J Dairy Sci. (2007) 90:2367–76. doi: 10.3168/jds.2006-605
36. Meyer J, Daniels SU, Grindler S, Tröscher-Mußotter J, Alaedin M, Frahm J, et al. Effects of a Dietary L-Carnitine Supplementation on Performance, Energy Metabolism and Recovery from Calving in Dairy Cows. Animals. (2020) 10:342. doi: 10.3390/ani10020342
37. Gietzen DW, Leung PMB, Rogers QR. Norepinephrine and amino acids in prepyriform cortex of rats fed imbalanced amino acid diets. Physiol Behav. (1986) 36:1071–80. doi: 10.1016/0031-9384(86)90482-8
38. Gietzen DW, Hao S, Anthony TG. Mechanisms of food intake repression in indispensable amino acid deficiency. Annu Rev Nutr. (2007) 27:63–78. doi: 10.1146/annurev.nutr.27.061406.093726
39. Allen MS, Piantoni P. Metabolic control of feed intake. Vet Clin North Am Food Anim Pract. (2013) 29:279–97. doi: 10.1016/j.cvfa.2013.04.001
40. Girma DD, Ma L, Wang F, Jiang QR, Callaway TR, Drackley JK, et al. Effects of close-up dietary energy level and supplementing rumen-protected lysine on energy metabolites and milk production in transition cows. J Dairy Sci. (2019) 102:7059–72. doi: 10.3168/jds.2018-15962
41. Swanepoel N, Robinson PH, Erasmus LJ. Amino acid needs of lactating dairy cows: Impact of feeding lysine in a ruminally protected form on productivity of lactating dairy cows. Anim Feed Sci Technol. (2010) 157:79–94. doi: 10.1016/j.anifeedsci.2010.02.008
42. Weiss WP. Effects of feeding diets composed of corn silage and a corn milling product with and without supplemental lysine and methionine to dairy cows. J Dairy Sci. (2019) 102:2075–84. doi: 10.3168/jds.2018-15535
43. Morris DL, Kononoff PJ. Effects of rumen-protected lysine and histidine on milk production and energy and nitrogen utilization in diets containing hydrolyzed feather meal fed to lactating Jersey cows. J Dairy Sci. (2020) 103:7110–23. doi: 10.3168/jds.2020-18368
44. Giallongo F, Harper MT, Oh J, Lopes JC, Lapierre H, Patton RA, et al. Effects of rumen-protected methionine, lysine, and histidine on lactation performance of dairy cows. J Dairy Sci. (2016) 99:4437–52. doi: 10.3168/jds.2015-10822
45. Misciattelli L, Kristensen VF, Vestergaard M, Weisbjerg MR, Sejrsen K, Hvelplund T. Milk production, nutrient utilization, and endocrine responses to increased postruminal lysine and methionine supply in dairy cows. J Dairy Sci. (2003) 86:275–86. doi: 10.3168/jds.S0022-0302(03)73606-6
46. Alharthi AS, Batistel F, Abdelmegeid MK, Lascano G, Parys C, Helmbrecht A, et al. Maternal supply of methionine during late-pregnancy enhances rate of Holstein calf development in utero and postnatal growth to a greater extent than colostrum source. J Anim Sci Biotechnol. (2018) 9:83. doi: 10.1186/s40104-018-0298-1
47. Toledo MZ, Stangaferro ML, Gennari RS, Barletta RV, Perez MM, Wijma R, et al. Effects of feeding rumen-protected methionine pre- and postpartum in multiparous Holstein cows: Lactation performance and plasma amino acid concentrations. J Dairy Sci. (2021) 104:7583–603. doi: 10.3168/jds.2020-19021
48. Thorup VM, Edwards D, Friggens NC. On-farm estimation of energy balance in dairy cows using only frequent body weight measurements and body condition score. J Dairy Sci. (2012) 95:1784–93. doi: 10.3168/jds.2011-4631
49. Ordway RS, Boucher SE, Whitehouse NL, Schwab CG, Sloan BK. Effects of providing two forms of supplemental methionine to periparturient Holstein dairy cows on feed intake and lactational performance. J Dairy Sci. (2009) 92:5154–66. doi: 10.3168/jds.2009-2259
50. Robinson PH, Swanepoel N, Shinzato I, Juchem SO. Productive responses of lactating dairy cattle to supplementing high levels of ruminally protected lysine using a rumen protection technology. Anim Feed Sci Technol. (2011) 168:30–41. doi: 10.1016/j.anifeedsci.2011.03.019
51. Hanigan MD, Crompton LA, Reynolds CK, Wray-Cahen D, Lomax MA, France J. An integrative model of amino acid metabolism in the liver of the lactating dairy cow. J Theor Biol. (2004) 228:271–89. doi: 10.1016/j.jtbi.2004.01.010
52. Waterman R, Schultz LH. Methionine Hydroxy Analog Treatment of Bovine Ketosis: Effects on Circulating Metabolites and Interrelationships. J Dairy Sci. (1972) 55:1513–6. doi: 10.3168/jds.S0022-0302(72)85707-2
53. Junior VC, Lopes F, Schwab CG, Toledo MZ, Collao-Saenz EA. Effects of rumen-protected methionine supplementation on the performance of high production dairy cows in the tropics. Loor JJ, editor. PLoS ONE. (2021) 16:e0243953. doi: 10.1371/journal.pone.0243953
54. Cant JP, Kim JJM, Cieslar SRL, Doelman J. Symposium review: amino acid uptake by the mammary glands: where does the control lie? J Dairy Sci. (2018) 101:5655–66. doi: 10.3168/jds.2017-13844
55. Robinson PH. Impacts of manipulating ration metabolizable lysine and methionine levels on the performance of lactating dairy cows: a systematic review of the literature. Livest Sci. (2010) 127:115–26. doi: 10.1016/j.livsci.2009.10.003
56. Larsen M, Lapierre H, Kristensen NB. Abomasal protein infusion in postpartum transition dairy cows: effect on performance and mammary metabolism. J Dairy Sci. (2014) 97:5608–22. doi: 10.3168/jds.2013-7247
57. Lapierre H, Berthiaume R, Raggio G, Thivierge MC, Doepel L, Pacheco D, et al. The route of absorbed nitrogen into milk protein. Anim Sci. (2005) 80:11–22. doi: 10.1079/ASC41330011
58. Giallongo F, Harper MT, Oh J, Parys C, Shinzato I, Hristov AN. Histidine deficiency has a negative effect on lactational performance of dairy cows. J Dairy Sci. (2017) 100:2784–800. doi: 10.3168/jds.2016-11992
59. Lee C, Hristov AN, Cassidy TW, Heyler KS, Lapierre H, Varga GA, et al. Rumen-protected lysine, methionine, and histidine increase milk protein yield in dairy cows fed a metabolizable protein-deficient diet. J Dairy Sci. (2012) 95:6042–56. doi: 10.3168/jds.2012-5581
60. Yoder PS, Huang X, Teixeira IA, Cant JP, Hanigan MD. Effects of jugular infused methionine, lysine, and histidine as a group or leucine and isoleucine as a group on production and metabolism in lactating dairy cows. J Dairy Sci. (2020) 103:2387–404. doi: 10.3168/jds.2019-17082
61. Lapierre H, Martineau R, Hanigan MD, Van Lingen HJ, Kebreab E, Spek JW, et al. Review: Impact of protein and energy supply on the fate of amino acids from absorption to milk protein in dairy cows. Animal. (2020) 14:S87–102. doi: 10.1017/S1751731119003173
62. Zhou Z, Bulgari O, Vailati-Riboni M, Trevisi E, Ballou MA, Cardoso FC, et al. Rumen-protected methionine compared with rumen-protected choline improves immunometabolic status in dairy cows during the peripartal period. J Dairy Sci. (2016) 99:8956–69. doi: 10.3168/jds.2016-10986
63. Bernard JK, Chandler PT, Sniffen CJ, Chalupa W. Response of cows to rumen-protected lysine after peak lactation. Prof Anim Sci. (2014) 30:407–12. doi: 10.15232/pas.2014-01307
64. Robinson PH, Swanepoel N, Evans E. Effects of feeding a ruminally protected lysine product, with or without isoleucine, valine and histidine, to lactating dairy cows on their productive performance and plasma amino acid profiles. Anim Feed Sci Technol. (2010) 161:75–84. doi: 10.1016/j.anifeedsci.2010.07.017
65. Clements AR, Ireland FA, Freitas T, Tucker H, Shike DW. Effects of supplementing methionine hydroxy analog on beef cow performance, milk production, reproduction, and preweaning calf performance1. J Anim Sci. (2017) 95:5597–605. doi: 10.2527/jas2017.1828
66. Redifer C, Tucker H, Loy D, Gunn P. 266 Evaluation of peri-partum supplementation of methionine hydroxy analog on cow-calf performance. J Anim Sci. (2018) 96(suppl_3):90–1. doi: 10.1093/jas/sky404.200
67. Schukken YH, Wilson DJ, Welcome F, Garrison-Tikofsky L, Gonzalez RN. Monitoring udder health and milk quality using somatic cell counts. Vet Res. (2003) 34:579–96. doi: 10.1051/vetres:2003028
68. Alhussien MN, Dang AK. Milk somatic cells, factors influencing their release, future prospects, and practical utility in dairy animals: an overview. Vet World. (2018) 11:562–77. doi: 10.14202/vetworld.2018.562-577
69. Arriola Apelo SI, Bell AL, Estes K, Ropelewski J, de Veth MJ, Hanigan MD. Effects of reduced dietary protein and supplemental rumen-protected essential amino acids on the nitrogen efficiency of dairy cows. J Dairy Sci. (2014) 97:5688–99. doi: 10.3168/jds.2013-7833
Keywords: periparturient cattle, amino acids, energy balance, methionine, β-hydroxybutyrate, milk, lysine, body condition score
Citation: Elsaadawy SA, Wu Z, Wang H, Hanigan MD and Bu D (2022) Supplementing Ruminally Protected Lysine, Methionine, or Combination Improved Milk Production in Transition Dairy Cows. Front. Vet. Sci. 9:780637. doi: 10.3389/fvets.2022.780637
Received: 21 September 2021; Accepted: 14 February 2022;
Published: 25 March 2022.
Edited by:
Ahmed Elolimy, National Research Centre, EgyptReviewed by:
Abdulrahman Alharthi, King Saud University, Saudi ArabiaJacquelyn Boerman, Purdue University, United States
Copyright © 2022 Elsaadawy, Wu, Wang, Hanigan and Bu. This is an open-access article distributed under the terms of the Creative Commons Attribution License (CC BY). The use, distribution or reproduction in other forums is permitted, provided the original author(s) and the copyright owner(s) are credited and that the original publication in this journal is cited, in accordance with accepted academic practice. No use, distribution or reproduction is permitted which does not comply with these terms.
*Correspondence: Dengpan Bu, budengpan@caas.cn