The Thermal and Mechanical Properties of Medium Chain-Length Polyhydroxyalkanoates Produced by Pseudomonas putida LS46 on Various Substrates
- Department of Biosystems Engineering, University of Manitoba, Winnipeg, MB, Canada
Medium chain-length polyhydroxyalkanoates (mcl-PHA) were produced by Pseudomonas putida LS46 cultured with a variety of carbohydrate and fatty acid substrates. The monomer compositions and molecular weights of the polymers varied greatly and was dependent on whether the substrate was metabolized via the fatty acid degradation or the de novo fatty acid synthesis pathways. The highest molecular weights were obtained from medium chain-length fatty acids, whereas low molecular weights were obtained from longer chain-length and more unsaturated fatty acids or carbohydrates. The differences in monomer compositions and molecular weights due to the choice of substrate did not affect the polymer thermal degradation point. The glass transition temperatures varied from −39.4°C to −52.7°C. The melting points, when observed, ranged from 43.2°C to 51.2°C. However, a profound substrate effect was observed on the crystallinity of these polymers. Reduced crystallinity was observed when the monomer compositions deviated away from C8–C10 monomer lengths. The highest crystallinity was observed from medium chain-length fatty acids, which resulted in polymers with the highest tensile strength. The polymer produced from octanoic acid exhibited the highest tensile strength of 4.3 MPa with an elongation-at-break of 162%, whereas the polymers produced from unsaturated, long-chain fatty acids remained amorphous. A comparative analysis of the substrate effect on the physical-mechanical and thermal properties of mcl-PHAs better clarifies the relationship between the monomer composition and their potential applications, and also aids to direct future PHA synthesis research toward properties of interest.
Introduction
Polyhydroxyalkanoates (PHAs) are a diverse class of microbially-produced biopolymers known to vary in sidechain length, monomer back-bone length and functional groups (Steinbüchel and Valentin, 1995; Kim et al., 2007). The polymer structure is determined by microbial biosynthetic pathways, and is affected by the microbial species, the carbon substrate and the culturing conditions. This variability has produced PHAs with drastically different polymer properties (Brandl et al., 1988). PHAs have been compared to polypropylene, low-density polyethylene, rubber and adhesives due to the range of observed PHA properties (Anderson and Dawes, 1990; de Koning et al., 1994; Chen, 2010; Mozejko and Ciesielski, 2014). Possible applications for PHAs include molds and films for replacing single-use plastics, laminates, composites, coatings, adhesives, and biocompatible products for the biomedical (Madison and Huisman, 1999).
The commercial applications of PHAs have been limited by the comparatively high cost of production against competing petrochemical plastics. Substrate utilization has represented 30–50% of the reported PHA production costs, imparting the need for cheaper waste sources of triacylglycerides and simple sugars (Chanprateep, 2010; Jiang et al., 2016; Favaro et al., 2019). For further cost reduction, culturing conditions are optimized for high volumetric productivity of PHAs using a variety of feeding methods and co-substrates to obtain desired monomer compositions and improve the yield coefficient of carbon for PHA production (Blunt et al., 2018b).
The objective of PHA production is sustainable and renewable plastic products capable of replacing current petroleum-derived plastics that are non-biodegradable and a major source of environmental pollution. The challenge of using waste substrates to reduce PHA production costs is the affected chemical, thermal and mechanical properties of the polymer. The production and properties of medium chain-length PHA (mcl-PHA) have been studied on a wide range of substrates using various Pseudomonas spp. subjected to nutrient-limited minimal medium. Mcl-PHAs have been “tailor-made” based on changes in monomer composition, but better understanding of these effects on thermal and mechanical properties are required to justify a preferred monomer composition for a given application. In this study, the cell mass production, PHA production, polymer subunit composition, and molecular weights of mcl-PHAs synthesized by Pseudomonas putida LS46 were assessed for a suite of carbohydrate and fatty acid substrates. This analysis was conducted to further understand the effect of substrate type on mcl-PHA biosynthesis, removing the variability of strain and culturing conditions. The corresponding thermal and mechanical properties are reported. This further elucidates the biochemical response to substrate changes by these microorganisms during PHA production, and how the resulting material properties of these mcl-PHAs can direct production design for niche applications.
Methods and Materials
Culturing and PHA Synthesis
P. putida LS46 (International Depository Authority of Canada Accession Number 181110-03) (Sharma et al., 2012) was used for production of PHAs. Frozen stocks were revived using LB medium. All culturing occurred at 30°C. Minimal growth medium (Ramsay et al., 1990) was used for both inoculum and experimental conditions and adjusted to pH 7. 160 C-mM of substrate was added to minimal medium, however substrate toxicity was observed in some conditions. Hexanoic acid, heptanoic acid, octanoic acid and nonanoic acid were provided 120 C-mM (Blunt et al., 2018a), while shorter fatty acids could not be provided in sufficient concentration to effectively synthesize mcl-PHA under these conditions, and were produced with ammonium sulfate and substrate reduced to 0.1 g/L and 16 C-mM (our unpublished data). Reagent grade chemicals were used for medium production (Sigma Chemical Co., St. Louis, MO; Fisher Scientific, Toronto, ON). Food-grade vegetable oils were hydrolyzed to their respective LCFAs. The composition of canola LCFAs was 4.7% palmitic acid, 2.1% steric acid, 67.1% oleic acid, 16.8% linoleic acid, and 6.1% linolenic acid. The composition of flax LCFAs was 6.7% palmitic acid, 4.7% steric acid, 21.1% oleic acid, 15.1% linoleic acid, and 52.4% linolenic acid.
The inocula were prepared in 250 mL baffled shaker flasks with 50 mL working volumes and cultured in a shaking incubator overnight. A 1% inoculum (v/v) to 500 mL baffled experimental flasks with 150 mL working volumes were incubated in a rotary shaker for 30 h for the determination of chemical and thermal properties. Scale-up of PHA production for the determination of tensile properties was performed using 1 L baffled flasks or in a 7 L bioreactor (Applikon Biotechnology, Foster City, CA).
Biomass Processing
The culture was centrifuged at 16,000 × g for 10 min. The supernatant was discarded, and the pellet was washed with PBS buffer before being re-suspended in H2O and transferred to pre-weighed aluminum dishes. The biomass was dried at 60°C for 24 h and weighed to determine cell dry mass. The PHA was converted to methyl ester monomers by methanolysis of 5 mg of dried biomass (Brandl et al., 1988). A flame ionization detector on the Agilent 7890 gas chromatograph after separation through an Agilent DB-23 column were used to detect the produced methyl esters. Response factors were determined using purchased standards where available (Blunt et al., 2018a), which were used to determine the monomer composition and PHA content. GC-MS confirmed unsaturated PHA monomers, and their response factors were assumed to be the same as their saturated counterpart. Gravimetric PHA content analysis was consistent with the PHA content determined using these response factors (data not shown). PHA extraction and purification methods were modified from Jiang et al. (2006). Extraction was performed in a soxhlet with chloroform, and twice purified by cold precipitation with methanol. 1H-NMR and 13C-NMR using a Brucker AMX-300 spectrometer (Brucker Biospin AG, Billerica, MA) were used to determine the chemical structure and monomer composition of purified PHAs. 10 mg of polymer sample was dried over Na2SO4 and re-dissolved in CDCl3.
Analysis of Thermal Properties
Differential scanning calorimetry (DSC, TA Instruments Q2000, New Castle, DE) of 5–10 mg of purified PHA samples were analyzed using a heat-cool-heat protocol at a heating rate of 10 °C/min from −60°C up to 200°C. Thermogravimetric analysis (TGA) were performed as previously described (Sharma et al., 2017) from 35°C to 500°C at 5°C under air, after determining that degradation was non-oxidative using argon gas.
Analysis of Molecular Weight
High-performance liquid chromatography (HPLC) using a Waters 1515 with Waters 2414 refractive index detector (Waters Corp., Milford, MA) using an Agilent 5 μm PLgel Mixed-C column and guard column were used to conduct gel permeation chromatography (GPC) at 30°C. A calibration curve was produced using Agilent EasiCal PS-1 polystyrene narrow molecular weight standards (Agilent Technologies, Santa Clara, CA). Purified PHA polymers were diluted to a concentration of 1.5 mg/mL in HPLC-grade chloroform and filter sterilized with 0.45 μm PTFE syringe filters (Fisher Scientific, Toronto, ON). Twenty microliter samples were injected into the HPLC-grade chloroform mobile phase with a flow rate of 1 mL/min.
Analysis of Mechanical Properties
Tensile strips were melt-cast using a “dog-bone” stainless steel form (Precision ADM, Winnipeg, MB) in accordance with ASTM D638-03 (50 × 10 × 1 mm, LxWxT) and conditioned as per D618-13 to a relative humidity of 63%. The plates of a Lloyd LS5 tensile tester were separated at a rate of 10 mm/min.
Results
Figure 1 displays the monomer compositions of mcl-PHAs produced from a variety of carbon substrates (Supplementary Table 1). Odd-numbered carbon length monomers were only observed from odd-numbered fatty acid substrates. 3-hydroxydecanoate (C10) was the dominant monomer from carbohydrates and the volatile fatty acids (VFAs) shorter than hexanoic acid. For the substrates in the range of hexanoic acid to nonanoic acid, the dominant monomer was the same length as the substrate. Even-numbered substrates of eight to eighteen carbons were all preferentially incorporated to 3-hydroxyoctanoate (C8) and C10 monomers. These results are consistent with the dominant monomers observed with P. putida KT2442 by which we can infer that fatty acids longer than valeric acid rely primarily upon the fatty acid β-oxidation pathway for PHA monomer production, while structurally-unrelated substrates rely solely on the fatty acid biosynthesis pathway (Huijberts et al., 1994).
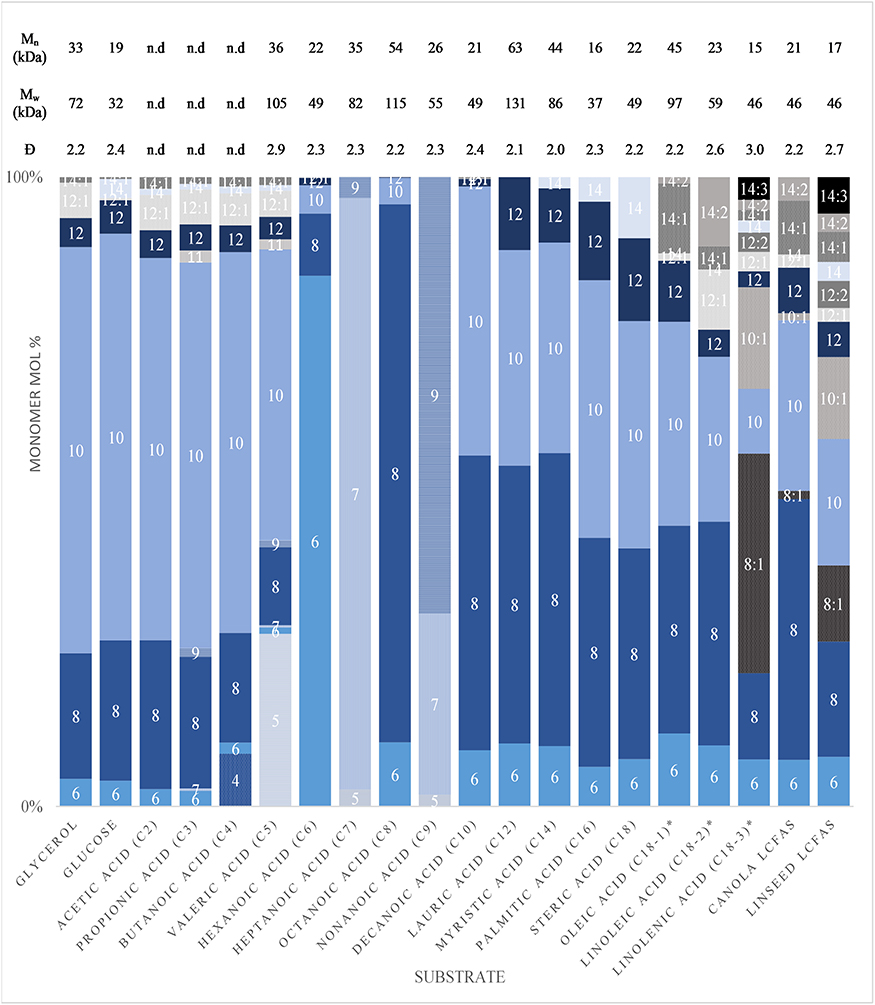
Figure 1. The 3-Hydroxyalkanoate monomer compositions and molecular weights produced by P. putida LS46 on varying carbon substrates.
Huijberts et al. (1994) suggested that the main source of longer monomers from hexanoic acid were from an elongation of hexanoic acid with acetyl-CoA, over a completely de novo fatty biosynthesis route. Here, the ratio of C8/C10 from hexanoic, and the inclusion of some 3-hydroxynonanoate (C9) from heptanoic acid agree with their findings. However, PHA production from propionic acid resulted in only 3.7 mol% of uneven-length monomers longer than the substrate, whereas the remaining 96.3 mol% of monomers were even-length, and longer than the substrate. A similar trend was observed by PHA production from valeric acid, except for the incorporation of 3-hydroxyvalerate (C5). PHA production from butanoic acid, with exception to the 8.5 mol% 3-hydroxybutyrate (C4) incorporated, resulted in monomer ratios similar to those obtained from glycerol and glucose. This indicates that the main route for PHA production from substrates shorter than hexanoic acid is by oxidation of the substrates to acetyl-CoA and subsequent de novo fatty acid biosynthesis route for PHA production.
Increased fatty acid substrate length resulted in an increased proportion of 3-hydroxydodecanoate (C12) and 3-hydroxytetradecanoate (C14). An increase in LCFA unsaturation resulted in an non-proportional increase of unsaturated 3-hydroxyacids. When fatty acid biosynthesis was required for PHA synthesis, small proportions of saturated C12 and C14 monomers along with monounsaturated 3-hydroxydodecenoic acid (C12:1) and 3-hydroxytetradecenoic acid (C14:1) were observed.
The molecular weight data corresponding to the mcl-PHAs produced by these substrates is also tabulated in Figure 1. Clear trends in PHA molecular weights based on carbon substrates could not be identified, despite the dispersity of molecular weights being consistent. Low molecular weights with higher dispersity were obtained from highly unsaturated mcl-PHAs.
The intracellular PHA content was highest from medium chain fatty acids (MCFAs) and lowest in substrates with high proportions of polyunsaturated fatty acids and steric acid, the later likely due to substrate mass transfer limitations (Table 1). The non-PHA cell mass (NPCM) ranged from 0.17 to 2.96 g/L where the lowest titers were obtained from MCFA substrates and the highest obtained from LCFAs. The higher NPCM values obtained from LCFAs could be partially explained by artificial increases due to contaminating substrate not removed during biomass processing, but these results are consistent with previous observation under microaerophilic conditions (Blunt et al., 2018a), which could impact these flask culturing conditions. PHAs produced from VFAs shorter than hexanoic acid resulted in an NPCM range of 0.25 g/L to 0.32 g/L and a PHA content range of 31.4% to 42.2%. The lower cell titer was due to one-tenth medium concentration required due to substrate toxicity.
The crystallinity of mcl-PHAs, as determined by melt enthalpy, was highest in polymers produced from octanoic acid (Table 2). Decreases in melting temperature, glass transition temperature and melt enthalpy were observed with longer fatty acid substrates and LCFAs lacked any crystallinity, consistent with previous reports (Ashby and Foglia, 1998). Polymers produced from carbohydrates were observed to crystallize into films, but with low crystallinity and lower glass transition temperature. The melting temperature for the mcl-PHA films ranges from 43.2 to 51.2°C. Upon a cooling and second heat cycles, no melting temperature was observed, unlike PHB and PHBV polymers which observe a similar melting behavior (data not shown). This is due to the slow crystallization behavior of mcl-PHAs (Marchessault and Yu, 2005). Interestingly, polymers produced from valeric acid displayed no crystallinity, and those from hexanoic acid resulted in very low enthalpy melting at 131.1°C. The glass transition temperature of mcl-PHA from hexanoic acid and the melt enthalpies of mcl-PHA from MCFAs are distinguishable from the other mcl-PHA thermograms (Figure 2). The behavior of mcl-PHA from flax above 70°C could be the result of thermal polymer cross-linking. The tensile strength (Table 2) increased with polymer crystallinity. Mcl-PHAs produced from carbohydrates tore easily resulting in low tensile strength and low elongation-at-break. The polymers produced from octanoic, nonanoic or decanoic acid produced highly elastic polymers with elongation-at-break between 162 and 184%. Octanoic acid polymers had a significantly higher tensile strength than nonanoic or decanoic acid polymers, and lower elongation-at-break percentages. The saturated LCFAs longer than decanoic acid could not be effectively scaled-up in a bioreactor for property analysis. These fatty acids are solid at cultivation temperatures and poor mass transfer results in significant foaming issues with air sparging causing removal of the substrate.
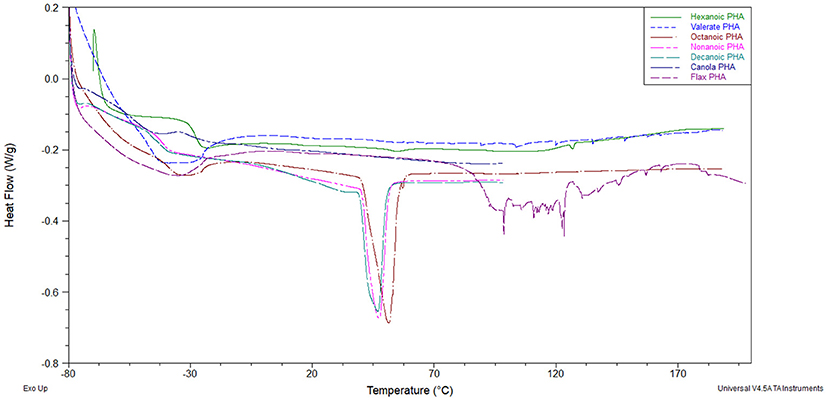
Figure 2. First heat thermogram overlay of mcl-PHAs obtained from various carbons substrates. Legend inset.
Discussion
Mcl-PHA production by Pseudomonas spp. across a variety of substrates exhibit a biosynthetic preference for the incorporation of C8, C9, and C10 monomer lengths. P. putida KT442 incorporated high C10 from glycerol and glucose substrates and very small fractions of longer monomers were observed with some unsaturation. From decanoate and C18 fatty acid substrates, C8 became the dominant monomer (Eggink et al., 1992). Similar results were obtained with other Pseudomonas spp. across the literature (Ashby and Foglia, 1998; Haba et al., 2007; Bassas et al., 2008; Song et al., 2008; Impallomeni et al., 2011), consistent with the substrate effect on mcl-PHA monomer composition observed in this study (Figure 1). Comparing the monomer compositions of various Pseudomonas sp. cultivated with oleic acid, the dominant monomer varied between C8 and C10 and significant variability is seen among the other monomers, thus demonstrating the difficulty in comparing properties across the literature for the increased variability attributed to strain and culturing conditions (Eggink et al., 1992; Ashby and Foglia, 1998; Solaiman et al., 2001; Fernández et al., 2005; Conte et al., 2006; Haba et al., 2007; Impallomeni et al., 2011).
The uneven-length monomer compositions from nonanoic acid and heptanoic acid (Figure 1) are consistent with those reported by other Pseudomonas sp. (Thakor et al., 2005; Sun et al., 2009; Wang et al., 2011). The monomer composition obtained from growth on undecenoic acid suggested a biosynthetic preference for C9 monomers (Hartmann et al., 2006, 2010). The high monomer mol% of C6 and C7 observed from hexanoic acid and heptanoic acid, respectively, is a result of the availability of those monomers from the β-oxidation pathway and the apparent minor role of the de novo fatty acid biosynthesis for PHA production from fatty acids longer than valeric acid.
Unsaturated positions were conserved in the polymer sidechains of sufficient length resulting in saturated, mono- or di-unsaturated C14 monomers from petroselinic (Δ-6), oleic (Δ-9) and linoleic (Δ-9,12) acids respectively. Mono-unsaturated C12 monomers were obtained from linoleic acid. (de Waard et al., 1993). Linolenic acid (Δ-9,12,15) retains the Δ-15 unsaturation down to C8 monomers (Casini et al., 1997). Due to the added olefin and the biosynthetic preference of C8 and C10 monomers, PHAs produced from linolenic acid contain a drastically higher unsaturation content (Figure 1). The theoretical ratio of unsaturated moieties is 10.3:2.9:1 for linolenic:linoleic:oleic acids based on the average monomer lengths obtained from P. putida LS46 using C18 LCFA substrates; and the observed ratio was 5.3:2.5:1 which was lowered due to the technical grades of the reagents having 70, 60, and 90% purity respectively.
The molecular weights observed from P. putida LS46 under these conditions were smaller but comparable with literature values (Figure 1, Table 3). The molecular weight of mcl-PHAs was highest when produced from saturated fatty acids such as octanoic acid and lowest from the unsaturated LCFAs. Higher double-bond content results in lower molecular weight. Unsaturated fatty acids are hypothesized to increase polymer chain termination which resulted in smaller number average molecular weights (Ashby et al., 1998a). The molecular weights were unaffected in mcl-PHAs produced with terminal double-bonds (de Koning et al., 1994; Schmid et al., 2007), indicating that chain termination could be caused by steric effects of having kinked sidechains.
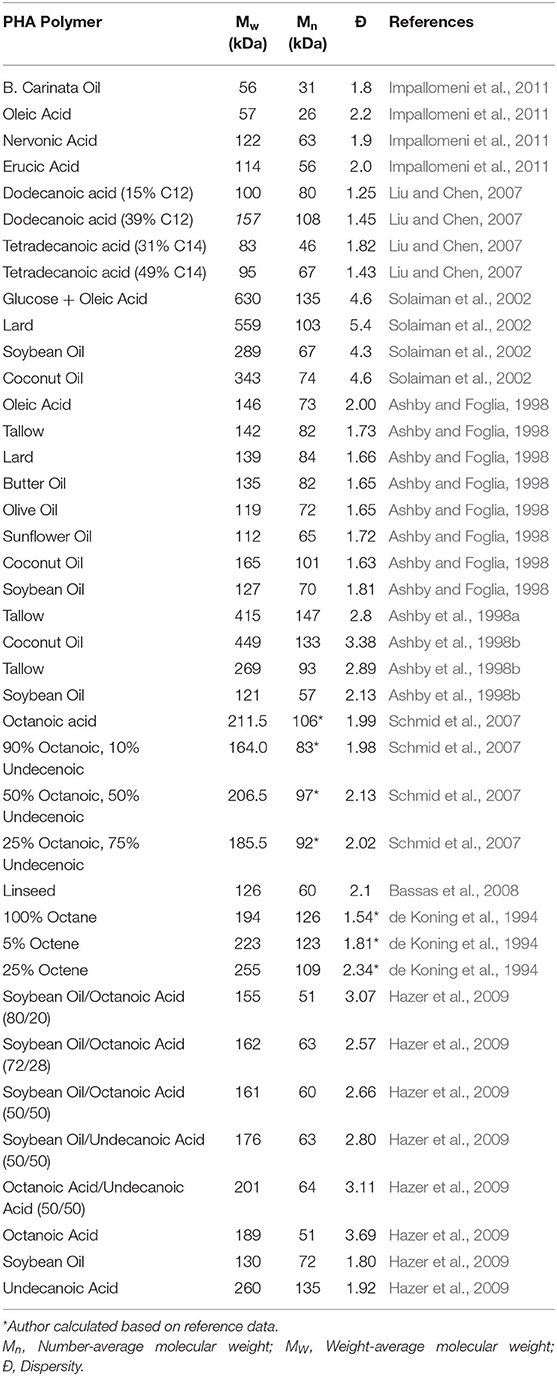
Table 3. Compilation of mcl-PHA average molecular weights produced from various substrates using Pseudomonas spp.
Several conclusions can be postulated about the substrate effect on the thermal data presented in Table 2 when also considering the associated molecular weights and monomer compositions (Figure 1) and comparing to results across the literature (Table 4). The crystallinity of PHAs depends on the length of the monomer sidechains. The crystal structure of scl-PHA chains is disrupted by the conformational requirements of longer mcl-PHA sidechains (Marchessault et al., 1990). The incorporation of C4 and C5 monomers by native P. putida LS46 remains low despite using butanoic or valeric acid, instead relying on the de novo fatty acid synthesis pathway for mcl-PHA production. The mcl-PHA obtained from valeric acid exhibited no crystallinity in contrast to the crystallinity observed from glucose, confirming that co-polymers of scl-PHA and mcl-PHA are not isomorphic. The C6 dominant mcl-PHA produced from hexanoic acid exhibited a very weak melt enthalpy consistent with the melting temperature of PHV, whereas previous reports indicated no melt point (Marchessault et al., 1990). Homopolymers of C6 and C7 PHA monomers exhibited no crystallinity in one report using mutant P. putida KT2442 (Wang et al., 2011), however exhibited melting temperatures of 59 and 45°C respectively using engineered E. coli. The x-ray diffraction pattern of a C6 homopolymer indicated an alternative crystal structure to either scl-PHA or mcl-PHA (Abe et al., 2012). Sidechains of 3-4 carbons may be too long for crystal structures with attractive forces between parallel back-bone chains, but too short to form any potential interactions of sidechain close-packing. Alternatively, the unique crystallization of these polymers may not be isomorphic with the wild-type co-monomer composition of mcl-PHA, and the discrepancy between C6 homopolymers.
Monomers of C6 act as effective internal plasticizers for scl-PHA copolymers where some disruption to crystallinity can improve elastic performance (Sudesh et al., 2000). Efforts to increase the monomer mol percentage of C8 or C9 from their respective fatty acids using acrylic acid as a β-oxidation inhibitor, subsequently reducing the presence of C6 or C7 monomers, resulted in increased melting temperatures and enthalpies (Jiang et al., 2012). As previously noted, Pseudomonas spp. predominantly produced C8 and C10 monomers from LCFAs. Mutant P. putida strains were produced that incorporated higher contents of C12 and C14 monomers resulting in mcl-PHAs with increased sidechain crystallization and elevated melting temperatures (Liu and Chen, 2007; Liu et al., 2011; Abe et al., 2012). The increased content of C12 and C14 monomers in mcl-PHAs produced from glucose or unsaturated LCFAs is unlikely to contribute to their reduced crystallinity, instead the olefin moieties are responsible for the reduction in crystallinity (Ashby and Foglia, 1998). Where terminal olefins did not affect the mcl-PHA molecular weights, increased unsaturation content resulted in drastic reduction in melt enthalpy (de Koning et al., 1994; Schmid et al., 2007).
The mechanical properties of mcl-PHAs were consistent with literature reports for similar substrates (Ashby et al., 1998a; Liu and Chen, 2007; Larrañaga et al., 2014). The tensile strips always broke near the clamps due to the localization of stress from the dog-bone mold which resulted in slightly dampened results. The tensile properties are reflective of the polymer crystallinity. Mcl PHAs are viscoelastic polymers compared to thermoplastic scl-PHAs associated with the greater motional freedom of sidechain crystallinity (Marchessault and Yu, 2005; Liu et al., 2011). Improved tensile properties can be achieved by manipulating the monomer composition of mcl-PHAs to contain saturated monomers of C8 or longer. Unsaturated monomers and short-length monomers reduce the crystallinity of mcl-PHAs and detract from the viscoelastic film properties. On the other hand, the inclusion of these monomers may be preferential for alternative applications. Mcl-PHAs of low crystallinity are tacky and may find application as biodegradable adhesives (Madison and Huisman, 1999). Unsaturated moieties have also been used to improve tensile properties or produce amphiphilic polymers by co-polymer grafting, cross-linking and through chemical modification (Hazer and Steinbüchel, 2007; Kim et al., 2007).
The results described herein further elucidates the relationship between carbon substrate, the biochemical pathways of Pseudomonas, and the resulting polymer properties. Mcl-PHAs of varying monomer compositions were obtained from a suite of carbon substrates indicating that crystallinity, measured by melt enthalpy, was dependent on monomer length and monomer saturation which ultimately determined tensile properties. The diversity in mcl-PHA monomer composition continues to increase through the genetic modification of PHA accumulating microorganisms, culturing conditions and chemical modification of PHAs. Careful consideration of the polymer properties beyond monomer composition is required to direct culturing methods and genetic modifications toward polymers tailored for diverse applications.
Data Availability Statement
The original contributions presented in the study are included in the article/Supplementary Materials, further inquiries can be directed to the corresponding author/s.
Author Contributions
CD contributed to experimental design, experimental execution, data analysis, and manuscript writing. WB contributed to experimental design, laboratory assistance, and manuscript review. PS contributed P. putida LS46 and laboratory assistance. SL contributed laboratory infrastructure and experimental design. NC and DL contributed funding, laboratory infrastructure, intellectual input and manuscript review. All authors contributed to the article and approved the submitted version.
Funding
This study was funded by Genome Canada, through the Genome Applications and Partnership Program (GAPP), and the Natural Sciences and Engineering Research Council (NSERC) of Canada through a Collaborative Research and Development (CRD) grant with Minto BioProducts Ltd. as the industrial partner (Grant Number CRDPJ-490630-15).
Conflict of Interest
The authors declare that this study received funding from Minto BioProducts Ltd., of Minto, Manitoba Canada. This company provided both cash and in-kind contributions to the research, and its primary role in the research was to provide access to a large-scale fermentation facility for production of larger amounts of PHA polymers.
The authors declare that the research was conducted in the absence of any commercial or financial relationships that could be construed as a potential conflict of interest.
Supplementary Material
The Supplementary Material for this article can be found online at: https://www.frontiersin.org/articles/10.3389/fbioe.2020.617489/full#supplementary-material
Supplementary Table 1. The 3-Hydroxyalkanoate monomer compositions produced by P. putida LS46 on varying carbon substrates.
References
Abe, H., Ishii, N., Sato, S., and Tsuge, T. (2012). Thermal properties and crystallization behaviors of medium-chain-length poly(3-hydroxyalkanoate)s. Polymer 53, 3026–3034. doi: 10.1016/j.polymer.2012.04.043
Anderson, A., and Dawes, E. (1990). Occurrence, metabolism, metabolic role, and industrial uses of bacterial polyhydroxyalkanoates. Microbiol. Rev. 54, 450–472. doi: 10.1128/MR.54.4.450-472.1990
Ashby, R. D., Cromwick, A. M., and Foglia, T. A. (1998a). Radiation crosslinking of a bacterial medium-chain-length poly(hydroxyalkanoate) elastomer from tallow. Int. J. Biol. Macromol. 23, 61–72. doi: 10.1016/S0141-8130(98)00034-8
Ashby, R. D., and Foglia, T. A. (1998). Poly(hydroxyalkanoate) biosynthesis from triglyceride substrates. Appl. Microbiol. Biotechnol. 49, 431–437. doi: 10.1007/s002530051194
Ashby, R. D., Foglia, T. A., Liu, C., and Hampson, J. W. (1998b). Improved film properties of radiation-treated medium-chain-length poly(hydroxyalkanoates). Biotechnol. Lett. 20, 1047–1052.
Bassas, M., Diaz, J., Rodriguez, E., Espuny, M. J., Prieto, M. J., and Manresa, A. (2008). Microscopic examination in vivo and in vitro of natural and cross-linked polyunsaturated mclPHA. Appl. Microbiol. Biotechnol. 78, 587–596. doi: 10.1007/s00253-008-1350-4
Blunt, W., Dartiailh, C., Sparling, R., Gapes, D., Levin, D. B., and Cicek, N. (2018a). Carbon flux to growth or polyhydroxyalkanoate synthesis under microaerophilic conditions is affected by fatty acid chain-length in Pseudomonas putida LS46. Appl. Microbiol. Biotechnol. 102, 6437–6449. doi: 10.1007/s00253-018-9055-9
Blunt, W., Levin, D. B., and Cicek, N. (2018b). Bioreactor operating strategies for improved polyhydroxyalkanoate (PHA) productivity. Polymers 10:1197. doi: 10.3390/polym10111197
Brandl, H., Gross, R., a Lenz, R. W., and Fuller, R. C. (1988). Pseudomonas oleovorans as a source for novel poly(β-hydroxyalkanoates) for potential applications as biodegradable polyesters. Appl. Environ. Microbiol. 54, 1977–1982. doi: 10.1128/AEM.54.8.1977-1982.1988
Casini, E., De Rijk, T. C., De Waard, P., and Eggink, G. (1997). Synthesis of poly(hydroxyalkanoate) from hydrolyzed linseed oil. J. Environ. Polym. Degrad. 5, 153–158.
Chanprateep, S. (2010). Current trends in biodegradable polyhydroxyalkanoates. J. Biosci. Bioeng. 110, 621–632. doi: 10.1016/j.jbiosc.2010.07.014
Chen, G.-Q. (2010). Industrial Production of PHA. Plast. from Bact. Nat. Funct. Appl. 14, 121–132. doi: 10.1007/978-3-642-03287-5_6
Conte, E., Catara, V., Greco, S., Russo, M., Alicata, R., Strano, L., et al. (2006). Regulation of polyhydroxyalkanoate synthases (phaC1 and phaC2) gene expression in Pseudomonas corrugata. Appl. Microbiol. Biotechnol. 72, 1054–1062. doi: 10.1007/s00253-006-0373-y
de Koning, G. J. M., van Bilsen, H. M. M., Lemstra, P. J., Hazenberg, W., Witholt, B., Preusting, H., et al. (1994). A biodegradable rubber by crosslinking poly(hydroxyalkanoate) from Pseudomonas oleovorans. Polymer 35, 2090–2097. doi: 10.1016/0032-3861(94)90233-X
de Waard, P., van der Wal, H., Huijberts, G. N., and Eggink, G. (1993). Heteronuclear NMR analysis of unsaturated fatty acids in poly(3-hydroxyalkanoates). Study of β-oxidation in Pseudomonas putida. J. Biol. Chem. 268, 315–319.
Eggink, G., de Waard, P., and Huijberts, G. N. M. (1992). The role of fatty acid biosynthesis and degradation in the supply of substrates for poly(3-hydroxyalkanoate) formation in Pseudomonas putida. FEMS Microbiol. Lett. 103, 159–163. doi: 10.1111/j.1574-6968.1992.tb05833.x
Favaro, L., Basaglia, M., and Casella, S. (2019). Improving polyhydroxyalkanoate production from inexpensive carbon sources by genetic approaches: a review. Biofuels Bioprod. Biorefining 13, 208–227. doi: 10.1002/bbb.1944
Fernández, D., Rodríguez, E., Bassas, M., Viñas, M., Solanas, A. M., Llorens, J., et al. (2005). Agro-industrial oily wastes as substrates for PHA production by the new strain Pseudomonas aeruginosa NCIB 40045: effect of culture conditions. Biochem. Eng. J. 26, 159–167. doi: 10.1016/j.bej.2005.04.022
Haba, E., Vidal-Mas, J., Bassas, M., Espuny, M. J., Llorens, J., and Manresa, A. (2007). Poly 3-(hydroxyalkanoates) produced from oily substrates by Pseudomonas aeruginosa 47T2 (NCBIM 40044): effect of nutrients and incubation temperature on polymer composition. Biochem. Eng. J. 35, 99–106. doi: 10.1016/j.bej.2006.11.021
Hartmann, R., Hany, R., Pletscher, E., Ritter, A., Witholt, B., and Zinn, M. (2006). Tailor-made olefinic medium-chain-length poly[(R)-3-hydroxyalkanoates] by Pseudomonas putida GPo1: batch vs. chemostat production. Biotechnol. Bioeng. 93, 737–746. doi: 10.1002/bit.20756
Hartmann, R., Hany, R., Witholt, B., and Zinn, M. (2010). Simultaneous biosynthesis of two copolymers in Pseudomonas putida GPO1 using a two-stage continuous culture system. Biomacromolecules 11, 1488–1493. doi: 10.1021/bm100118t
Hazer, B., and Steinbüchel, A. (2007). Increased diversification of polyhydroxyalkanoates by modification reactions for industrial and medical applications. Appl. Microbiol. Biotechnol. 74, 1–12. doi: 10.1007/s00253-006-0732-8
Hazer, D. B., Hazer, B., and Kaymaz, F. (2009). Synthesis of microbial elastomers based on soybean oily acids. Biocompatibility studies. Biomed. Mater. 4:035011. doi: 10.1088/1748-6041/4/3/035011
Huijberts, G. N. M., Eggink, G., De Waard, P., Huisman, G. W., and Witholt, B. (1994). 13C Nuclear magnetic resonance studies of Pseudomonas putida fatty acid metabolic routes involved in poly(3-Hydroxyalkanoate) synthesis. Synthesis 176, 1661–1666. doi: 10.1128/JB.176.6.1661-1666.1994
Impallomeni, G., Ballistreri, A., Carnemolla, G. M., Guglielmino, S. P. P., Nicolò, M. S., and Cambria, M. G. (2011). Synthesis and characterization of poly(3-hydroxyalkanoates) from Brassica carinata oil with high content of erucic acid and from very long chain fatty acids. Int. J. Biol. Macromol. 48, 137–145. doi: 10.1016/j.ijbiomac.2010.10.013
Jiang, G., Hill, D. J., Kowalczuk, M., Johnston, B., Adamus, G., Irorere, V., et al. (2016). Carbon sources for polyhydroxyalkanoates and an integrated biorefinery. Int. J. Mol. Sci. 17:1157. doi: 10.3390/ijms17071157
Jiang, X., Ramsay, J. A., and Ramsay, B. A. (2006). Acetone extraction of mcl-PHA from Pseudomonas putida KT2440. J. Microbiol. Methods 67, 212–219. doi: 10.1016/j.mimet.2006.03.015
Jiang, X., Sun, Z., Marchessault, R. H., Ramsay, J. A., and Ramsay, B. A. (2012). Biosynthesis and properties of medium-chain-length polyhydroxyalkanoates with enriched content of the dominant monomer. Biomacromolecules 13, 2926–2932. doi: 10.1021/bm3009507
Kim, D., Kim, H., Chung, M., and Rhee, Y. H. (2007). Biosynthesis, modification, and biodegradation of bacterial medium-chain-length polyhydroxyalkanoates. J. Microbiol. 45, 87–97.
Larrañaga, A., Fernández, J., Vega, A., Etxeberria, A., Ronchel, C., Adrio, J. L., et al. (2014). Crystallization and its effect on the mechanical properties of a medium chain length polyhydroxyalkanoate. J. Mech. Behav. Biomed. Mater. 39, 87–94. doi: 10.1016/j.jmbbm.2014.07.020
Liu, Q., Luo, G., Zhou, X. R., and Chen, G. Q. (2011). Biosynthesis of poly(3-hydroxydecanoate) and 3-hydroxydodecanoate dominating polyhydroxyalkanoates by β-oxidation pathway inhibited Pseudomonas putida. Metab. Eng. 13, 11–17. doi: 10.1016/j.ymben.2010.10.004
Liu, W., and Chen, G. Q. (2007). Production and characterization of medium-chain-length polyhydroxyalkanoate with high 3-hydroxytetradecanoate monomer content by fadB and fadA knockout mutant of Pseudomonas putida KT2442. Appl. Microbiol. Biotechnol. 76, 1153–1159. doi: 10.1007/s00253-007-1092-8
Madison, L. L., and Huisman, G. W. (1999). Metabolic engineering of poly(3-hydroxyalkanoates): from DNA to plastic. Microbiol. Mol. Biol. Rev. 63, 21–53. doi: 10.1128/MMBR.63.1.21-53.1999
Marchessault, R., and Yu, G. (2005). Crystallization and material properties of polyhydroxyalkanoates. Biopolym. Online 79, 157–166. doi: 10.1002/3527600035.bpol3b07
Marchessault, R. H., Monasterios, C. J., Morin, F. G., and Sundararajan, P. R. (1990). Chiral poly(β-hydroxyalkanoates): an adaptable helix influenced by the alkane side-chain. Int. J. Biol. Macromol. 12, 158–165. doi: 10.1016/0141-8130(90)90068-L
Mozejko, J., and Ciesielski, S. (2014). Pulsed feeding strategy is more favorable to medium-chain-length polyhydroxyalkanoates production from waste rapeseed oil. Biotechnol. Prog. 30, 1243–1246. doi: 10.1002/btpr.1914
Ramsay, B. A., Lomaliza, K., Chavarie, C., Dube, B., Bataille, P., and Ramsay, J. A. (1990). Production of poly-(β-hydroxybutyric-Co-β-hydroxyvaleric) acids. Appl. Environ. Microbiol. 56, 2093–2098. doi: 10.1128/AEM.56.7.2093-2098.1990
Schmid, M., Ritter, A., Grubelnik, A., and Zinn, M. (2007). Autoxidation of medium chain length polyhydroxyalkanoate. Biomacromolecules 8, 579–584. doi: 10.1021/bm060785m
Sharma, P., Munir, R., Blunt, W., Dartiailh, C., Cheng, J., Charles, T., et al. (2017). Synthesis and physical properties of polyhydroxyalkanoate polymers with different monomer compositions by recombinant Pseudomonas putida LS46 expressing a novel PHA SYNTHASE (PhaC116) enzyme. Appl. Sci. 7:242. doi: 10.3390/app7030242
Sharma, P. K., Fu, J., Cicek, N., Sparling, R., and Levin, D. B. (2012). Kinetics of medium-chain-length polyhydroxyalkanoate production by a novel isolate of Pseudomonas putida LS46. Can. J. Microbiol. 58, 982–989. doi: 10.1139/w2012-074
Solaiman, D. K. Y., Ashby, R. D., and Foglia, T. A. (2001). Production of polyhydroxyalkanoates from intact triacylglycerols by genetically engineered Pseudomonas. Appl. Microbiol. Biotechnol. 56, 664–669. doi: 10.1007/s002530100692
Solaiman, D. K. Y., Ashby, R. D., and Foglia, T. A. (2002). Physiological characterization and genetic engineering of Pseudomonas corrugata for medium-chain-length polyhydroxyalkanoates synthesis from triacylglycerols. Curr. Microbiol. 44, 189–195. doi: 10.1007/s00284-001-0086-5
Song, J. H., Jeon, C. O., Choi, M. H., Yoon, S. C., and Park, W. (2008). Polyhydroxyalkanoate (PHA) production using waste vegetable oil by Pseudomonas sp. strain DR2. J. Microbiol. Biotechnol. 18, 1408–1415.
Steinbüchel, A., and Valentin, H. E. (1995). Diversity of bacterial polyhydroxyalkanoic acids. FEMS Microbiol. Lett. 128, 219–228. doi: 10.1016/0378-1097(95)00125-O
Sudesh, K., Abe, H., and Doi, Y. (2000). Synthesis, structure and properties of polyhydroxyalkanoates: biological polyesters. Prog. Polym. Sci. 25, 1503–1555. doi: 10.1016/S0079-6700(00)00035-6
Sun, Z., Ramsay, J. A., Guay, M., and Ramsay, B. A. (2009). Carbon-limited fed-batch production of medium-chain-length polyhydroxyalkanoates from nonanoic acid by Pseudomonas putida KT2440. Appl. Microbiol. Biotechnol. 82, 657–662. doi: 10.1007/s00253-008-1785-7
Thakor, N., Trivedi, U., and Patel, K. C. (2005). Biosynthesis of medium chain length poly(3-hydroxyalkanoates) (mcl-PHAs) by Comamonas testosteroni during cultivation on vegetable oils. Bioresour. Technol. 96, 1843–1850. doi: 10.1016/j.biortech.2005.01.030
Keywords: polyhydroxyalkanoates (PHAs), Pseudomonas putida, microbial metabolism, subunit composition, crystallinity, tensile strength, thermal properties
Citation: Dartiailh C, Blunt W, Sharma PK, Liu S, Cicek N and Levin DB (2021) The Thermal and Mechanical Properties of Medium Chain-Length Polyhydroxyalkanoates Produced by Pseudomonas putida LS46 on Various Substrates. Front. Bioeng. Biotechnol. 8:617489. doi: 10.3389/fbioe.2020.617489
Received: 14 October 2020; Accepted: 21 December 2020;
Published: 21 January 2021.
Edited by:
Susana Rodriguez-Couto, Independent Researcher, Vigo, SpainReviewed by:
Takeharu Tsuge, Tokyo Institute of Technology, JapanElena Dimitrova Vassileva, Sofia University, Bulgaria
Copyright © 2021 Dartiailh, Blunt, Sharma, Liu, Cicek and Levin. This is an open-access article distributed under the terms of the Creative Commons Attribution License (CC BY). The use, distribution or reproduction in other forums is permitted, provided the original author(s) and the copyright owner(s) are credited and that the original publication in this journal is cited, in accordance with accepted academic practice. No use, distribution or reproduction is permitted which does not comply with these terms.
*Correspondence: David B. Levin, David.Levin@umanitoba.ca
†Present address: Warren Blunt, Aquatic and Crop Resource Development, National Research Council Canada, Montréal, QC, Canada