The Aversion Function of the Limbic Dopaminergic Neurons and Their Roles in Functional Neurological Disorders
- 1Institute of Brain and Psychological Sciences, Sichuan Normal University, Chengdu, China
- 2Department of Psychology, Jiangsu University Medical School, Zhenjiang, China
- 3Children’s Hospital of Nanjing Medical University, Nanjing, China
- 4School of Foreign Languages, China University of Geosciences, Wuhan, China
- 5Department of Neurology, Lianyungang Hospital of Chinese Medicine, Affiliated Hospital of Nanjing University of Chinese Medicine, Nanjing, China
- 6Department of Surgery, Texas A&M University College of Medicine, Temple, TX, United States
The Freudian theory of conversion suggested that the major symptoms of functional neurological disorders (FNDs) are due to internal conflicts at motivation, especially at the sex drive or libido. FND patients might behave properly at rewarding situations, but they do not know how to behave at aversive situations. Sex drive is the major source of dopamine (DA) release in the limbic area; however, the neural mechanism involved in FND is not clear. Dopaminergic (DAergic) neurons have been shown to play a key role in processing motivation-related information. Recently, DAergic neurons are found to be involved in reward-related prediction error, as well as the prediction of aversive information. Therefore, it is suggested that DA might change the rewarding reactions to aversive reactions at internal conflicts of FND. So DAergic neurons in the limbic areas might induce two major motivational functions: reward and aversion at internal conflicts. This article reviewed the recent advances on studies about DAergic neurons involved in aversive stimulus processing at internal conflicts and summarizes several neural pathways, including four limbic system brain regions, which are involved in the processing of aversion. Then the article discussed the vital function of these neural circuits in addictive behavior, depression treatment, and FNDs. In all, this review provided a prospect for future research on the aversion function of limbic system DA neurons and the therapy of FNDs.
Introduction
Functional neurological disorders (FNDs) are a common mental disorder (Ludwig et al., 2018). FND is a medical condition in which there is a problem with the functioning of the brain, rather than a structural disease. The exact prevalence of FND is unknown; research suggests that FND is the second most common reason for a neurological outpatient visit after headache/migraine, accounting for one-sixth of diagnoses (Voon et al., 2016). This means that FND is as common as multiple sclerosis or Parkinson’s disease (PD). FND was firstly known as conversion disorder, which was introduced by Breuer and Freud (1895–1995), who suggested that FNDs are due to the contradiction in the affective idea, which is converted into a somatic phenomenon. The Freudian theory of conversion proposed that the major symptoms of FND are due to inhibition of internal motivation, especially the sex drive or libido. FND patients always try to hide their internal motivations and always behave like acting in a play. FND patients might behave properly at happy situations; but they do not behave properly at aversive situations, when they are trying to hide their emotions. Sex drive is the major source of dopamine (DA) release in the brain; however, how DA in the limbic area is involved in FND is not clear. DA has been known as a reward neurotransmitter in the brain, and a large number of studies have shown that the secretion of DA in the limbic area has an inseparable relationship with joy (Gu et al., 2015). Since the 1960s, a great number of studies have confirmed the vital role of DA in the process of reward and motivation (Fouriezos and Wise, 1976; Corbett and Wise, 1980), so DA has gradually become a synonym for joy (Wang F. et al., 2020). However, what happens to the DA at the internal conflict for FND patients?
Schultz (Schultz et al., 1997) and others put forward the viewpoint of reward prediction error for DA in the 1990s, which induced most of the studies about dopaminergic (DAergic) neurons focusing on reward prediction error in the following 20 years. Meanwhile, there are an increasing number of studies investigating the neural circuitry of reward prediction error during this period. Brain regions such as the ventral tegmental area (VTA), substantia nigra (SN) pars compacta (SNc) (He et al., 2021), nucleus accumbens (NAc), striatum, lateral hypothalamus (LHa), and lateral habenula (LHb) have been found to be critically associated with the prediction error for rewards (Bromberg-Martin et al., 2010; Morales and Margolis, 2017). The most interesting thing is that the DAergic neurons are not only involved in reward-related prediction error, but they are also in the prediction of aversive information (Matsumoto and Hikosaka, 2009). Therefore, DA might work for both rewarding and aversive predictions, and DAergic neurons might induce two main motivational functions: reward and aversion at internal conflicts (Bromberg-Martin et al., 2010).
There have been many reports about the role of DA in reward, so this article only discusses the aversive function of DAergic neurons and their neural circuits. First, we briefly explain the role of DA neurons in the limbic system and the possible aversion mechanisms for FND. Then we review the neural circuits that limbic system DA neurons participate in. Finally, in order to better understand the neural mechanisms of aversion, we summarize the shortcoming of current research and recommend future studies, to provide new ideas for neuropsychiatric diseases, such as addiction, depression, and FND.
Aversion Function of Dopamine
Historically, FND has traditionally been viewed as an entirely psychological disorder in which repressed psychological stress or trauma gets “converted” into a physical symptom. This is where the term “conversion disorder” comes from. Psychological disorders and stressful life events, both recent and in childhood, may be risk factors for developing the condition in some patients, but they rarely provide a full explanation for the cause of the condition and are absent in many patients. Modern theories propose that FND has many causes, especially the monoamine neurotransmitters (Liang et al., 2021).
Dopaminergic neurons synthesize and release DA to a large area of the brain and change the emotional states of the whole brain (Morales and Margolis, 2017), including positive and negative reinforcement, decision making, and motivation (Adcock et al., 2006; Brischoux et al., 2009). It is revealed that DA is vital to the establishment of memory relevant to the search for reward motivation and reward cues, according to Dalley et al. (2005), and for the maintenance and promotion of action (i.e., motivation). However, the function of DA motivation was thought to be limited to approaching motivation or rewarding behavior. With the technological advancement, such as microdialysis methods, voltammetry method, and other electrophysiological methods, further studies (Salamone and Correa, 2012) found that DAergic neurons are also found to be involved non-reward events (Pezze and Feldon, 2004; Lisman and Grace, 2005; Redgrave and Gurney, 2006); for example, some researchers believe that DAergic neurons are also involved in the processing of other non-reward signals related to surprise, novelty, specificity, and even aversion. Surprisingly, DA has been shown to be involved in the opposite processes of reward, the aversion, which is also crucial for individual’s adaptation to environmental changes and cognitive processes such as learning and memory (Pignatelli and Bonci, 2015).
The pursuit of reward and the avoidance of punishment are two fundamental forces that drive animal’s behavior (Hu, 2016). Both Pavlov’s classical conditioned reflex and Skinner’s operational conditioned reflex have suggested that the pursuit of reward and the avoidance of punishment is beneficial evolutionally (Roy et al., 2014). Reward invokes approaching behaviors and induces satisfaction, which ultimately leads to behavior reinforcement, while an aversive stimulus produces negative emotions, including dislike and fear, resulting in avoidance and reducing the production of similar behaviors (Salamone and Correa, 2012; Hu, 2016).
Aversion
Reward and aversion are two major important components for motivational control (Bromberg-Martin et al., 2010). DAergic neurons in the limbic system have been shown to be involved in these processes and cooperate with different neural circuits to coordinate the downstream cognitive structure and to control the motivational behavior (Bromberg-Martin et al., 2010; Fagan et al., 2020). The term “motivation” is widely used for reward (Salamone and Correa, 2012), and it is defined by the famous German philosopher Schopenhauer (1999) as “choose, seize, and even seek out the means of satisfaction.” Early psychologists such as Wundt, James, and Freud have all talked about motivation. However, motivation also involves avoiding, so a broad definition of motivation is the mental process, or an inner psychological process that is involved in approaching or avoiding a target. Young (1961) defined motivation as “the process of arousing actions, sustaining the activity in progress, and regulating the pattern of activity.” Peng (2012) defined motivation as an inner psychological process or internal motivation, guided, inspired, and maintained by a goal or object. The latest definition of motivation by Salamone (1992) is a series of processes by which organisms regulate the possibility, proximity, and availability of stimuli. Due to the phased characteristics of motivation, Salamone (2010) further defined stages of motivation as “desire, preparation, action, approach or seek.” FND patients might show some abnormal behaviors when the motivation was inhibited.
Given that motivation is a psychological process that consists of a series of phased psychological processes, including reward and aversion, reward motivation as approaching or enjoying an object or event induces positive emotion or pleasure (Schultz, 2010). According to Berridge and Kringelbach (2015), a similar definition of reward is that rather than a single process, reward contains several psychological components, namely, liking, wanting, and learning. On the contrary, aversion is convinced as “a stimulus that one always avoids or prevents.” This definition soon met negative comments, and it is criticized for failure to reflect the characteristics of learning reinforcement of aversive stimuli (Murphy, 2008). Aversion learning includes two processes: (1) operant aversion to learning (1a), punishment learning (1b), and active avoidance of learning; and (2) aversive Pavlovian classical conditioning. From the perspective of biological evolution, aversion is born to sustain survival (Jean-Richard-Dit-Bressel et al., 2018). In other words, aversion is an inner feeling generated by an individual to avoid the potentially harmful stimuli (toxin, instead of predator and threat) to the body. Aversion behavior might be the contrary of reward, which includes avoidance behavior (Verharen et al., 2020). Thus, FND patients might have problems especially at aversion behaviors: they can behave properly at rewarding behavior, but they do not know how to behave at aversive situations.
Of note, aversion should be differentiated from disgust, which is regarded as a basic emotion. Disgust and aversion are two common psychological concepts. As we discussed above, aversion derives from motivation; it is a kind of feelings opposed to reward. On the other hand, disgust is a kind of prototypical emotion, which originates from eating toxic foods and infected by pathogens and parasites (Berridge, 2018). From this perspective, the feeling of aversion is a complex emotion, which contains a series of basic emotions, such as disgust and fear (Chapman et al., 2009). However, both disgust and aversion can induce avoidance behavior.
Dopamine Responses to Aversive Stimuli
Even though DA was suggested to be the major neurotransmitter for reward (Salamone et al., 1993), recent studies show that various disgusting or stressful events also increased DA release or metabolism in the NAc (Davidson et al., 2004), which indicates that DAergic neurons in the limbic system may be involved in the processing of aversive stimuli (Abercrombie et al., 1989; Bradberry et al., 1991; Joseph et al., 2003; Barr et al., 2009; Fadok et al., 2009). Other scholars, however, believe that aversive stimuli belong to motivation valence, which usually induces low-level DA activities (Liu et al., 2008; Roitman et al., 2008). In addition, others suggested that this kind of DA activation is related to the processing of stimulus arousal attributes (i.e., alertness) rather than value (aversion). Further studies show that two mixed activation modes of DAergic neurons exist in the limbic system (Ventura et al., 2001).
They are coexisting high-level and low-level activation modes of DA release in different brain regions (Pascucci et al., 2007). In recent years, with the development of viral vector-based methods (e.g., cell type-specific and projection-specific electrophysiology, in vivo imaging, and optogenetics), researchers induce aversion in mice by exposing them to chronic or acute aversive stimuli (foot electric shock, foot shock, forced swimming test, hind paw injection of formalin, social frustration stress, and fear conditioned reflex) and by intraoral injection of the solution, which leads to oral and facial reactions (Berridge, 2000; Fadok et al., 2009; Lammel et al., 2012; Tye et al., 2013; Friedman et al., 2014). Results show that DAergic neurons can be divided into multiple subpopulations, which are responsible for the processing of different stimuli information. The DAergic neurons that are involved in processing of aversive stimuli are multiple subpopulations of midbrain DA neurons (Kim et al., 2016; Groessl et al., 2018; de Jong et al., 2019). For example, Stephan Lammel’s laboratory has been dedicated to the study of DA aversion function for many years; in their recent studies, they injected viruses into the brains of mice and measured the release of DA in different ventral striatal subregions of mice with fiber photometry under different experimental task conditions (aversion and reward conditioned reflex experiment, real-time place preference experiment, open field experiment, and approach-avoidance experiment. It is found that NAc DAergic neuron terminals in the ventral NAc medial shell (vNAcMed) was excited at aversive cues (de Jong et al., 2019). This result indicates that although most DAergic neurons in the NAc are excited by reward stimuli and reward cues, there is a cluster of DAergic neurons in the NAc that are excited at aversive stimuli and cues (Lammel et al., 2011, 2012, 2015; Yang et al., 2018; Cerniauskas et al., 2019; de Jong et al., 2019). Further studies show that during rewarding events and when aversion to toxic events decrease, DA activity in the dorsomedial NAc shell (dmNAc) increases. In contrast, the release of DA in the ventromedial NAc shell (vmNAc) was increased by both rewarding and aversive stimuli, while the DA-sensor signal in the central vmNAc (ceNAc) and ventrolateral NAc shell (vlatNAc) showed complex dynamics (Yuan et al., 2019). The same result was also found in other projection terminals of VTA DA and SN DA including the medial prefrontal cortex (mPFC) (Kim et al., 2016; Ellwood et al., 2017; Morales and Margolis, 2017; Vander Weele et al., 2018), amygdala (Fadok et al., 2009; Lutas et al., 2019), and dorsolateral and caudal striata (Lerner et al., 2015; Menegas et al., 2015). In addition to the traditional DAergic neuron aggregation areas such as the VTA and SN, dorsal raphe nucleus (DRN), and midbrain periaqueductal gray matter (PAG) have recently been found to have relatively sparse DA neuronal activities at aversive stimulation (Cardozo Pinto et al., 2019); however, there are really some DAergic neurons in these regions, which have been reported to be activated in response to aversive stimuli (Matthews et al., 2016; Groessl et al., 2018; Verharen et al., 2020). Therefore, the above research shows that some special subtypes of DAergic neurons exist in multiple brain areas of the limbic system (such as the striatum, which includes the NAc, VTA, and SN) and show excitement when aversive stimuli and cues appear.
However, some researchers still hold opposite views on whether DAergic neurons are involved in the processing of aversive stimuli. For example, Schultz doubts about the aversive function of DAergic neurons; instead, he proposed reward prediction error (Schultz, 2010). He suggested that that majority of DAergic neurons in the limbic system showed excitement toward reward stimuli and inhibition toward aversive ones. According to Schultz, DAergic neurons are excited by the learning process about both rewarding and aversive stimuli, while they make no response to aversion itself. In some studies, however, the response of DAergic neurons in the limbic system to aversive stimuli is different studies with microdialysis, rapid scanning cyclic voltammetry, and electrophysiological methods (Roitman et al., 2008; Badrinarayan et al., 2012). Based on these conflict results, some researchers proposed other assumptions on the role of DAergic neurons in the limbic system in the processing of aversive stimuli (Verharen et al., 2020). These assumptions are as follows: (1) the aversive stimulus may reflect the physical attributes rather than aversion itself (Lammel et al., 2014; Menegas et al., 2018); (2) animals experience rewards or relief when aversive stimuli terminate (Brodsky and Lajoie, 2013); (3) a high-return environment may lead to excitement toward aversive stimuli (Matthews et al., 2016); and (4) DAergic neurons transmit safety signals when an animal successfully avoids nasty events (Luo et al., 2018). These four assumptions are indeed the symptoms of FND patients; however, further studies are needed to address these problems and to provide further evidence for the involvement of DAergic neurons in the limbic system in the processing of aversive stimuli, especially in FND patients.
Dopamine Receptors
The psychological effects of DA are mainly mediated by DA receptors, which are composed of five different but closely related G protein-coupled receptors, including D1, D2, D3, D4, and D5 DA receptors (Beaulieu and Gainetdinov, 2011). At first, Spano et al. (1978) found that only a subset of DA receptors are positively correlated with the activity of adenylyl cyclase (AC). Then DA receptors can be separated into D1-like (D1 and D5) and D2-like (D2, D3, and D4) receptors (Vallone et al., 2000; Beaulieu and Gainetdinov, 2011) based on the structure, pharmacology, biochemical characteristics, and downstream signaling pathways. D1-like receptors can enhance the activity of AC by activating Gαs/olf family and can promote the production of cAMP (response element-binding protein). However, D2-like receptors activate Gs/ol family and inhibit the activity of AC and the production of cAMP (Xia et al., 2019). The five DA receptors are different in distribution and function. D1 receptors (D1Rs) are expressed mainly in the SNc; in midbrain limbic and middle cerebral cortex areas, such as the caudate–putamen (striatum), NAc, SN, olfactory bulb, amygdala, and frontal cortex; and at lower levels in the hippocampus, cerebellum, thalamic areas, and hypothalamic areas (Beaulieu and Gainetdinov, 2011). D1Rs can mediate PFC and brain-derived neurotrophic factor (BDNF) to impact partial working memory and learning process (Sarinana et al., 2014). The activation of hippocampal dentate gyrus D1Rs promotes the representation of explicit contexts and closely correlated to semantic memory (Fan et al., 2010). Besides, D1Rs also play a vital role in locomotor activity, rewarding reinforcement, and motivation. D2 receptors (D2Rs) are mainly distributed in the striatum, NAc septi, olfactory tubercle, SN, VTA, hypothalamus, cortical areas, septum, amygdala, and hippocampus (Gerfen, 2000; Vallone et al., 2000; Seeman, 2006). D2Rs play an important role in controlling motor action (Fan et al., 2010). The activation of presynaptic D2-like receptors may reduce the release of DA and then cause the reduction of motor action, but the activation of postsynaptic receptors will promote locomotor activity. The different functions of presynaptic and postsynaptic D2-like receptors may be attributed to different subtypes. It should be noted that the splice variants of the D2 DA receptors, D2RL (D2R long) and D2RS (D2R short), seem to have different neuronal distributions, with D2RS being predominantly presynaptic and D2RL being postsynaptic (Usiello et al., 2000; Mei et al., 2009). Like D1Rs, D2Rs are also vital to motivation, but they have totally different functions. It is found that there are two types of neurons projected in the dorsal striatum with two types of DA receptors, with the first type representing D1Rs and projecting in direct pathway in the basal ganglion to promote body motion, which is called rewarding approach, and the second type represents D2Rs and projects in an indirect pathway to suppress the body motion, called aversion avoidance (Hikida et al., 2010; Kravitz et al., 2010). And Kravitz et al., 2010, 2012 used ontogenetic stimuli and found that D1-medium spiny neurons (D1-MSNs) selectively activate the D1-type MSNs in the dorsal striatum, promote locomotion, and induce persistent reinforcement. In contrast, activation of the D2-type MSNs suppressed locomotion and induced transient punishment (Kravitz et al., 2010, 2012). Thus, rewarding and aversive stimuli can activate different DA neurons with different receptors and lead to the approaching or avoiding behaviors. This indicates that the rewarding and aversive neurons can be separated by different receptors. However, Al-Hasani et al. (2015) find that the activation of DAergic neurons in the ventral part of the NAc is mainly overlapped with D1-MSNs but reacts to aversive stimuli. Therefore, it is worthy further studying the different types of DAergic neurons with different DA receptors.
Although considerable progress has been made in the physiological function of D3, D4, and D5 receptors, the specific physiological functions of these receptors are yet unknown (Beaulieu and Gainetdinov, 2011), especially the function of DA neurons in rewarding and aversion. D3, D4, and D5 receptors are mainly expressed in the limbic system (Leriche et al., 2006; Rondou et al., 2010; Beaulieu and Gainetdinov, 2011). It is proved that D3 is vital to cocaine addiction (Zhang et al., 2017) and locomotion activity (Sibley, 1999). And the increase of D3 receptors (D3Rs) is closely related to tardive dyskinesia (TD) in a non-human primate model (Mahmoudi et al., 2014). Recent studies have shown that D4 receptors have the potential to improve mental disorders and cognitive functions (Guo et al., 2017). D5 receptor has beneficial effects on reward and novelty and on improving cognitive impairment (Leriche et al., 2006; Beaulieu et al., 2007; Mei et al., 2009; Shen et al., 2016). DA receptors also play a vital role in the treatment of many mental diseases, especially the FND, are targets of some psychiatric pharmaceuticals, and the target of many medications that are the main methods used to treat PD, restless legs syndrome, schizophrenia, bipolar disorder, and major depressive disorder, such as bromocriptine, pramipexole, ropinirole, chlorpromazine, risperidone, metoclopramide, brexpiprazole, and cariprazine (Pajonk, 2004; Bonuccelli et al., 2009; Fawcett et al., 2016; Kim et al., 2016; Klein et al., 2019).
Prediction Errors and Functional Neurological Disorder
The concept of reward prediction error proposed by Schultz et al. (1997) is regarded as a milestone in the study of DA function. Along with his colleagues, Schultz found that there is a significant relationship between the activation level of VTA DAergic neurons and the reward prediction before the appearance of stimuli. Later on, Gu et al. (2019b) deduced the famous reward prediction error formula through modeling:
which quantifies the relationship between DAergic neuron excitement and reward prediction error. That is to say, the joy at reward stimuli is determined not only by the amount of reward but also by individual’s expectation of the reward stimuli (Wang F. et al., 2020). Chances are that the joy of a fully expected big reward is less than that of an unexpected small reward. Correspondingly, DAergic neurons perform differently at expected errors (as expected, better than expected, and worse than expected). Rewards regarded as “expected” might incur tonic DA release, those as “better than expected” produce phase burst signals, and those as “worse than expected” generate suspension of activation of DAergic neurons. Bursts of action potentials induce more DA release in a specific projection area than spikes of the same number in the spaced action potential tissue (Pignatelli and Bonci, 2015). It is worth noting that DAergic neurons in the limbic system are traditionally believed not to be sensitive to aversion prediction errors, because the midbrain DAergic neurons might only process the information of reward prediction errors, while they make no response in accordance with the occurrence of aversive clues [conditioned stimuli (CSs)] (Fiorillo et al., 2013; McHugh et al., 2014).
However, a recent study showed a totally different result, in contrast to previous studies that showed that the VTA and SN in the traditional DA neuron aggregation area are not activated by aversion prediction errors (Mileykovskiy and Morales, 2011; Schultz, 2015), and even showed inhibitory response to aversion prediction cues (Ungless, 2004; Tan et al., 2012). However, related results show that midbrain DAergic neurons are distributed not only in the VTA and SN but also in the PAG and DRN (Dougalis et al., 2012). Despite sparse distribution in the VTA and SN, these DAergic neurons are highly likely to play an important role in aversion prediction errors according to the characteristics of neurons in these brain areas (Matthews et al., 2016; Cardozo Pinto et al., 2019). Groessl et al. (2018) investigated the activities of DAergic neurons at CSs (such as noise) and unconditioned stimuli (USs; such as foot shock). Results showed that PAG and DRN DAergic neurons are activated by the aversive stimuli clues (noise). These mice showed three kinds of prediction errors: as expected, worse than expected, and better than expected. It turns out that the DAergic neurons of the PAG and DRN produced a potential activity similar to that of the DAergic neurons of the VTA during the reward prediction error. In other words, aversion regarded as “as expected” will incur tonic release; reward as “worse than expected” will incur a phase burst signal; and aversion as “as expected” will incur a suspension of activation. These results suggest that the DAergic neurons of the PAG and DRN are likely to be involved in the processing of aversive prediction errors.
Besides, human beings might also follow this principle in the process of decision making. Nobel Prize winner Professor Kahneman proposed in his classic loss aversion theory that human beings also follow the principle of seeking advantages and avoiding disadvantages in the process of decision making. Decision making under risk usually expresses different levels of aversion preferences and shows higher sensitivity to potential aversion than the same level of acquisition (Kahneman and Tversky, 1979). The involvement of the PAG in the processing of aversive prediction errors has been demonstrated in a functional magnetic resonance imaging (fMRI) study on the human brain (Roy et al., 2014). Roy et al. (2014) compared two different tests on aversion to avoid acquisition and degree of pain, and then they established two types of prediction errors. One is “all or nothing” and the other “continuous change.” Changes in relevant brain regions under three prediction errors (as expected, worse than expected, and better than expected) are recorded using fMRI. With Bayesian statistics processing, the results showed that the PAG is the key brain region of prediction error processing; at the same time, an important loop of aversive prediction error is identified: PAG–mPFC. Due to the intrusive nature of neuronal imaging (Verharen et al., 2020), it is not yet determined whether it is DAergic neurons that are activated by aversive prediction errors in the PAG. But with research results in mice by Groessl et al. (2018), it can be speculated that there exist a cluster of DAergic neurons in the PAG of animals that respond to aversive stimuli and engage in the processing of aversive prediction errors. The activation of aversive prediction errors in the human brain is likely to be incurred by a subgroup located in the area of DAergic neurons.
According to the description of the Diagnostic and Statistical Manual of Mental Disorders, fifth edition (DSM-5), FND is defined as a neurological disease with symptoms (such as motor and cognitive disorder) that cannot be explained by current neurological theories. When it comes to behavior research, FND patients are different in emotion and recognition from normal people, embodied by their more negative emotion and worse prediction (Pick et al., 2019). An fMRI study of FND patients found that the PAG activation increases when they face facial expressions, and the connection of the dorsolateral PFC (dlPFC) and insula increases according to an emotion-related study on them (Aybek et al., 2015; Espay et al., 2018; Szaflarski et al., 2018). Therefore, it is fair to say that the behavior and fMRI results are both strongly correlated with DAergic neurons in the PAG area. Accordingly, it is predicted that the abnormality of DAergic neurons in the PAG area is one of potential causes of FND. FND patients often show hysterical behaviors in stressful situations. FND patients tend to be more negative at loss and gain lower feelings of happiness from reward than healthy people. Consequently, they feel exhausted, indifferent, and even depressed, which appears to be consistent with symptoms of lacking DA (Logan and McClung, 2019; Xia et al., 2019; Liang et al., 2021). In conclusion, it is necessary to further explore the activation difference of DAergic neurons between FND patients and healthy people with predicting reward or aversion, so as to uncover the causes for FND.
Parkinson’s Disease and Functional Neurological Disorder
There are symptoms of movement disorders at the onset stage of both PD and FND, for example, tremors; and related studies have found that the activation patterns of the basal ganglia are abnormal in patients with both diseases (Lehn et al., 2016; Klein et al., 2019). One of the main input areas of the basal ganglia is the striatum. Ninety percent of neurons in the striatum are GABAergic spiny projection neurons (SPNs), which highly express D1Rs and D2Rs and control movement by DAergic projections (Gerfen and Surmeier, 2011). Nowadays, it has proved that PD is related to the abnormalities in the pathway (Bonuccelli et al., 2009; McKinley et al., 2019), and recent studies of FND have also found that motor conversion disorders in FND patients may be related to abnormalities in this area (Voon et al., 2010; Lehn et al., 2016). PD is a common neurodegenerative movement disorder (Klein et al., 2019), which can be mainly divided into two subtypes. The first type is tremor-dominant PD whose symptoms are mainly motor retardation, muscle rigidity, resting tremor, and posture and gait disorder. The second type is non-tremor-dominant PD, which usually progresses faster and has higher functional disability than tremor-dominant PD (Jankovic et al., 1990; Kalia and Lang, 2015). The common incidence of PD happens in the elderly over 60 years old, and the incidence rate is about 13 cases per 100,000. And the incidence rate of men is higher than that of women (Williams-Gray and Worth, 2016).
The main motor symptoms of PD are caused by the degeneration of SN DAergic neurons (Suárez et al., 2014), which mainly project in the dorsal striatum. The main function of the striatum is to evaluate the “action plans” produced by the cerebral cortex; to construct a movement diagram based on the sensory state, motivational state, and past experience; and then to transmit the evaluation to other basal ganglion nuclei (Zhai et al., 2019). At the receptor level, the abnormal regulation of the direct and indirect pathway spiny projection D1Rs and D2Rs in the dorsal stratum is the cause of the main motor symptoms of PD (Gerfen and Surmeier, 2011). It was suggested that D1Rs and D2Rs control the “direct pathway” and “indirect pathway” and promote or inhibit body movements, respectively (Xia et al., 2019). And it has been found that the excitability of D1 and D2 shifted in opposite directions in PD patients, causing an imbalance in the regulation of the thalamus movement (Gerfen and Surmeier, 2011). Meanwhile, some studies have shown that the lack of DA alters the induction of long-term plasticity at glutamatergic synapses, and the lack of D1R signal may cause the long-term bias of the direct pathway of glutamatergic synapses, but the lack of D2Rs signal can cause long-term potentiation (LTP) of direct pathway glutamatergic synapses (Mallet et al., 2006). Thus, the current treatment for PD is using drugs that increase DA concentration or directly stimulate DA receptors for symptomatic treatment.
Similarly, FND symptoms are characterized by physical symptoms without disease pathology, mainly manifested as tremor, myodystonia, and gait disorder (Voon et al., 2010). FND patients account for about 30% of neurological outpatients (Carson et al., 2003). FND patients also suffer more than other known mental illness patients, because the causes of FND are not yet clear. At the same time, severe FND can also cause physical disability (Pick et al., 2019). In addition to physical symptoms, FND patients often report a series of emotional dysfunctions, such as anxiety, depression, alexithymia, and/or affective regulation disorder (Brown and Reuber, 2016; Carson and Lehn, 2016). Recently, some researchers have begun to study the differences between FND patients and normal people from the perspective of emotional processing and put forward some hypotheses for the causes of FND from the perspective of emotion. For example, Voon et al. (2010) applied an fMRI in studying the difference in brain activation between normal subjects and FND patients in recognizing emotional faces. They found that there was no difference of the activation of the amygdala when normal people watch emotional faces (happiness, neutral, and fear). And further analysis has found a greater interaction between the right amygdala and the right supplementary motor area in FND patients compared with normal subjects (Voon et al., 2010), which indicates some abnormalities in the amygdala of FND patients. The basolateral amygdala receives the signals from the hippocampus and cerebral cortex and then projects to the dorsal and ventral striata. Meanwhile, the basolateral amygdala can also send projections to the central nucleus of the amygdala and the terminal striae of the lateral basilar nucleus (extended to the amygdala) and then project to the gray matter around the aqueduct, the LHa (autonomous responses), and the midbrain nucleus, such as the VTA (Lang and Davis, 2006). The DAergic neurons projected via both pathways are related to motor control (Zhai et al., 2019) and reward prediction errors (Schultz et al., 1997; Groessl et al., 2018). The greater functional connectivity of the marginal zone in FND patients affects the motor preparation zone, which may be a pathophysiological basis of FND (Voon et al., 2010). Schrag et al. (2013) have some similar findings. Anyway, the causes of FND are complicated, and FND may not be caused by a dysfunction of a single brain area. Therefore, future studies are needed to start with the interactions between emotion and motor brain area and to study the DAergic projection in different neural networks.
Neural Networks for Dopamine Projection
The limbic system has been called the emotional brain, which is located at the underside of the brain and the inner side of the brain [including the anterior cingulate cortex (ACC)], the lower corpus callosum, the hippocampal structures in the hippocampus and deep hippocampus, and some subcortical brain structures, including the thalami, VTA, and SN (Shen and Lin, 1993; He et al., 2009). The VTA and SN in the ventral side of the limbic system have long been considered as the main source of DA transmitters in the cerebral cortex and subcutaneous tissue (Bjorklund and Dunnett, 2007). This area is also involved the processing of reward prediction error calculation (Schultz et al., 1997), with gamma-aminobutyric acid (GABA) in the VTA regulating the amount of reward by inhibiting the activity of DAergic neurons (Roberts et al., 2021), thereby calculating the reward prediction error (Cohen et al., 2012). At the same time, some animal studies have indicated that there are multiple clusters of neurons in this region that are responsible for different motivational functions and may be involved in different neural circuits to process reward and aversive information (Lammel et al., 2011). However, most studies mainly focus on the reward function of DA in the limbic system, lacking studies on the aversion function and its neural circuits. In addition, some researchers even questioned the aversive function of DA neurons in the limbic system (Schultz, 2010).
Bromberg-Martin et al. (2010) proposed that DAergic neurons of the limbic system act on different motivational controls when they participate in different neural networks. Therefore, it has been a vital research direction in the related field to find the aversive circuit of DAergic neurons of the limbic system. In recent years, with the development of virus-tracking technology, some aversive circuits of DA have been discovered (Hu, 2016; Verharen et al., 2020). For example, some studies have reported four limbic system brain areas: the VTA, SN, PAG, and DRN. The next discussion about the aversive circuit of DAergic neurons in the limbic system will also focus on these brain areas.
The Aversion Function of Dopaminergic Neurons in the Ventral Tegmental Area
The VTA is an important nucleus for DAergic neurons, which play an important role in reward prediction error. Indeed, the mesolimbic DA system (refer to the DA pathway from VTA DAergic neurons to the NAc) has always been considered to play an important role in processing information about rewards, approaching behaviors and positive reinforcement (Saunders et al., 2018; Yuan et al., 2019). However, some researchers suggested that the DA release of neural circuits connected to DAergic neurons in this region is involved in the processing of aversive stimuli. For example, Lammel et al. (2011, 2012) discovered a circuit of VTA DAergic neurons processing aversive stimuli. Many new ways of studying have shed some light in this field, by injecting viruses and fluorescent tracers into the VTA of mice; applying conditioned place preference (CPP), conditioned place aversion (CPA), and open field tasks to these mice; and recording the activities of DAergic neurons in the VTA. And these results showed that there are two kinds of VTA DAergic pathways: the rostromedial tegmental nucleus (RMTg) → VTA DA → dorsal NAc pathway, which is mainly responsible for processing rewarding stimuli, and the other one is the LHb → VTA DA → mPFC pathway, which is involved in processing the aversive stimuli (Figure 1). Besides, there are studies that further reported several subgroups of DAergic neurons in the dorsal VTA, which are embedded in different circuits and participated in different behavioral functions. However, these pathways have been questioned by some later studies (Tian and Uchida, 2015; Tian et al., 2016); for example, Tian et al. (2016) showed that aversive stimulus processing function was not affected by VTA impairment, which suggested that the reaction is insensitive to the decline of the frequency of rewards. However, this aversive pathway is supported by many other studies (Pignatelli and Bonci, 2015; Hu, 2016). Alternatively, de Jong et al. (2019) reported another pathway for aversive processing associated with VTA DAergic neurons, which is LHb → RMTg → VTA DA → vNAcMed (Lerner et al., 2015). In all, these studies indicate that some DAergic neurons participate in the processing of aversive stimuli through the VTA as a start point projecting to different brain regions.
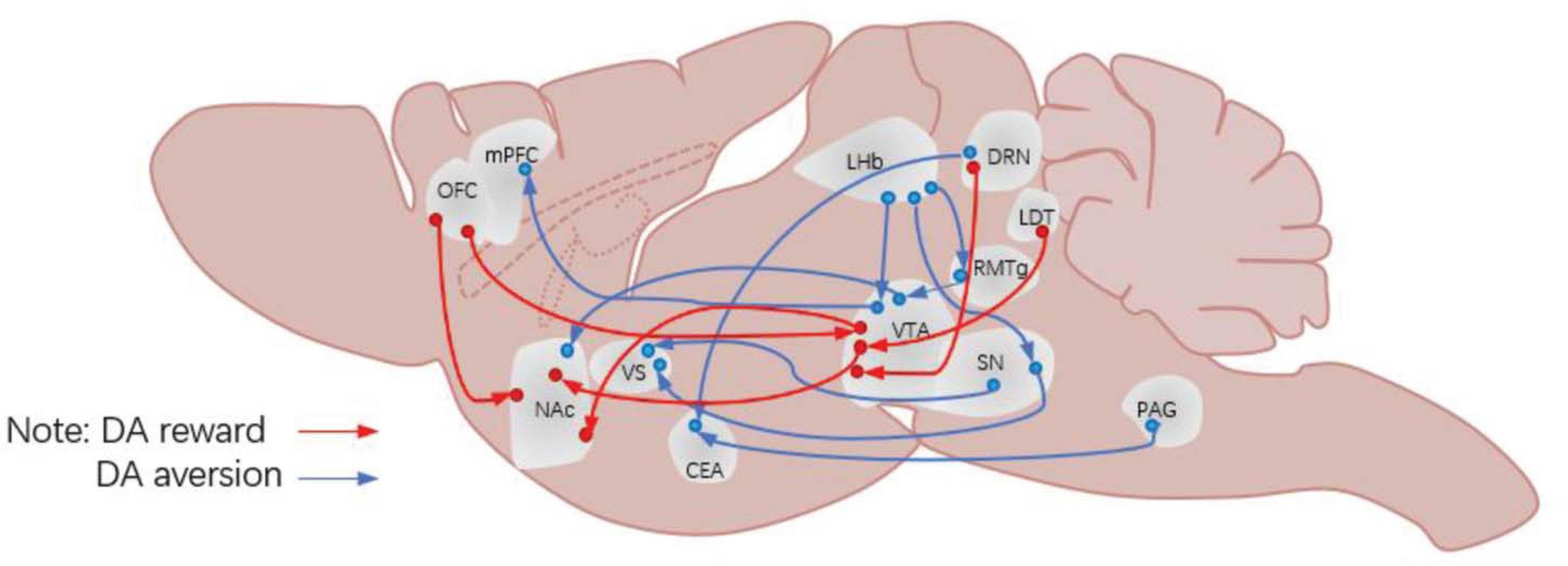
Figure 1. Neural circuits for DA projection. DA, dopamine; OFC, orbitofrontal cortex; mPFC, medial prefrontal cortex; NAc, nucleus accumbens; LHb, lateral habenula; VS, ventral striatum; CEA, central amygdala; VTA, ventral tegmental area; SN, substantia nigra pars compacta; RMTg, rostromedial tegmental nucleus; DRN, dorsal raphe nucleus; PAG, periaqueductal gray matter; LDT, laterodorsal tegmental nucleus.
The Aversive Function of Dopaminergic Neurons in the Substantia Nigra
Like the VTA, the SN is also an important nucleus of DAergic neurons. Some recent studies also found some aversive pathways related to SN DAergic neurons. Matsumoto and Hikosaka (2009) conducted a study about Pavlov’s classical conditioned reflex and found that the LHb → SN DA → dorsal striatum (dVS) pathway is likely responsible for processing aversive stimulus. And the same result was also found in the SN DA → ventral striatum (VS) pathway. Menegas et al. (2017, 2018) found that the SN DA → VS pathway played an important role in the fear aversion learning task of rats. The discovery of the pathway is consistent with their previous findings that lateral SN DAergic neurons are involved in the processing of aversive stimuli.
Aversive Function of Periaqueductal Gray Matter and Dorsal Raphe Nucleus Dopaminergic Neurons
Unlike the VTA and SN, the presence of DAergic neurons in the PAG and DRN has only been discovered in recent studies (Cardozo Pinto et al., 2019). Therefore, there is still some controversy about whether there are DAergic neurons in these two brain regions. With the advent of electrophysiology and other biological imaging techniques, some researchers have begun to study the characteristics of DAergic neurons in these two regions (Dougalis et al., 2012; Li et al., 2016) and have made a series of reports, which further support the existence of DAergic neurons in these two brain regions. Similarly, some studies have reported about the aversive function of PAG and DRN DAergic neurons, which have shown that the two brain regions (the PAG and DRN) play an important role in coping with pain stimuli (Eippert and Tracey, 2014; Gadotti et al., 2019), and the amygdala is an important targeting area of these two brain regions (McNally and Cole, 2006). Therefore, it is important to explore whether the PAG/DRN → amygdala circuit is involved in the processing of aversion stimuli. The study conducted by Groessl et al. (2018) found that PAG and DRN DAergic neurons play an important role in the processing of aversive stimulus and aversive prediction error processing (Charney, 2004). At the same time, it is found that PAG and DRN DAergic neurons projected to the central amygdala (CE) and confirmed that the PAG/DRN → CE circle is responsible for the aversive stimulus and the processing of aversive clues.
In addition to the above regions, DAergic neurons in other areas in the limbic system have also been found to be engaged in the processing of aversive stimuli. A recent study of Kramar et al. (2021) showed that the VTA DA release promotes the activation of DAergic neurons in the dorsal hippocampus, which reinforces late consolidation of aversive memory (Clewett and Murty, 2019; Shao et al., 2021). The hypothalamus is an important area of emotional processing in the limbic system with its medial and lateral nuclei processing different information. The medial nucleus is mainly responsible for the processing of reward stimuli such as food intake and sexual behavior, while the lateral nuclei are responsible for the processing of aversive information including stress and tension (Barbosa et al., 2017). It is worth noting that a recent study found that LHb projection to LHA neurons is responsible for encoding aversive information (Lazaridis et al., 2019). As Lammel et al. (2012) previously reported that aversive information is processed by DA transmission of (LHb) → VTA DA → mPFC circle, is the LHb → LHA projection related to DAergic neurons? Therefore, further research should focus not only on traditional distribution of DAergic neurons mentioned above but also on whether other areas of the limbic system and DAergic neurons in nuclei are involved in the processing of aversive information.
Dopamine and Norepinephrine
Dopamine and norepinephrine (NE) neurons project widely in the whole brain (Charney, 2004). NE is mainly produced by neurons in the locus coeruleus (LC), and the LC–NE systems respond to stress by globally priming neurons in the brain (Nestler and Aghajanian, 1997; McCall et al., 2015). The already known functions of NE are alerting (Mandalaywala et al., 2017), arousal (Carter et al., 2010), recognition (Wagatsuma et al., 2018), sleep/awake transitions (Carter et al., 2012), and drug addiction (Cao et al., 2010). Some recent studies have also found that the LC–NE is related to stress recovery (Valentino and Van Bockstaele, 2015; Liang et al., 2021; Sullivan and Ballantyne, 2021) as the function of the pursuit of reward and the avoidance of punishment in DA and the function of alerting and arousal in norepinephrine are respectively relative to the valence and arousal in the theory of emotional dimension. Wang F. et al. (2020) added serotonin (5-HT) as another neurotransmitter and put up “three primary color model” of basic emotions (Gu et al., 2019b). The theory proposed that all emotions are composed of some basic emotions, such as happiness, sadness, and anger and fear, which are subsided respectively by the three neurotransmitters: DA, happiness; 5-HT, sadness; and NE, fear (anger). Recent studies have indicated that the VTA is a downstream projection area of the LC (Isingrini et al., 2016), and the LC–NE neurons manage negative emotion through suppressing VTA DAergic neurons. Current studies on the effect of NE transmitter to DAergic neurons focusing on addictive behavior (Grace et al., 2007) and the treatment of depression (Friedman et al., 2016; Gu et al., 2020) suggested that the LC–NE neurons projection to midbrain DA neurons and that their target structures play a vital role in the changes of neural circuits. These changes might be the causes for pathological behaviors, including the development and maintenance of addiction and the alteration of the reward and aversion neural circuits in patients with addiction or depression (Everitt and Robbins, 2005; Hyman et al., 2006; Schultz, 2007; Admon et al., 2017). It is crucially important to explore the mechanisms of changes of DAergic neurons, which are modulated by NE neurotransmitters. Relevant studies have shown that DAergic neurons are tensely distributed with α1 and β3 adrenergic receptors (Zhang et al., 2019). In the process of reward, the VTA DAergic neurons mainly receive the projection of glutamic acid (Mingote et al., 2019; Wang J. et al., 2020), GABA (Bouarab et al., 2019), and NE neurons. And the main function of DAergic neurons is to suppress the activation of GABAergic neuron. On the one hand, α1-Ars receptors can promote release of DA from DAergic neurons (Mitrano et al., 2012). In addition, α1-Ars receptors can also affect the release of GABA directly in the VTA DAergic neurons (Velasquez-Martinez et al., 2015) and further strengthen the activation of DAergic neurons (Figure 2). Velasquez-Martinez et al. (2015) found that cocaine-induced inhibition of monoamine reuptake may further enhance the availability of NE in synapse and invoke stronger stimulation of α1-ARs but may reduce the release of GABA in the VTA neurons (Martins et al., 2019). It may reverse neurotransmitter actions related to aversive behaviors, increase the nervous excitability of DAergic neurons, and then cause sensitization to drugs like cocaine (Clewett and Murty, 2019; Velasquez-Martinez et al., 2020). Zhang et al. (2019) reported in a study of social pressure and sensitivity that mice with stronger psychological resilience will release more NE than those with weaker psychological resilience in the circumstance of depression. After depressive-like behaviors were reversed, NE release increased rapidly, which indicates that the LC–NE system has an important function in mediating the psychological resilience of humans and animals.
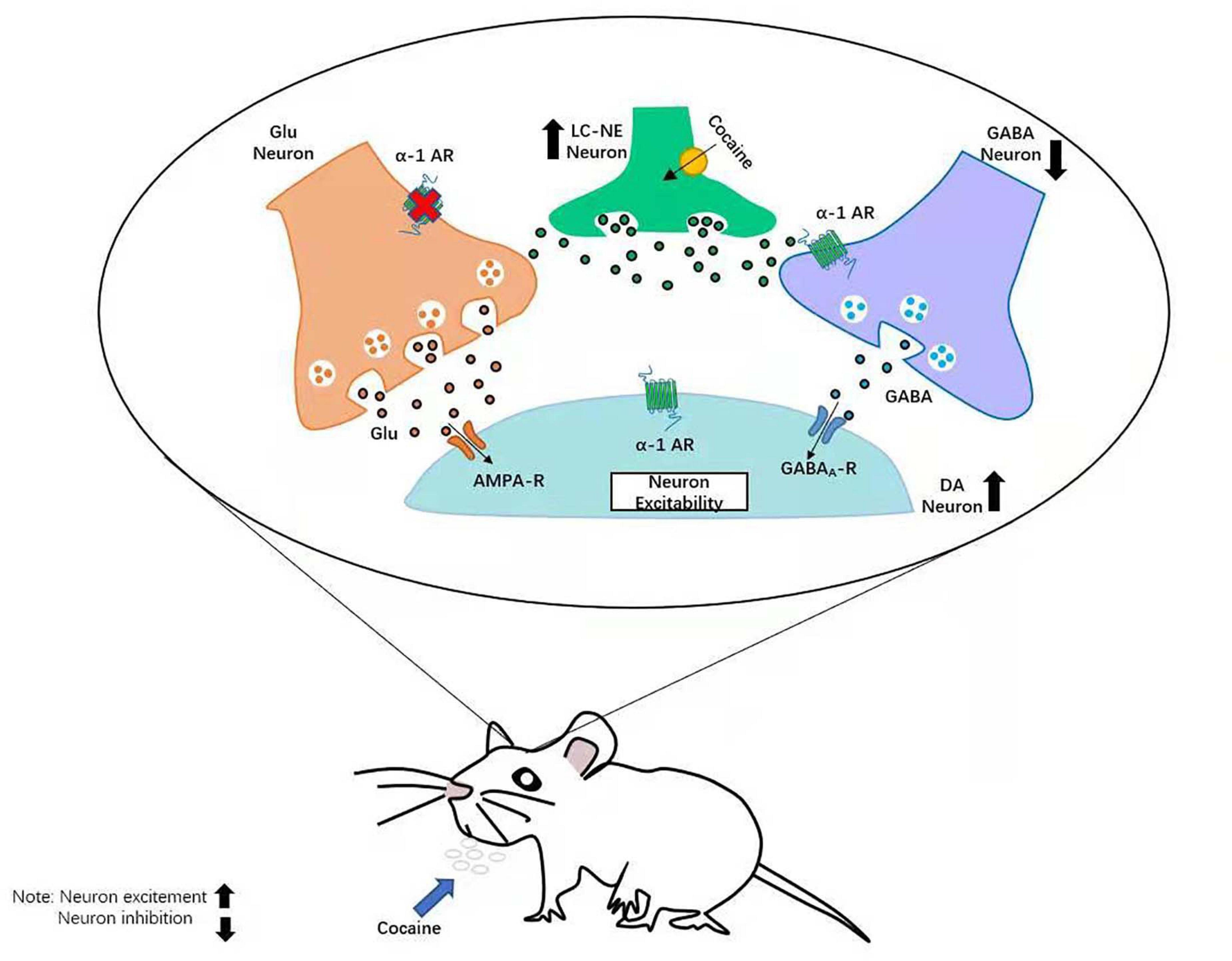
Figure 2. Neural mechanism of LC–NE affecting DA in addictive behavior. LC, locus coeruleus; NE, norepinephrine; DA, dopamine.
In summary, NE (especially α1-Ars) participates in the processing of reward information of the VTA DAergic neurons, which directly promote the activation of DAergic neurons and hinder the release of antagonist GABA of DA. It will further affect the processing of reward information to the VTA DA and is significantly meaningful to the relevant treatment of addictive behavior, depression, and some diseases. Meanwhile, other studies (Mitrano et al., 2012) have found that the function of DA system and NE system interacts in other brain regions, which affect many psychological processes.
Dopamine and Serotonin
5-HT was first suggested as a neurotransmitter for aversion in Bradley et al. (1986), and there is a great overlap in function of both 5-HT and DA (Yagishita, 2020). But 5-HT plays a vital role in motivation-related functions, such as reward and aversion learning, incentive processing, decision making, and goal-oriented behavior (Hu, 2016). The main gathering area for 5-HT neurons is the DRNs. The 5-HT neurons of the DRN send projection to the entire brain, including the striatum and thalamus (including the posterior complex and the lateral geniculate nucleus, the anterior ventral nucleus, and the anterior ventral nucleus), cingulate cortex, PFC (including medial PFC), temporal lobe, and sensory cortex (Conio et al., 2020), and receive the input from the hypothalamus, amygdala, midbrain, and the anterior neocortex (Weissbourd et al., 2014). The types of 5-HT receptors are more complicated than those of DA. There are 14 types of 5-HT receptors and can be divided into seven main families according to different G-proteins coupled. Different receptors in different families have different functions. For example, 5-HT1 plays a role in rewards (Akizawa et al., 2019) and anxiolytics (Ramboz et al., 1998), 5-HT2A receptors are correlated to hallucinations and insights (Vollenweider et al., 1998); 5-HT4 receptors have an effect on memory, depression, and feeding (Bockaert et al., 2008); and 5-HT7 is related to cognitive processes, such as learning and memory (Zareifopoulos and Papatheodoropoulos, 2016).
Although the psychiatric DA hypothesis has been the main hypothesis in the field of psychiatry in the past 50s, almost all drugs for the treatment of psychosis involve DA receptors, especially D2Rs (Stahl, 2018). However, more and more studies have found that 5-HT is also vital to treating mental disorders. Some symptoms of mental patients can also be alleviated by drugs interfered with 5-HT or 5-HT receptors in related brain regions (Stahl, 2016). Therefore, some scholars put forward that psychosis is a condition involving the disorder of multiple neurotransmitters in multiple pathways, but DA and 5-HT may be functioned in some psychosis processes at the same time (Rolland et al., 2014). New therapies for pathways and receptors besides D2Rs in the mesolimbic pathway have received more and more attention. The classic psychiatric DA hypothesis indicates that the abnormal DA pathway in the middle-marginal DA pathway is the cause of most psychosis, and almost all antipsychotic drugs block D2Rs there (Stahl, 2018).
However, a large number of clinical researches have indicated that blocking the D2Rs in this pathway will simultaneously block the D2Rs in the pathway of the SN to the dorsal striatum, leading to some motor disorders, such as meditation, drug-induced PD, and long-term TD (Stahl, 2016). Meanwhile, although the method of blocking D2Rs is effective for patients with manic, depressive psychosis and schizophrenia, it is not ideal for treating PD, Alzheimer’s, and other types of psychosis and even makes the symptoms worse (Ravina et al., 2007; Fénelon et al., 2010). Therefore, researchers have started to study other neurotransmitters in the treatment for psychiatric patients. The 5-HT theory is proposed in this context, which believes that psychosis may be the result of excessive activation of 5-HT2A receptors in glutamate neurons. Thus, blocking the overdose neurotransmission of 5-HT in patients with 5-HT2A receptors in psychosis can theoretically restore the balance between 5-HT and DA and can reduce visual hallucinations and delusions instead of worsening motor symptoms. It can effectively treat PD and Alzheimer’s (Stahl, 2018). Current studies have shown that the loss of DA in the dorsal striatum due to the SNc in patients of PD will change the normal balance between 5-HT and DA. Meanwhile, the 5-HT neurons in the fissure will degenerate with the original loss of 5-HT and worsen with the PD. Pyramidal neurons in 5-HT-related brain regions degenerate, and the number of 5-HT2A receptors is increased in the remaining neurons in the cerebral cortex, which leads to hallucinations in patients (Ballanger et al., 2010; Huot et al., 2010). In other words, 5-HT abnormalities are not significantly related to motor symptoms in PD but may be related to non-motor symptoms. Related clinical studies have found that 5-HT2A antagonists without d2 antagonists have been shown to be effective in the treatment of psychotic symptoms caused by PD. Among the second-generation antipsychotic drugs, 5-HT2A receptors are also important targets for drug theory. Simultaneous targeted therapy of DA D2Rs and 5-HT 5-HT2A receptors shows better performance than a single intervention on D2Rs and fewer side effects (Xia et al., 2019). To sum up, FND patients show obvious physical symptoms and mood disorders. 5-HT is related to many affective and executive functions. Related researches have indicated that 5-HT is associated with anxiety (Deakin and Graeff, 1991), depression (Kraus et al., 2017), alexithymia (Li et al., 2020), and emotion regulation disorder (Stiedl et al., 2009). Meanwhile, the function of 5-HT and FND patients in rewarding and aversion processing has received more and more attention. The aversive function of 5-HT has been early discovered (Bocchio et al., 2016), and behavior inhabitation (Crockett et al., 2009) has also been proved to be a vital function of 5-HT. And it is found that the NAc, meanwhile, receives the projection of 5-HT, and these neurons projected by 5-HT are also activated when receiving the projection of rewarding stimuli, which shows that it is closely related to rewarding (Barot et al., 2007). And DRN 5-HT neurons are excited about the actual reward (Liu et al., 2014) regardless of whether the reward is predicted or not. Therefore, considering that 5-HT is involved in the two major obstacles of FND at the same time, drug intervention in the 5-HT concentration of FND patients or drug treatment with 5-HT receptors as a target may alleviate some symptoms of FND patients to a certain extent. In the future, it is necessary to further clarify the role of DA and 5-HT in the common projection area (such as the NAc) domain, which is meaningful to intervene related transmitter receptors precisely and cue some related mental diseases.
Shortcomings and Prospects
The aversive function of DAergic neurons in the limbic system has been confirmed by many experiments, and the results have gradually obtained unanimous agreement. Many regions in the limbic system such as the VTA, SN, PAG, DRN, and VS (NAc) are suggested to be involved in processing aversive stimuli, and more relevant studies are gathering momentum. However, there are still some concerns in this area of research.
First, the technology of DA measurement is of concern. Problems still exist despite that significant progress has been done in the DA measurement with improved technology of electrophysiology, imaging, and virus tracking; DA imaging is still impossible to be applied to the human brain due to its invasive nature (Verharen et al., 2020). It can be speculated that DA in the human brain is engaged in the processing of aversive stimuli according to research on mice and rhesus monkeys. Humans, however, as higher organisms, show significant difference on brain structure and function with mice and monkeys. Further studies are needed on whether DAergic neurons in the limbic system of the human brain operate and function the same as the brains of mice and monkeys.
Second, whether DAergic neurons can be identified accurately emerges as a more important question. Morales and Margolis (2017) raised doubts about the identification of DAergic neurons in the VTA in the experiments. For example, neurons in mice can produce mRNA for tyrosine hydroxylase (TH) but cannot synthesize the enzyme required for DA. Therefore, false positives may be caused by identifying or manipulating DA neurons in the experiment on TH mRNA of mice (Yamaguchi et al., 2015).
Another concern relates to the ambiguous definition of aversion. Biologically, an aversive feeling is defined as an inner feeling when a creature avoids potential harm of stimuli (toxins, predators, and mechanical stress) on body for survival (Tovote et al., 2016). This definition, however, is too broad, as it includes almost all negative emotions and it leads to many problems in specific experiments. Stimuli in psychology and biology such as fear, pain, and social isolation are all considered as aversive stimuli; their value and degree of arousal, however, lack clear distinction (Lammel et al., 2012; Li et al., 2016; Matthews et al., 2016). So which stimulus incurs the reaction of DAergic neurons? Is it fear, pain, or the psychological effects of social isolation? Failure to distinguish value (aversion) and arousal (alertness) will lead to the confusion between aversion and alertness. Which feature of the stimulus produces a high level release of DA (Bromberg-Martin et al., 2010; Gu et al., 2019a)? Therefore, more studies are needed in studying how to further distinguish biological aversive stimuli, especially the distinction between value and arousal.
Conclusion
To sum up, the article starts with the aversion function of DA, discussing the definition of aversion. Then according to series of studies, it discusses some special subtypes of DAergic neurons that exist in multiple brain areas of the limbic system. Though some researchers hold opposite views on whether DAergic neurons are involved in the processing of aversive stimuli, they show excitation when giving aversive stimuli and cues. Then, the article discusses the prediction error function of DA. According to some performances of FND patients in event prediction, it is speculated that the abnormal prediction error loop of DA may be the potential cause of FND. Next, the article summarizes the neural circuits that DA neurons in the limbic system participate in the processing of aversive stimuli and propose some assumptions about neural circuits of DA projection. After that, it discusses the relationship of NE and DA based on the researches of addictive behavior and the treatment of depression. Finally, shortcomings of current researches and prospects of future study are summarized, attempting to help further research on DA.
Author Contributions
ZH, YJ, DQ, and FW conceived the study. ZH, YJ, SG, FW, JH, GF, and XM wrote and revised the manuscript. All the authors contributed to the article and approved the submitted version.
Funding
This study was supported by a grant from Foundation of Humanities and Arts from the Ministry of Education in China (19YJAZH083).
Conflict of Interest
The authors declare that the research was conducted in the absence of any commercial or financial relationships that could be construed as a potential conflict of interest.
Publisher’s Note
All claims expressed in this article are solely those of the authors and do not necessarily represent those of their affiliated organizations, or those of the publisher, the editors and the reviewers. Any product that may be evaluated in this article, or claim that may be made by its manufacturer, is not guaranteed or endorsed by the publisher.
References
Abercrombie, E. D., Keefe, K. A., DiFrischia, D. S., and Zigmond, M. J. (1989). Differential effect of stress on in vivo da release in striatum, nucleus accumbens, and medial frontal cortex. J. Neurochem. 52, 1655–1658.
Adcock, R. A., Thangavel, A., Whitfield-Gabrieli, S., Knutson, B., and Gabrieli, J. D. E. (2006). Reward-Motivated learning: mesolimbic activation precedes memory formation. Neuron 50, 507–517. doi: 10.1016/J.NEURON.2006.03.036
Admon, R., Kaiser, R. H., Dillon, D. G., Beltzer, M., Goer, F., Olson, D. P., et al. (2017). DArgic enhancement of striatal response to reward in major depression. Am. J. Psychiatry 174, 378–386. doi: 10.1176/appi.ajp.2016.16010111
Akizawa, F., Mizuhiki, T., Setogawa, T., Takafuji, M., and Shidara, M. (2019). The effect of 5-HT1A receptor antagonist on reward-based decision-making. J. Physiol. Sci. 69, 1057–1069. doi: 10.1007/s12576-019-00725-1
Al-Hasani, R., McCall, J. G., Shin, G., Gomez, A. M., Schmitz, G. P., Bernardi, J. M., et al. (2015). Distinct subpopulations of nucleus accumbens dynorphin neurons drive aversion and reward. Neuron 87, 1063–1077. doi: 10.1016/J.NEURON.2015.08.019
Aybek, S., Nicholson, T. R., O’Daly, O., Zelaya, F., Kanaan, R. A., and David, A. S. (2015). Emotion-motion interactions in conversion disorder: an FMRI study. PLoS One 10:e0123273. doi: 10.1371/journal.pone.0123273
Badrinarayan, A., Wescott, S. A., Vander Weele, C. M., Saunders, B. T., Couturier, B. E., Maren, S., et al. (2012). Aversive stimuli differentially modulate real-time DA transmission dynamics within the nucleus accumbens core and shell. J. Neurosci. 32, 15779–15790. doi: 10.1523/JNEUROSCI.3557-12.2012
Ballanger, B., Strafella, A. P., Eimeren, T. V., Zurowski, M., Rusjan, P. M., Houle, S., et al. (2010). 5-HT 2A receptors and visual hallucinations in parkinson disease. JAMA Neurol. 67, 416–421. doi: 10.1001/ARCHNEUROL.2010.35
Barbosa, D. A. N., de Oliveira-Souza, R., Monte Santo, F., de Oliveira Faria, A. C., Gorgulho, A. A., and De Salles, A. A. F. (2017). The hypothalamus at the crossroads of psychopathology and neurosurgery. Neurosurg. Focus 43:E15. doi: 10.3171/2017.6.FOCUS17256
Barot, S. K., Ferguson, S. M., and Neumaier, J. F. (2007). 5-HT(1B) receptors in nucleus accumbens efferents enhance both rewarding and aversive effects of cocaine. Eur. J. Neurosci. 25, 3125–3131. doi: 10.1111/j.1460-9568.2007.05568.x
Barr, G. A., Moriceau, S., Shionoya, K., Muzny, K., Gao, P., Wang, S., et al. (2009). Transitions in infant learning are modulated by DA in the amygdala. Nat. Neurosci. 12, 1367–1369. doi: 10.1038/nn.2403
Beaulieu, J. M., and Gainetdinov, R. R. (2011). The physiology, signaling, and pharmacology of DA receptors. Pharmacol. Rev. 63, 182–217. doi: 10.1124/pr.110.002642
Beaulieu, J. M., Tirotta, E., Sotnikova, T. D., Masri, B., Salahpour, A., Gainetdinov, R. R., et al. (2007). Regulation of akt signaling by D2 and D3 DA receptors in vivo. J. Neurosci. 27, 881–885. doi: 10.1523/JNEUROSCI.5074-06.2007
Berridge, K. C. (2000). Measuring hedonic impact in animals and infants: microstructure of affective taste reactivity patterns. Neurosci. Biobehav. Rev. 24, 173–198. doi: 10.1016/S0149-7634(99)00072-X
Berridge, K. C. (2018). Evolving concepts of emotion and motivation. Front. Psychol. 9:1647. doi: 10.3389/fpsyg.2018.01647
Berridge, K. C., and Kringelbach, M. L. (2015). Pleasure systems in the brain. Neuron 86, 646–664. doi: 10.1016/j.neuron.2015.02.018
Bjorklund, A., and Dunnett, S. B. (2007). DA neuron systems in the brain: an update. Trends Neurosci. 30, 194–202. doi: 10.1016/j.tins.2007.03.006
Bocchio, M., McHugh, S. B., Bannerman, D. M., Sharp, T., and Capogna, M. (2016). 5-HT, amygdala and fear: assembling the puzzle. Front. Neural Circuits 10:24. doi: 10.3389/fncir.2016.00024
Bockaert, J., Claeysen, S., Compan, V., and Dumuis, A. (2008). 5-HT(4) receptors: history, molecular pharmacology and brain functions. Neuropharmacology 55, 922–931. doi: 10.1016/j.neuropharm.2008.05.013
Bonuccelli, U., Dotto, P. D., and Rascol, O. (2009). Role of DA receptor agonists in the treatment of early PD. Parkinsonism Related Dis. 15, (Suppl. 4), S44–S53. doi: 10.1016/S1353-8020(09)70835-1
Bouarab, C., Thompson, B., and Polter, A. M. (2019). VTA gaba neurons at the interface of stress and reward. Front. Neural Circuits 13:78. doi: 10.3389/fncir.2019.00078
Bradberry, C. W., Lory, J. D., and Roth, R. H. (1991). The anxiogenic β-Carboline FG 7142 selectively increases DA release in rat prefrontal cortex as measured by microdialysis. J. Neurochem. 56, 748–752. doi: 10.1111/J.1471-4159.1991.TB01987.X
Bradley, P. B., Engel, G., Feniuk, W., Fozard, J. R., Humphrey, P. P., Middlemiss, D. N., et al. (1986). Proposals for the classification and nomenclature of functional receptors for 5-hydroxytryptamine. Neuropharmacology 25, 563–576. doi: 10.1016/0028-3908(86)90207-8
Brischoux, F., Chakraborty, S., Brierley, D. I., and Ungless, M. A. (2009). Phasic excitation of DA neurons in ventral VTA by noxious stimuli. Proc. Natl. Acad. Sci. U S A. 106, 4894–4899. doi: 10.1073/PNAS.0811507106
Brodsky, E. E., and Lajoie, L. J. (2013). Anthropogenic seismicity rates and operational parameters at the salton sea geothermal field. Science 341, 543–546. doi: 10.1126/SCIENCE.1239213
Bromberg-Martin, E. S., Matsumoto, M., and Hikosaka, O. (2010). DA in motivational control: rewarding, aversive, and alerting. Neuron 68, 815–834. doi: 10.1016/j.neuron.2010.11.022
Brown, R. J., and Reuber, M. (2016). Psychological and psychiatric aspects of psychogenic non-epileptic seizures (PNES): a systematic review. Clin. Psychol. Rev. 45, 157–182. doi: 10.1016/J.CPR.2016.01.003
Cao, J. L., Vialou, V. F., Lobo, M. K., Robison, A. J., Neve, R. L., Cooper, D. C., et al. (2010). Essential role of the cAMP-cAMP response-element binding protein pathway in opiate-induced homeostatic adaptations of locus coeruleus neurons. Proc. Natl. Acad. Sci. U S A. 107, 17011–17016. doi: 10.1073/pnas.1010077107
Cardozo Pinto, D. F., Yang, H., Pollak Dorocic, I., de Jong, J. W., Han, V. J., Peck, J. R., et al. (2019). Characterization of transgenic mouse models targeting neuromodulatory systems reveals organizational principles of the dorsal raphe. Nat. Commun. 10:4633. doi: 10.1038/s41467-019-12392-2
Carson, A., Best, S., Postma, K., Stone, J., Warlow, C., and Sharpe, M. (2003). The outcome of neurology outpatients with medically unexplained symptoms: a prospective cohort study. J. Neurol. Neurosurg. Psychiatry 74, 897–900. doi: 10.1136/JNNP.74.7.897
Carson, A., and Lehn, A. (2016). Epidemiology. Handb. Clin. Neurol. 139, 47–60. doi: 10.1016/B978-0-12-801772-2.00005-9
Carter, M. E., Brill, J., Bonnavion, P., Huguenard, J. R., Huerta, R., and de Lecea, L. (2012). Mechanism for hypocretin-mediated sleep-to-wake transitions. Proc. Natl. Acad. Sci. U S A. 109, E2635–E2644. doi: 10.1073/pnas.1202526109
Carter, M. E., Yizhar, O., Chikahisa, S., Nguyen, H., Adamantidis, A., Nishino, S., et al. (2010). Tuning arousal with optogenetic modulation of locus coeruleus neurons. Nat. Neurosci. 13, 1526–1533. doi: 10.1038/nn.2682
Cerniauskas, I., Winterer, J., de Jong, J. W., Lukacsovich, D., Yang, H., Khan, F., et al. (2019). Chronic stress induces activity, synaptic, and transcriptional remodeling of the lateral habenula associated with deficits in motivated behaviors. Neuron 104, 899–915.e8. doi: 10.1016/J.NEURON.2019.09.005
Chapman, H. A., Kim, D. A., Susskind, J. M., and Anderson, A. K. (2009). In bad taste: evidence for the oral origins of moral disgust. Science 323, 1222–1226. doi: 10.1126/SCIENCE.1165565
Charney, D. S. (2004). Psychobiological mechanisms of resilience and vulnerability: implications for successful adaptation to extreme stress. Am. J. Psychiatry 161, 195–216. doi: 10.1176/APPI.AJP.161.2.195
Clewett, D., and Murty, V. P. (2019). Echoes of emotions past: how neuromodulators determine what we recollect. eNeuro 6:ENEURO.0108-18.2019. doi: 10.1523/ENEURO.0108-18.2019
Cohen, J. Y., Haesler, S., Vong, L., Lowell, B. B., and Uchida, N. (2012). Neuron-type-specific signals for reward and punishment in the ventral tegmental area. Nature 482, 85–88. doi: 10.1038/nature10754
Conio, B., Martino, M., Magioncalda, P., Escelsior, A., Inglese, M., Amore, M., et al. (2020). Opposite effects of DA and 5-HT on resting-state networks: review and implications for psychiatric disorders. Mol. Psychiatry 25, 82–93. doi: 10.1038/s41380-019-0406-4
Corbett, D., and Wise, R. A. (1980). Intracranial self-stimulation in relation to the ascending DArgic systems of the midbrain: a moveable electrode mapping study. Brain Res. 185, 1–15. doi: 10.1016/0006-8993(80)90666-6
Crockett, M. J., Clark, L., and Robbins, T. W. (2009). Reconciling the role of 5-HT in behavioral inhibition and aversion: acute tryptophan depletion abolishes punishment-induced inhibition in humans. J. Neurosci. 29, 11993–11999. doi: 10.1523/JNEUROSCI.2513-09.2009
Dalley, J. W., Lääne, K., Theobald, D. E. H., Armstrong, H. C., Corlett, P. R., Chudasama, Y., et al. (2005). Time-limited modulation of appetitive Pavlovian memory by D1 and NMDA receptors in the nucleus accumbens. Proc. Natl. Acad. Sci. U S A. 102, 6189–6194. doi: 10.1073/PNAS.0502080102
Davidson, M. C., Horvitz, J. C., Tottenham, N., Fossella, J. A., Watts, R., Ulug, A. M., et al. (2004). Differential caudate and cingulate activation following unexpected nonrewarding stimuli. Neuroimage 23, 1039–1045. doi: 10.1016/j.neuroimage.2004.07.049
de Jong, J. W., Afjei, S. A., Pollak Dorocic, I., Peck, J. R., Liu, C., Kim, C. K., et al. (2019). A neural circuit mechanism for encoding aversive stimuli in the mesolimbic DA system. Neuron 101, 133–151.e7. doi: 10.1016/j.neuron.2018.11.005
Deakin, J. F. W., and Graeff, F. G. (1991). 5-HT and mechanisms of defence. J. Psychopharmacol. 5, 305–315. doi: 10.1177/026988119100500414
Dougalis, A. G., Matthews, G. A. C., Bishop, M. W., Brischoux, F., Kobayashi, K., and Ungless, M. A. (2012). Functional properties of DA neurons and co-expression of vasoactive intestinal polypeptide in the dorsal raphe nucleus and ventro-lateral periaqueductal grey. Eur. J. Neurosci. 36, 3322–3332. doi: 10.1111/J.1460-9568.2012.08255.X
Eippert, F., and Tracey, I. (2014). Pain and the PAG: learning from painful mistakes. Nat. Neurosci. 17, 1438–1439. doi: 10.1038/nn.3844
Ellwood, I. T., Patel, T., Wadia, V., Lee, A. T., Liptak, A. T., Bender, K. J., et al. (2017). Tonic or phasic stimulation of DArgic projections to prefrontal cortex causes mice to maintain or deviate from previously learned behavioral strategies. J. Neurosci. 37, 8315–8329. doi: 10.1523/JNEUROSCI.1221-17.2017
Espay, A. J., Maloney, T., Vannest, J., Norris, M. M., Eliassen, J. C., Neefus, E., et al. (2018). Impaired emotion processing in functional (psychogenic) tremor: a functional magnetic resonance imaging study. Neuroimage Clin. 17, 179–187. doi: 10.1016/j.nicl.2017.10.020
Everitt, B. J., and Robbins, T. W. (2005). Neural systems of reinforcement for drug addiction: from actions to habits to compulsion. Nat. Neurosci. 8, 1481–1489. doi: 10.1038/nn1579
Fadok, J. P., Dickerson, T. M. K., and Palmiter, R. D. (2009). DA is necessary for cue-dependent fear conditioning. J. Neurosci. 29, 11089–11097. doi: 10.1523/JNEUROSCI.1616-09.2009
Fagan, R. R., Kearney, P. J., and Melikian, H. E. (2020). In situ regulated DA transporter trafficking: there’s no place like home. Neurochem. Res. 45, 1335–1343. doi: 10.1007/s11064-020-03001-6
Fan, X., Xu, M., and Hess, E. J. (2010). D2 DA receptor subtype-mediated hyperactivity and amphetamine responses in a model of ADHD. Neurobiol. Dis. 37, 228–236. doi: 10.1016/j.nbd.2009.10.009
Fawcett, J., Rush, A. J., Vukelich, J., Diaz, S. H., Dunklee, L., Romo, P., et al. (2016). Clinical experience with high-dosage pramipexole in patients with treatment-resistant depressive episodes in unipolar and bipolar depression. Am. J. Psychiatry 173, 107–111. doi: 10.1176/APPI.AJP.2015.15060788
Fénelon, G., Soulas, T., Zenasni, F., and Langavant, L. C. D. (2010). The changing face of PD-associated psychosis: a cross-sectional study based on the new NINDS-NIMH criteria. Mov. Disord. 25, 763–766. doi: 10.1002/MDS.22839
Fiorillo, C. D., Yun, S. R., and Song, M. R. (2013). Diversity and homogeneity in responses of midbrain DA neurons. J. Neurosci. 33, 4693–4709. doi: 10.1523/JNEUROSCI.3886-12.2013
Fouriezos, G., and Wise, R. A. (1976). Pimozide-induced extinction of intracranial self-stimulation: response patterns rule out motor or performance deficits. Brain Res. 103, 377–380. doi: 10.1016/0006-8993(76)90809-X
Friedman, A. K., Juarez, B., Ku, S. M., Zhang, H., Calizo, R. C., Walsh, J. J., et al. (2016). KCNQ channel openers reverse depressive symptoms via an active resilience mechanism. Nat. Commun. 7:11671. doi: 10.1038/ncomms11671
Friedman, A. K., Walsh, J. J., Juarez, B., Ku, S. M., Chaudhury, D., Wang, J., et al. (2014). Enhancing depression mechanisms in midbrain DA neurons achieves homeostatic resilience. Science 344, 313–319. doi: 10.1126/SCIENCE.1249240
Gadotti, V. M., Zhang, Z., Huang, J., and Zamponi, G. W. (2019). Analgesic effects of optogenetic inhibition of basolateral amygdala inputs into the prefrontal cortex in nerve injured female mice. Mol. Brain 12:105. doi: 10.1186/s13041-019-0529-1
Gerfen, C. R. (2000). Molecular effects of DA on striatal-projection pathways. Trends Neurosci. 23, S64–S70. doi: 10.1016/S1471-1931(00)00019-7
Gerfen, C. R., and Surmeier, D. J. (2011). Modulation of striatal projection systems by DA. Annu. Rev. Neurosci. 34, 441–466. doi: 10.1146/annurev-neuro-061010-113641
Grace, A. A., Floresco, S. B., Goto, Y., and Lodge, D. J. (2007). Regulation of firing of DArgic neurons and control of goal-directed behaviors. Trends Neurosci. 30, 220–227. doi: 10.1016/j.tins.2007.03.003
Groessl, F., Munsch, T., Meis, S., Griessner, J., Kaczanowska, J., Pliota, P., et al. (2018). Dorsal tegmental DA neurons gate associative learning of fear. Nat. Neurosci. 21, 952–962. doi: 10.1038/s41593-018-0174-5
Gu, S., Li, Y., Liang, F., Feng, R., Zeng, Z., and Wang, F. (2020). The mediating effects of coping style on the effects of breath count mindfulness training on depressive symptoms among international students in China. Neural Plast. 2020:8859251. doi: 10.1155/2020/8859251
Gu, S., Wang, F., Cao, C., Wu, E., Tang, Y. Y., and Huang, J. H. (2019a). An integrative way for studying neural basis of basic emotions with fMRI. Front. Neurosci. 13:628. doi: 10.3389/fnins.2019.00628
Gu, S., Wang, F., Patel, N. P., Bourgeois, J. A., and Huang, J. H. (2019b). A model for basic emotions using observations of behavior in Drosophila. Front. Psychol. 10:781. doi: 10.3389/fpsyg.2019.00781
Gu, S., Wang, F., Patel, N. P., Bourgeois, J. A., and Huang, J. H. (2015). Differentiation of primary emotions through neuromodulators: review of literature. Int. J. Neurol. Res. 1, 43–50. doi: 10.17554/j.issn.2313-5611.2015.01.19
Guo, F., Zhao, J., Zhao, D., Wang, J., Wang, X., Feng, Z., et al. (2017). DA D4 receptor activation restores CA1 LTP in hippocampal slices from aged mice. Aging Cell 16, 1323–1333. doi: 10.1111/ACEL.12666
He, L., Ni, D., and Qi, D. (2009). Neural Mechanisms of Aging Effect on Emotion Processing. 17, 356–361.
He, S., Wang, F., Yung, K. K. L., Zhang, S., and Qu, S. (2021). Effects of alpha-Synuclein-Associated post-translational modifications in PD. ACS Chem. Neurosci. 12, 1061–1071. doi: 10.1021/acschemneuro.1c00028
Hikida, T., Kimura, K., Wada, N., Funabiki, K., and Nakanishi, S. (2010). Distinct roles of synaptic transmission in direct and indirect striatal pathways to reward and aversive behavior. Neuron 66, 896–907. doi: 10.1016/J.NEURON.2010.05.011
Hu, H. (2016). Reward and aversion. Annu. Rev. Neurosci. 39, 297–324. doi: 10.1146/annurev-neuro-070815-014106
Huot, P., Johnston, T. H., Darr, T., Hazrati, L. N., Visanji, N. P., Pires, D., et al. (2010). Increased 5-HT2A receptors in the temporal cortex of parkinsonian patients with visual hallucinations. Mov. Disord. 25, 1399–1408. doi: 10.1002/MDS.23083
Hyman, S. E., Malenka, R. C., and Nestler, E. J. (2006). Neural mechanisms of addiction: the role of reward-related learning and memory. Annu. Rev. Neurosci. 29, 565–598. doi: 10.1146/annurev.neuro.29.051605.113009
Isingrini, E., Perret, L., Rainer, Q., Amilhon, B., Guma, E., Tanti, A., et al. (2016). Resilience to chronic stress is mediated by noradrenergic regulation of DA neurons. Nat. Neurosci. 19, 560–563. doi: 10.1038/nn.4245
Jankovic, J., McDermott, M. P., Carter, J. T., Gauthier, S. A., Goetz, C. G., Golbe, L. I., et al. (1990). Variable expression of PD A base-line analysis of the DAT ATOP cohort. Neurology 40, 1529–1529. doi: 10.1212/WNL.40.10.1529
Jean-Richard-Dit-Bressel, P., Killcross, S., and McNally, G. P. (2018). Behavioral and neurobiological mechanisms of punishment: implications for psychiatric disorders. Neuropsychopharmacology 43, 1639–1650. doi: 10.1038/s41386-018-0047-3
Joseph, M. H., Datla, K., and Young, A. M. J. (2003). The interpretation of the measurement of nucleus accumbens DA by in vivo dialysis: the kick, the craving or the cognition? Neurosci. Biobehav. Rev. 27, 527–541. doi: 10.1016/J.NEUBIOREV.2003.09.001
Kahneman, D., and Tversky, A. (1979). Prospect theory: an analysis of decision under risk. Econometrica 47, 263–291.
Kalia, L. V., and Lang, A. E. (2015). Parkinson’s disease. Lancet 386, 896–912. doi: 10.1016/s0140-6736(14)61393-3
Kim, C. K., Yang, S. J., Pichamoorthy, N., Young, N. P., Kauvar, I., Jennings, J. H., et al. (2016). Simultaneous fast measurement of circuit dynamics at multiple sites across the mammalian brain. Nat. Methods 13, 325–328. doi: 10.1038/nmeth.3770
Klein, M. O., Battagello, D. S., Cardoso, A. R., Hauser, D. N., Bittencourt, J. C., and Correa, R. G. (2019). DA: functions, signaling, and association with neurological diseases. Cell Mol. Neurobiol. 39, 31–59. doi: 10.1007/S10571-018-0632-3
Kramar, C. P., Castillo-Díaz, F., Gigante, E. D., Medina, J. H., and Barbano, M. F. (2021). The late consolidation of an aversive memory is promoted by VTA DA release in the dorsal hippocampus. Eur. J. Neurosci. 53, 841–851. doi: 10.1111/ejn.15076
Kraus, C., Castren, E., Kasper, S., and Lanzenberger, R. (2017). 5-HT and neuroplasticity - Links between molecular, functional and structural pathophysiology in depression. Neurosci. Biobehav. Rev. 77, 317–326. doi: 10.1016/j.neubiorev.2017.03.007
Kravitz, A. V., Freeze, B. S., Parker, P. R. L., Kay, K., Thwin, M. T., Deisseroth, K., et al. (2010). Regulation of parkinsonian motor behaviours by optogenetic control of basal ganglia circuitry. Nature 466, 622–626. doi: 10.1038/NATURE09159
Kravitz, A. V., Tye, L. D., and Kreitzer, A. C. (2012). Distinct roles for direct and indirect pathway striatal neurons in reinforcement. Nat. Neurosci. 15, 816–818. doi: 10.1038/NN.3100
Lammel, S., Ion, D. I., Roeper, J., and Malenka, R. C. (2011). Projection-specific modulation of DA neuron synapses by aversive and rewarding stimuli. Neuron 70, 855–862. doi: 10.1016/j.neuron.2011.03.025
Lammel, S., Lim, B. K., and Malenka, R. C. (2014). Reward and aversion in a heterogeneous midbrain DA system. Neuropharmacology 76(Pt B), 351–359. doi: 10.1016/j.neuropharm.2013.03.019
Lammel, S., Lim, B. K., Ran, C., Huang, K. W., Betley, M. J., Tye, K. M., et al. (2012). Input-specific control of reward and aversion in the ventral tegmental area. Nature 491, 212–217. doi: 10.1038/nature11527
Lammel, S., Steinberg, E. E., Földy, C., Wall, N. R., Beier, K., Luo, L., et al. (2015). Diversity of transgenic mouse models for selective targeting of midbrain DA neurons. Neuron 85, 429–438. doi: 10.1016/J.NEURON.2014.12.036
Lang, P. J., and Davis, M. (2006). Emotion, motivation, and the brain: reflex foundations in animal and human research. Prog. Brain Res. 156, 3–29. doi: 10.1016/S0079-6123(06)56001-7
Lazaridis, I., Tzortzi, O., Weglage, M., Martin, A., Xuan, Y., Parent, M., et al. (2019). A hypothalamus-habenula circuit controls aversion. Mol. Psychiatry 24, 1351–1368. doi: 10.1038/s41380-019-0369-5
Lehn, A., Gelauff, J., Hoeritzauer, I., Ludwig, L., McWhirter, L., Williams, S., et al. (2016). Functional neurological disorders: mechanisms and treatment. J. Neurol. 263, 611–620. doi: 10.1007/s00415-015-7893-2
Leriche, L., Bezard, E., Gross, C., Guillin, O., Foll, B. L., Diaz, J., et al. (2006). The DA D3 receptor: a therapeutic target for the treatment of neuropsychiatric disorders. CNS Neurol. Dis. Drug Targets 5, 25–43. doi: 10.2174/187152706784111551
Lerner, T. N., Shilyansky, C., Davidson, T. J., Evans, K. E., Beier, K. T., Zalocusky, K. A., et al. (2015). Intact-Brain analyses reveal distinct information carried by SNc DA subcircuits. Cell 162, 635–647. doi: 10.1016/j.cell.2015.07.014
Li, C., Sugam, J. A., Lowery-Gionta, E. G., McElligott, Z. A., McCall, N. M., Lopez, A. J., et al. (2016). Mu opioid receptor modulation of DA neurons in the periaqueductal gray/dorsal raphe: a role in regulation of pain. Neuropsychopharmacology 41, 2122–2132. doi: 10.1038/NPP.2016.12
Li, X., He, L., Liu, J., Guo, W., Wang, Q., Fang, P., et al. (2020). The rs6311 of 5-HT receptor 2A (5-HT2A) gene is associated with alexithymia and mental health. J. Affect. Disord. 272, 277–282. doi: 10.1016/j.jad.2020.03.153
Liang, F., Xu, Q., Jiang, M., Feng, R., Jiang, S., Yuan, B., et al. (2021). Emotion induced monoamine neuromodulator release affects functional neurological disorders. Front. Cell Dev. Biol. 9:633048. doi: 10.3389/fcell.2021.633048
Lisman, J. E., and Grace, A. A. (2005). The hippocampal-VTA loop: controlling the entry of information into long-term memory. Neuron 46, 703–713. doi: 10.1016/J.NEURON.2005.05.002
Liu, Z., Zhou, J., Li, Y., Hu, F., Lu, Y., Ma, M., et al. (2014). Dorsal raphe neurons signal reward through 5-HT and glutamate. Neuron 81, 1360–1374. doi: 10.1016/j.neuron.2014.02.010
Liu, Z. H., Shin, R., and Ikemoto, S. (2008). Dual role of medial A10 DA neurons in affective encoding. Neuropsychopharmacology 33, 3010–3020. doi: 10.1038/npp.2008.4
Logan, R. W., and McClung, C. A. (2019). Rhythms of life: circadian disruption and brain disorders across the lifespan. Nat. Rev. Neurosci. 20, 49–65. doi: 10.1038/s41583-018-0088-y
Ludwig, L., Pasman, J. A., Nicholson, T., Aybek, S., David, A. S., Tuck, S., et al. (2018). Stressful life events and maltreatment in conversion (functional neurological) disorder: systematic review and meta-analysis of case-control studies. Lancet Psychiatry 5, 307–320. doi: 10.1016/s2215-0366(18)30051-8
Luo, R., Uematsu, A., Weitemier, A., Aquili, L., Koivumaa, J., McHugh, T. J., et al. (2018). A DArgic switch for fear to safety transitions. Nat. Commun. 9:2483. doi: 10.1038/s41467-018-04784-7
Lutas, A., Kucukdereli, H., Alturkistani, O., Carty, C., Sugden, A. U., Fernando, K., et al. (2019). State-specific gating of salient cues by midbrain DArgic input to basal amygdala. Nat. Neurosci. 22, 1820–1833. doi: 10.1038/s41593-019-0506-0
Mahmoudi, S., Lévesque, D., and Blanchet, P. J. (2014). Upregulation of DA D3, not D2, receptors correlates with tardive dyskinesia in a primate model. Mov. Disord. 29, 1125–1133. doi: 10.1002/MDS.25909
Mallet, N., Ballion, B., Moine, C. L., and Gonon, F. (2006). Cortical inputs and GABA interneurons imbalance projection neurons in the striatum of parkinsonian rats. J. Neurosci. 26, 3875–3884. doi: 10.1523/JNEUROSCI.4439-05.2006
Mandalaywala, T. M., Petrullo, L. A., Parker, K. J., Maestripieri, D., and Higham, J. P. (2017). Vigilance for threat accounts for inter-individual variation in physiological responses to adversity in rhesus macaques: a cognition x environment approach. Dev. Psychobiol. 59, 1031–1038. doi: 10.1002/dev.21572
Martins, F., Oppolzer, D., Santos, C., Barroso, M., and Gallardo, E. (2019). Opioid use in pregnant women and neonatal abstinence syndrome-a review of the literature. Toxics 7:9. doi: 10.3390/toxics7010009
Matsumoto, M., and Hikosaka, O. (2009). Two types of DA neuron distinctly convey positive and negative motivational signals. Nature 459, 837–841. doi: 10.1038/nature08028
Matthews, G. A., Nieh, E. H., Weele, C. M. V., Halbert, S. A., Pradhan, R. V., Yosafat, A. S., et al. (2016). Dorsal raphe DA neurons represent the experience of social isolation. Cell 164, 617–631. doi: 10.1016/J.CELL.2015.12.040
McCall, J. G., Al-Hasani, R., Siuda, E. R., Hong, D. Y., Norris, A. J., Ford, C. P., et al. (2015). CRH engagement of the locus coeruleus noradrenergic system mediates stress-induced anxiety. Neuron 87, 605–620. doi: 10.1016/j.neuron.2015.07.002
McHugh, S. B., Barkus, C., Huber, A., Capitão, L., Lima, J., Lowry, J. P., et al. (2014). Aversive prediction error signals in the amygdala. J. Neurosci. 34, 9024–9033. doi: 10.1523/JNEUROSCI.4465-13.2014
McKinley, J. W., Shi, Z., Kawikova, I., Hur, M., Bamford, I. J., Sudarsana Devi, S. P., et al. (2019). DA deficiency reduces striatal cholinergic interneuron function in models of PD. Neuron 103, 1056–1072.e6. doi: 10.1016/j.neuron.2019.06.013
McNally, G. P., and Cole, S. (2006). Opioid receptors in the midbrain periaqueductal gray regulate prediction errors during Pavlovian fear conditioning. Behav. Neurosci. 120, 313–323. doi: 10.1037/0735-7044.120.2.313
Mei, C. D., Ramos, M., Iitaka, C., and Borrelli, E. (2009). Getting specialized: presynaptic and postsynaptic DA D2 receptors. Curr. Opin. Pharmacol. 9, 53–58. doi: 10.1016/J.COPH.2008.12.002
Menegas, W., Akiti, K., Amo, R., Uchida, N., and Watabe-Uchida, M. (2018). DA neurons projecting to the posterior striatum reinforce avoidance of threatening stimuli. Nat. Neurosci. 21, 1421–1430. doi: 10.1038/s41593-018-0222-1
Menegas, W., Babayan, B. M., Uchida, N., and Watabe-Uchida, M. (2017). Opposite initialization to novel cues in DA signaling in ventral and posterior striatum in mice. eLife 6:e21886. doi: 10.7554/ELIFE.21886
Menegas, W., Bergan, J. F., Ogawa, S. K., Isogai, Y., Venkataraju, K. U., Osten, P., et al. (2015). DA neurons projecting to the posterior striatum form an anatomically distinct subclass. eLife 4:e10032. doi: 10.7554/ELIFE.10032
Mileykovskiy, B., and Morales, M. (2011). Duration of inhibition of ventral tegmental area DA neurons encodes a level of conditioned fear. J. Neurosci. 31, 7471–7476. doi: 10.1523/JNEUROSCI.5731-10.2011
Mingote, S., Amsellem, A., Kempf, A., Rayport, S., and Chuhma, N. (2019). DA-glutamate neuron projections to the nucleus accumbens medial shell and behavioral switching. Neurochem. Int. 129:104482. doi: 10.1016/j.neuint.2019.104482
Mitrano, D. A., Schroeder, J. P., Smith, Y., Cortright, J. J., Bubula, N., Vezina, P., et al. (2012). alpha-1 adrenergic receptors are localized on presynaptic elements in the nucleus accumbens and regulate mesolimbic DA transmission. Neuropsychopharmacology 37, 2161–2172. doi: 10.1038/npp.2012.68
Morales, M., and Margolis, E. B. (2017). Ventral tegmental area: cellular heterogeneity, connectivity and behaviour. Nat. Rev. Neurosci. 18, 73–85. doi: 10.1038/nrn.2016.165
Murphy, G. H. (2008). The use of aversive stimuli in treatment: the issue of consent∗. J. Intellect. Disabil. Res. 37, 211–219. doi: 10.1111/J.1365-2788.1993.TB01280.X
Nestler, E. J., and Aghajanian, G. K. (1997). Molecular and cellular basis of addiction. Science 278, 58–63. doi: 10.1126/science.278.5335.58
Pajonk, F.-G. (2004). Risperidone in acute and long-term therapy of schizophrenia—a clinical profile. Prog. Neuro-Psychopharmacol. Biol. Psychiatry 28, 15–23. doi: 10.1016/s0278-5846(03)00164-7
Pascucci, T., Ventura, R., Latagliata, E. C., Cabib, S., and Puglisi-Allegra, S. (2007). The medial prefrontal cortex determines the accumbens DA response to stress through the opposing influences of norepinephrine and DA. Cereb. Cortex 17, 2796–2804. doi: 10.1093/CERCOR/BHM008
Pezze, M. A., and Feldon, J. (2004). Mesolimbic DArgic pathways in fear conditioning. Prog. Neurobiol. 74, 301–320. doi: 10.1016/J.PNEUROBIO.2004.09.004
Pick, S., Goldstein, L. H., Perez, D. L., and Nicholson, T. R. (2019). Emotional processing in functional neurological disorder: a review, biopsychosocial model and research agenda. J. Neurol. Neurosurg. Psychiatry 90, 704–711. doi: 10.1136/jnnp-2018-319201
Pignatelli, M., and Bonci, A. (2015). Role of DA neurons in reward and aversion: a synaptic plasticity perspective. Neuron 86, 1145–1157. doi: 10.1016/j.neuron.2015.04.015
Ramboz, S., Oosting, R., Amara, D. A., Kung, H. F., Blier, P., Mendelsohn, M., et al. (1998). 5-HT receptor 1A knockout: an animal model of anxiety-related disorder. Proc. Natl. Acad. Sci. U S A. 95, 14476–14481. doi: 10.1073/PNAS.95.24.14476
Ravina, B., Marder, K., Fernandez, H. H., Friedman, J. H., McDonald, W., Murphy, D., et al. (2007). Diagnostic criteria for psychosis in PD: report of an NINDS. NIMH work group. Mov. Disord. 22, 1061–1068. doi: 10.1002/MDS.21382
Redgrave, P., and Gurney, K. (2006). The short-latency DA signal: a role in discovering novel actions? Nat. Rev. Neurosci. 7, 967–975. doi: 10.1038/NRN2022
Roberts, B. M., Lopes, E. F., and Cragg, S. J. (2021). Axonal modulation of striatal DA release by local gamma-aminobutyric acid (GABA) signalling. Cells 10:709. doi: 10.3390/cells10030709
Roitman, M. F., Wheeler, R. A., Wightman, R. M., and Carelli, R. M. (2008). Real-time chemical responses in the nucleus accumbens differentiate rewarding and aversive stimuli. Nat. Neurosci. 11, 1376–1377. doi: 10.1038/nn.2219
Rolland, B., Jardri, R., Amad, A., Thomas, P., Cottencin, O., and Bordet, R. (2014). Pharmacology of hallucinations: several mechanisms for one single symptom? Biomed. Res. Int. 2014:307106. doi: 10.1155/2014/307106
Rondou, P., Haegeman, G., and Craenenbroeck, K. V. (2010). The DA D4 receptor: biochemical and signalling properties. Cell. Mol. Life Sci. 67, 1971–1986. doi: 10.1007/S00018-010-0293-Y
Roy, M., Shohamy, D., Daw, N., Jepma, M., Wimmer, G. E., and Wager, T. D. (2014). Representation of aversive prediction errors in the human periaqueductal gray. Nat. Neurosci. 17, 1607–1612. doi: 10.1038/nn.3832
Salamone, J. D. (1992). Complex motor and sensorimotor functions of striatal and accumbens DA: involvement in instrumental behavior processes. psychopharmacology 107, 160–174. doi: 10.1007/BF02245133
Salamone, J. D., and Correa, M. (2012). The mysterious motivational functions of mesolimbic DA. Neuron 76, 470–485. doi: 10.1016/j.neuron.2012.10.021
Salamone, J. D., Mahan, K., and Rogers, S. (1993). Ventrolateral striatal DA depletions impair feeding and food handling in rats. Pharmacol. Biochem. Behav. 44, 605–610. doi: 10.1016/0091-3057(93)90174-R
Salamone, J. D. (2010). “Motor function and motivation,” in Encyclopedia of Behavioral Neuroscience, (Eds.) G. F. Koob, M. LeMoal, and R. F. Thompson (Oxford: Academic Press), 3.
Sarinana, J., Kitamura, T., Kunzler, P., Sultzman, L., and Tonegawa, S. (2014). Differential roles of the DA 1-class receptors, D1R and D5R, in hippocampal dependent memory. Proc. Natl. Acad. Sci. U S A. 111, 8245–8250. doi: 10.1073/pnas.1407395111
Saunders, B. T., Richard, J. M., Margolis, E. B., and Janak, P. H. (2018). DA neurons create Pavlovian conditioned stimuli with circuit-defined motivational properties. Nat. Neurosci. 21, 1072–1083. doi: 10.1038/S41593-018-0191-4
Schopenhauer, W. (1999). “Prize essay on the freedom of the will,” in Cambridge Texts in the History of Philosophy, ed. G. Zoller, trans. Payne, E.F.J (Cambridge: Cambridge University Press).
Schrag, A. E., Mehta, A. R., Bhatia, K. P., Brown, R. J., Frackowiak, R. S., Trimble, M. R., et al. (2013). The functional neuroimaging correlates of psychogenic versus organic dystonia. Brain 136(Pt 3), 770–781. doi: 10.1093/brain/awt008
Schultz, W. (2007). Multiple DA functions at different time courses. Annu. Rev. Neurosci. 30, 259–288. doi: 10.1146/ANNUREV.NEURO.28.061604.135722
Schultz, W. (2010). DA signals for reward value and risk: basic and recent data. Behav. Brain Funct. 6, 24–24. doi: 10.1186/1744-9081-6-24
Schultz, W. (2015). Neuronal reward and decision signals: from theories to data. Physiol. Rev. 95, 853–951. doi: 10.1152/physrev.00023.2014
Schultz, W., Dayan, P., and Montague, P. R. (1997). A neural substrate of prediction and reward. Science 275, 1593–1599. doi: 10.1126/science.275.5306.1593
Seeman, P. (2006). Targeting the DA D2 receptor in schizophrenia. Expert Opin. Ther. Targets 10, 515–531. doi: 10.1517/14728222.10.4.515
Shao, D., Cao, Z., Fu, Y., Yang, H., Gao, P., Zheng, P., et al. (2021). Projection from the basolateral amygdala to the anterior cingulate cortex facilitates the consolidation of long-term withdrawal memory. Add. Biol. Online ahead of print.
Shen, L., Yan, M., and He, L. (2016). D5 receptor agonist 027075 promotes cognitive function recovery and neurogenesis in a Aβ1-42-induced mouse model. Neuropharmacology 105, 72–83. doi: 10.1016/J.NEUROPHARM.2016.01.008
Sibley, D. R. (1999). New insights into DArgic receptor function using antisense and genetically altered animals. Annu. Rev. Pharmacol. Toxicol. 39, 313–341. doi: 10.1146/ANNUREV.PHARMTOX.39.1.313
Spano, P. F., Govoni, S., and Trabucchi, M. (1978). Studies on the pharmacological properties of DA receptors in various areas of the central nervous system. Adv. Biochem. Psychopharmacol. 19, 155–165.
Stahl, S. M. (2016). PD psychosis as a 5-HT-DA imbalance syndrome. CNS Spectr. 21, 355–359. doi: 10.1017/S1092852916000602
Stahl, S. M. (2018). Beyond the DA hypothesis of schizophrenia to three neural networks of psychosis: DA, 5-HT, and glutamate. CNS Spectr. 23, 187–191. doi: 10.1017/S1092852918001013
Stiedl, O., Jansen, R. F., Pieneman, A. W., Ogren, S. O., and Meyer, M. (2009). Assessing aversive emotional states through the heart in mice: implications for cardiovascular dysregulation in affective disorders. Neurosci. Biobehav. Rev. 33, 181–190. doi: 10.1016/j.neubiorev.2008.08.015
Suárez, L. M., Solís, O., Carames, J. M., Taravini, I. R. E., Solis, J. M., Murer, M. G., et al. (2014). L-DOPA treatment selectively restores spine density in DA receptor D2-expressing projection neurons in dyskinetic mice. Biol. Psychiatry 75, 711–722. doi: 10.1016/J.BIOPSYCH.2013.05.006
Sullivan, M. D., and Ballantyne, J. C. (2021). When physical and social pain coexist: insights into opioid therapy. Ann. Fam. Med. 19, 79–82. doi: 10.1370/afm.2591
Szaflarski, J. P., Allendorfer, J. B., Nenert, R., LaFrance, W. C. Jr., Barkan, H. I., et al. (2018). Facial emotion processing in patients with seizure disorders. Epilepsy Behav. 79, 193–204. doi: 10.1016/j.yebeh.2017.12.004
Tan, K. R., Yvon, C., Turiault, M., Mirzabekov, J. J., Doehner, J., Labouèbe, G., et al. (2012). GABA neurons of the VTA drive conditioned place aversion. Neuron 73, 1173–1183. doi: 10.1016/J.NEURON.2012.02.015
Tian, J., Huang, R., Cohen, J. Y., Osakada, F., Kobak, D., Machens, C. K., et al. (2016). Distributed and mixed information in monosynaptic inputs to DA neurons. Neuron 91, 1374–1389. doi: 10.1016/j.neuron.2016.08.018
Tian, J., and Uchida, N. (2015). Habenula lesions reveal that multiple mechanisms underlie DA prediction errors. Neuron 87, 1304–1316. doi: 10.1016/j.neuron.2015.08.028
Tovote, P., Esposito, M. S., Botta, P., Chaudun, F., Fadok, J. P., Markovic, M., et al. (2016). Midbrain circuits for defensive behaviour. Nature 534, 206–212. doi: 10.1038/NATURE17996
Tye, K. M., Mirzabekov, J. J., Warden, M. R., Ferenczi, E. A., Tsai, H.-C., Finkelstein, J., et al. (2013). DA neurons modulate neural encoding and expression of depression-related behaviour. Nature 493, 537–541. doi: 10.1038/NATURE11740
Ungless, M. A. (2004). DA: the salient issue. Trends Neurosci. 27, 702–706. doi: 10.1016/J.TINS.2004.10.001
Usiello, A., Baik, J. H., Rougé-Pont, F., Picetti, R., Dierich, A., LeMeur, M., et al. (2000). Distinct functions of the two isoforms of DA D2 receptors. Nature 408, 199–203. doi: 10.1038/35041572
Valentino, R. J., and Van Bockstaele, E. (2015). Endogenous opioids: the downside of opposing stress. Neurobiol. Stress 1, 23–32. doi: 10.1016/j.ynstr.2014.09.006
Vallone, D., Picetti, R., and Borrelli, E. (2000). Structure and function of DA receptors. Neurosci. Biobehav. Rev. 24, 125–132. doi: 10.1016/S0149-7634(99)00063-9
Vander Weele, C. M., Siciliano, C. A., Matthews, G. A., Namburi, P., Izadmehr, E. M., Espinel, I. C., et al. (2018). DA enhances signal-to-noise ratio in cortical-brainstem encoding of aversive stimuli. Nature 563, 397–401. doi: 10.1038/s41586-018-0682-1
Velasquez-Martinez, M. C., Santos-Vera, B., Velez-Hernandez, M. E., Vazquez-Torres, R., and Jimenez-Rivera, C. A. (2020). Alpha-1 adrenergic receptors modulate glutamate and GABA neurotransmission onto ventral tegmental DA neurons during cocaine sensitization. Int. J. Mol. Sci. 21:790. doi: 10.3390/ijms21030790
Velasquez-Martinez, M. C., Vazquez-Torres, R., Rojas, L. V., Sanabria, P., and Jimenez-Rivera, C. A. (2015). Alpha-1 adrenoreceptors modulate GABA release onto ventral tegmental area DA neurons. Neuropharmacology 88, 110–121. doi: 10.1016/j.neuropharm.2014.09.002
Ventura, R., Cabib, S., and Puglisi-Allegra, S. (2001). Opposite genotype-dependent mesocorticolimbic DA response to stress. Neuroscience 104, 627–631. doi: 10.1016/S0306-4522(01)00160-9
Verharen, J. P., Zhu, Y., and Lammel, S. (2020). Aversion hot spots in the DA system. Curr. Opin. Neurobiol. 64, 46–52. doi: 10.1016/J.CONB.2020.02.002
Vollenweider, F. X., Vollenweider-Scherpenhuyzen, M. F. I., Bäbler, A., Vogel, H., and Hell, D. (1998). Psilocybin induces schizophrenia-like psychosis in humans via a 5-HT-2 agonist action. Neuroreport 9, 3897–3902. doi: 10.1097/00001756-199812010-00024
Voon, V., Brezing, C., Gallea, C., Ameli, R., Roelofs, K., LaFrance, W. C., et al. (2010). Emotional stimuli and motor conversion disorder. Brain 133(Pt 5), 1526–1536. doi: 10.1093/brain/awq054
Voon, V., Cavanna, A. E., Coburn, K., Sampson, S., Reeve, A., and LaFrance, W. C. Jr. (2016). Functional neuroanatomy and neurophysiology of functional neurological disorders (Conversion Disorder). J. Neuropsychiatry Clin. Neurosci. 28, 168–190. doi: 10.1176/appi.neuropsych.14090217
Wagatsuma, A., Okuyama, T., Sun, C., Smith, L. M., Abe, K., and Tonegawa, S. (2018). Locus coeruleus input to hippocampal CA3 drives single-trial learning of a novel context. Proc. Natl. Acad. Sci. U S A. 115, E310–E316. doi: 10.1073/pnas.1714082115
Wang, F., Yang, J., Pan, F., Ho, R. C., and Huang, J. H. (2020). Editorial: neurotransmitters and emotions. Front. Psychol. 11:21. doi: 10.3389/fpsyg.2020.00021
Wang, J., Wang, F., Mai, D., and Qu, S. (2020). Molecular mechanisms of glutamate toxicity in PD. Front. Neurosci. 14:585584. doi: 10.3389/fnins.2020.585584
Weissbourd, B., Ren, J., DeLoach, K. E., Guenthner, C. J., Miyamichi, K., and Luo, L. (2014). Presynaptic partners of dorsal raphe serotonergic and GABAergic neurons. Neuron 83, 645–662. doi: 10.1016/j.neuron.2014.06.024
Williams-Gray, C. H., and Worth, P. F. (2016). PD. Medicine 44, 542–546. doi: 10.1016/j.mpmed.2016.06.001
Xia, Q. P., Cheng, Z. Y., and He, L. (2019). The modulatory role of DA receptors in brain neuroinflammation. Int. Immunopharmacol. 76:105908. doi: 10.1016/j.intimp.2019.105908
Yagishita, S. (2020). Transient and sustained effects of DA and 5-HT signaling in motivation-related behavior. Psychiatry Clin. Neurosci. 74, 91–98. doi: 10.1111/pcn.12942
Yamaguchi, T., Qi, J., Wang, H.-L., Zhang, S., and Morales, M. (2015). Glutamatergic and DArgic neurons in the mouse ventral tegmental area. Eur. J. Neurosci. 41, 760–772. doi: 10.1111/EJN.12818
Yang, Y., Cui, Y., Sang, K., Dong, Y., Ni, Z., Ma, S., et al. (2018). Ketamine blocks bursting in the lateral habenula to rapidly relieve depression. Nature 554, 317–322. doi: 10.1038/nature25509
Yuan, L., Dou, Y.-N., and Sun, Y.-G. (2019). Topography of reward and aversion encoding in the mesolimbic DArgic system. J. Neurosci. 39, 6472–6481. doi: 10.1523/JNEUROSCI.0271-19.2019
Zareifopoulos, N., and Papatheodoropoulos, C. (2016). Effects of 5-HT-7 receptor ligands on memory and cognition. Neurobiol. Learn. Mem. 136, 204–209. doi: 10.1016/j.nlm.2016.10.011
Zhai, S., Shen, W., Graves, S. M., and Surmeier, D. J. (2019). DArgic modulation of striatal function and PD. J. Neural Transm. (Vienna) 126, 411–422. doi: 10.1007/s00702-019-01997-y
Zhang, H., Chaudhury, D., Nectow, A. R., Friedman, A. K., Zhang, S., Juarez, B., et al. (2019). alpha1- and beta3-Adrenergic receptor-mediated mesolimbic homeostatic plasticity confers resilience to social stress in susceptible mice. Biol. Psychiatry 85, 226–236. doi: 10.1016/j.biopsych.2018.08.020
Keywords: limbic system, DA, aversion function, prediction error, functional neurological disorders
Citation: He Z, Jiang Y, Gu S, Wu D, Qin D, Feng G, Ma X, Huang JH and Wang F (2021) The Aversion Function of the Limbic Dopaminergic Neurons and Their Roles in Functional Neurological Disorders. Front. Cell Dev. Biol. 9:713762. doi: 10.3389/fcell.2021.713762
Received: 24 May 2021; Accepted: 07 July 2021;
Published: 20 September 2021.
Edited by:
Guohui Lu, The First Affiliated Hospital of Nanchang University, ChinaReviewed by:
Ying Xu, University at Buffalo, United StatesYuan-Xiang Tao, Rutgers, The State University of New Jersey, United States
Biao Zuo, University of Pennsylvania, United States
Copyright © 2021 He, Jiang, Gu, Wu, Qin, Feng, Ma, Huang and Wang. This is an open-access article distributed under the terms of the Creative Commons Attribution License (CC BY). The use, distribution or reproduction in other forums is permitted, provided the original author(s) and the copyright owner(s) are credited and that the original publication in this journal is cited, in accordance with accepted academic practice. No use, distribution or reproduction is permitted which does not comply with these terms.
*Correspondence: Xianjun Ma, maxianjun@126.com; Simeng Gu, gsm_2007@126.com; Fushun Wang, 13814541138@163.com