Mesenchymal Stem Cell Immunomodulation: A Novel Intervention Mechanism in Cardiovascular Disease
- 1First Teaching Hospital of Tianjin University of Traditional Chinese Medicine, Tianjin, China
- 2National Clinical Research Center for Chinese Medicine Acupuncture and Moxibustion, Tianjin, China
- 3Institute of Gerontology, Xiyuan Hospital, China Academy of Chinese Medical Sciences, Beijing, China
Mesenchymal stem cells (MSCs) are the member of multipotency stem cells, which possess the capacity for self-renewal and multi-directional differentiation, and have several characteristics, including multi-lineage differentiation potential and immune regulation, which make them a promising source for cell therapy in inflammation, immune diseases, and organ transplantation. In recent years, MSCs have been described as a novel therapeutic strategy for the treatment of cardiovascular diseases because they are potent modulators of immune system with the ability to modulating immune cell subsets, coordinating local and systemic innate and adaptive immune responses, thereby enabling the formation of a stable inflammatory microenvironment in damaged cardiac tissues. In this review, the immunoregulatory characteristics and potential mechanisms of MSCs are sorted out, the effect of these MSCs on immune cells is emphasized, and finally the application of this mechanism in the treatment of cardiovascular diseases is described to provide help for clinical application.
1 Introduction
Mesenchymal stem cells (MSCs) are self-sustaining stromal cells that are mainly found in bone marrow (BM), adipose tissue, fat, and umbilical cord. The multipotency of MSCs makes them an attractive therapeutic tool for organ transplantation in modern medicine (Ding et al., 2011). Currently, MSCs have been considered as a potential measure for the treatment of autoimmune and inflammatory diseases. Notably, clinical and experimental studies have demonstrated the therapeutic role of MSCs in cellular immunomodulation, organ-specific, and systemic inflammatory diseases. Recently, it has been demonstrated that MSCs can regulate the immune response of the body by regulating macrophage polarization, producing immunosuppressive molecules, and stimulating metabolites (Harrell et al., 2019a).
Cardiovascular diseases are currently the leading cause of death worldwide (Wang and Zhao. 2018). They are ischemic heart diseases that occur in the heart and its surrounding vessels, caused by lesions such as atherosclerosis, hypertension, and hyperlipidemia (Albany et al., 2019). MSCs have been widely concerned as a potential treatment for cardiovascular disease due to their potential to repair cardiac injury. Meta-analysis of animal studies has demonstrated that MSCs are safe and effective in the treatment of ischemic heart disease and have a great improvement in left ventricular fraction (LVEF). In previous studies, MSCs have been reported to repair damaged myocardium, promote myocardial regeneration, and restore normal cardiac function. They secrete various cytokines such as epidermal growth factor through the paracrine pathway for cardiac repair and regulate the expression of immune cells and related inflammatory cells to alleviate the inflammatory response after myocardial injury. Therefore, MSCs play a role in protecting cardiac function and treating cardiovascular diseases (Liu et al., 2020). This review explains the immunomodulatory effects of MSCs and their role in cardiovascular diseases, in order to provide help for the application of bone marrow stem cells in cardiovascular disease.
2 Overview of Mesenchymal Stem Cells
MSCs are early cells in mesoderm development and have differentiation potential, which were discovered by Alexander Friedenstein in the late 1960s (Uccelli et al., 2008; Spees et al., 2016). At present, the stem cells that can be used for research mainly include human umbilical cord mesenchymal stem cells (UCMSCs), adipose-derived mesenchymal stem cells (ADMSCs), BM mesenchymal stem cells (BMMSCs), and placenta-derived mesenchymal stem cells (PlaMSCs) (Li et al., 2015a). MSCs have high proliferation and self-renewal ability and can differentiate into neural cells, cardiomyocytes, blood cells, and other cells at different development stages and in different environments (He et al., 2013). MSCs are capable of repairing tissue damage through their differentiation function, and can also express a variety of characteristic immunophenotypes, such as CD105\CD105\CD90\CD44\CD71 (J and Debabrata. 2017).
MSCs secrete immunomodulatory factors, cytokines, growth factors, extracellular vesicles (EV) and other bioactive factors such as, which anti-apoptotic, anti-fibrotic, antioxidant and immunomodulatory effects and regulate a series of physiological processes. MSCs have been shown to secrete cytokines such as Chemokine C-C motif ligand 2 (CCL-2), Chemokine C-C motif ligand 5 (CCL-5), Insulin-like growth factor-1 (IGF-1), Interleukin-6 (IL-6), and Vascular endothelial growth factor (VEGF), which are involved in cell and tissue development, differentiation, and death (Wang et al., 2019). MSCs perform immune regulation by secreting cytokines such as IL-6, Interleukin-10 (IL-10), prostaglandin E2 (PGE2) and transforming growth factor-beta (TGF-β) (Lopatina et al., 2019), and can also participatein intercellular communication by secreting exosomes with immunomodulatory characteristics, thereby facilitating the immune system to recognize and eliminate antigenic foreign bodies (Lee et al., 2012). The chemokines of MSCs are mainly CXC chemokine receptor 3 (CXCR3) and CC chemokine receptor 5 (CCR5) ligands, as well as CXC chemokine ligand 9 (CXCR9), CXC chemokine ligand 10 (CXCR10), and CXC chemokine ligand 11(CXCR11). They are well-known immune cell chemotactic agents capable of modulating immune cells, such as T lymphocytes (T cells) (Shi et al., 2018a). MSCs regulate the immune response by regulating the activity of T cells and B -lymphocytes (B cells), thus inhibiting cell apoptosis. They interact with the immune system to induce the immunosuppressive activity of many immune cells, including lymphocytes, antigen-presenting cells, and natural killer cells (NK cells). In recent years, MSCs have gained much attention due to their potential application value in the treatment of autoimmune diseases (Table 1).
3 Modulation of Different Immune Cells by Mesenchymal Stem Cells
3.1 Macrophages
Macrophages are essential components of the human immune system, which can perform different functions in the body’s immune response, such as regulating apoptosis, phagocytosing of pathogens, remodeling the extracellular matrix, activating other immune cells, and so on. Under various stimuli, macrophages can polarize into two phenotypes, M1 and M2 (Wynn et al., 2013; Koelwyn et al., 2018). Among the two phenotypes of macrophages, classical M1-polarized macrophages can promote the secretion of pro-inflammatory factors, promote the antibacterial reaction, and alternative M2-polarized macrophages [activated by Interleukin-4 (IL-4) and Interleukin-13 (IL-13)], which are generally considered to have the immunosuppressive effect (Wang et al., 2016a). MSCs inhibit macrophages activation and transform the M1 phenotype to the M2 phenotype.
3.1.1 Regulation by Related Proteins and Inflammatory Factors
BMMSCs injected into the sulfur mustard-induced acute lung injury mouse model, the proportion of anti-inflammatory M2 macrophages was substantially increased, whereas that of M1 macrophages was decreased in BMMSCs-treated mice compared with the sulfur mustard group, and the expression of Toll-like receptor 4 (TLR4) greatly increased, that indicated BMMSCs can inhibit the differentiation of macrophages into pro-inflammatory M1 macrophages through the TRL4 signaling pathway and promote the differentiation of macrophages to anti-inflammatory M2 macrophages (Feng et al., 2019). Yap protein in the Hippo pathway can regulate the inflammatory response. MSCs control NLRP3 inflammasome assembly by activating the Hippo pathway in macrophages and regulating the interaction between yap and β-catenin (Li et al., 2019).
On the other hand, the immunomodulatory effect of MSCs can be achieved by secreting inflammatory factors. TGF-β secreted by MSCs activates the Akt/Forkhead box transcription factor O1 (FoxO1) pathway in mice macrophages with high body oxygen levels, alveolar fibrosis, and pulmonary vascular remodeling (Liu et al., 2019). Inflammatory chemokines CCL2 and CXC ligand 2 (CXCL2) secreted by BMMSCs play an essential role in polarizing mouse peritoneal macrophages into the IL-10 phenotype (Giri et al., 2020).
3.1.2 Regulation by Secreted Exosomes
MSCs secrete vesicles with a diameter of 40–100 nm, which are called exosomes. Exosomes contain a large amount of biologically active substances, like cytokines, proteins, miRNAs, DNA, and other biologically active substances. Exosomes from BMMSCs were extracted, some of them were treated with lipopolysaccharide (LPS), the results indicated that both the normal MSCs exosomes and the LPS-treated MSCs exosomes considerably reduced the positive rate of the M1 macrophages protein marker CD11 and increased the positive rate of the M2 macrophages protein marker CD206. Exosomes derived from MSCs are able to regulate macrophages polarization by inhibiting the Nuclear Factor Kappa-B (NF-κB) pathway and activating the Serine threonine-specific protein kinase (Akt1/Akt2) pathway, thereby reducing post-infarction inflammation and cardiomyocytes apoptosis (Xu et al., 2019). In the steroid-resistant mouse model, Bing Dong and his colleagues found that intratracheal administration of exosomes treatment reduced inflammation and reduced M1 macrophages, and promoted M2 macrophages. This effect could achieve by inhibiting the TNF receptor-associated factor 1 (TRAF1) (Dong et al., 2021). In this way, MSCs promote the polarization of macrophages from a pro-inflammatory M1 phenotype to an anti-inflammatory M2 phenotype and regulate through related proteins, exosomes, and inflammatory factors.
3.2 Dendritic Cells
Dendritic cells are individual antigen-presenting cells. They are heterogeneous population of leukocytes with different subpopulations, which are responsible for driving specific immune responses and initiating and modulating adaptive immune responses (Lee and Radford. 2019).
3.2.1 Regulation of the Proliferation, Maturation, and Differentiation of Dendritic Cells
MSCs are capable of inhibiting the proliferation, maturation, and differentiation of DCs, thus exerting immunomodulatory and immunosuppressive effects (De Miguel et al., 2012). MSCs and DCs derived from mouse bone marrow were co-cultured, and the results showed that MSCs derived exosomes were able to reduce the expression of surface markers, and inhibit the maturation of DCs treated with LPS (Zhang et al., 2017). TGF-β1 expressing lentiviruses were used for MSCs transduction, and then these MSCs were co-cultured with T cells and DCs, and the results showed that MSCs derived exosomes were able to inhibit the maturation of DCs through TGF-β and had an immunosuppressive effect (Daneshmandi et al., 2017).
DCs have numerous phenotypes, including major histocompatibility complex I (MHC-I) and major histocompatibility complex II (MHC-II) as well as CD370, CD207, CD205, CD1a, CD11c, CD11b, CD83, and CD40 (Clark et al., 2019). Hypoxia-inducible factor (HIF) exert significant biological effects such as angiogenesis and cell survival. Transduction of MSCs with GFP-HIF-1α lentiviral vector (HIF-MSCs) followed by co-culture with DCs revealed that HIF-MSCs significantly decreased DCs differentiation and increase resistance to NK cell lysis (Martinez et al., 2017).
3.2.2 Regulation of the Interaction Between Dendritic Cells and Lymphocytes
Dendritic cells can initiate T cells immunoregulation, and they regulate T cells differentiation toward Th1, Th2, Th17, or Tregs subpopulations. DCs also indirectly regulate T cells subpopulation differentiation by activating intrinsic lymphocytes to produce regulatory cytokines (Briseño et al., 2014). Immature dendritic cells (Im-DCs) and LPS-treated DCs were co-cultured with MSCs for 48 h. The expression of CD11c, CD80, CD86, and IL-6, Tumor necrosis factor α (TNF-α), and IFN-γ was decreased, while CD11b, IL-10, and TGF-β expression was largely increased and stimulated splenocytes to produce markers of Tregs (FOXP3, CD4, and CD25). Thus, MSCs can induce the transformation of immature DC phenotypes to regulatory dendritic cells (r-DCs), and MSCs which secrete anti-inflammatory cytokines (IL-10 and TGF-β) play a similar role to r-DCs, leading to the activation of Tregs (Jo et al., 2018). Tumor MSCs were able to inhibit DCs’ expression of cysteine through Signal transduction and activation protein 3 (STAT3), thereby preventing DCs from promoting the expansion of naive CD4+ and CD8+ T cells (Ghosh et al., 2016). So, MSCs regulate immune regulation by regulating the differentiation and maturation of DCs and the interaction between DCs and lymphocytes (Figure 1).
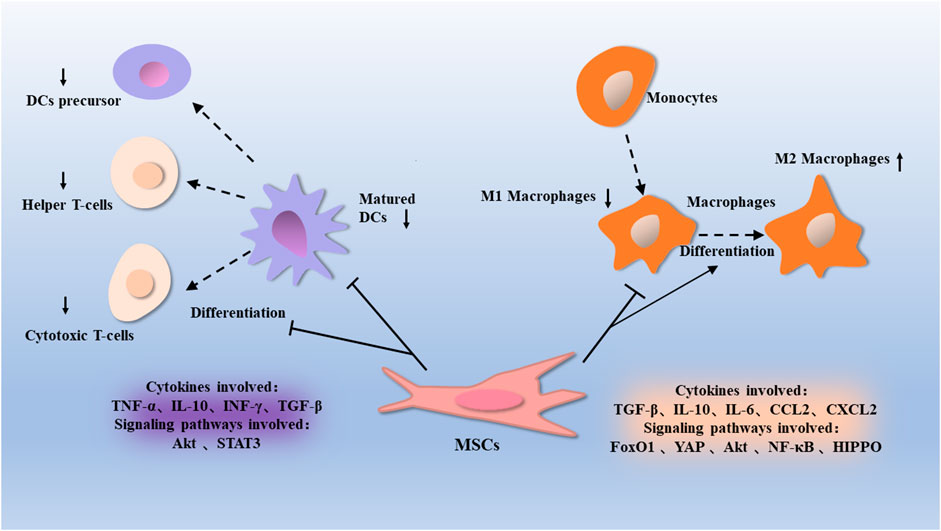
FIGURE 1. Macrophages are a type of immune cells that differentiate from differentiated monocytes derived from MSCs. MSCs inhibit macrophages activation through proteins such as Akt, FoxO1, NF-κB, and Hippo, can promote the conversion of macrophages from a pro-inflammatory M1 phenotype to an anti-inflammatory M2 phenotype. This is associated with factors such as TGF-β, IL-10, IL-6, CCL2, and CXCL2. Dendritic cells are differentiated from dendritic cell precursors and regulate the conversion of T cells to helper T cells and cytotoxic T cells. MSCs inhibit the proliferation of dendritic cells through proteins such as Akt and STAT3, thus affecting their regulatory T-cell function, which is associated with factors such as TNF-α, IL-10, INF γ, and TGF-β.
3.3 T Lymphocytes
MSCs are allowed to mediate immune responses by regulating T cells’ activity. T cells are immune cells that originate from the BM and differentiate and mature in the thymus gland. T cells are essential for the body’s immune response, maintenance of homeostasis, maintenance of immune memory, and recognition of pathogens (Kumar et al., 2018).
3.3.1 Regulation of the Activation and Proliferation of T Lymphocytes
Studies have shown that MSCs modulate immunity through T cells. Cytochalasin B-induced membrane vesicles (CIMVs) are an innovative therapeutic tool, MSCs-derived cytochalasin B membrane vesicles (CIMVs-MSCs) significantly inhibit the activation of helper T cells and cytotoxic T cells. In vitro studies have shown that CIMVs-MSCs cannot induce immune responses in mice, suggesting that CIMVs-MSCs have an immunosuppressive effect (Gomzikova et al., 2020). Intervention with exosomes given to in a mouse model of primary sclerosing cholecystitis (PSC) revealed that exosomes were able to reduce the extent of liver fibrosis and decrease the proliferation of T cells in the liver by inhibiting the NF-κB signaling pathway (Angioni et al., 2020).
3.3.2 Regulation the Differentiation and Phenotypic Transformation of T Lymphocytes
IFN-γ is a member of the type II interferon, which is widely involved in the immune response. BMMSCs were found to promote the phenotypic transformation of T cells. BMMSCs were administered to alum-treated mice and were found to cause Th2-mediated eosinophilic pneumonia. BMMSCs can promote the Th1 phenotype of antigen-specific CD4 +cells and inhibit Th2-mediated allergic airway inflammation through an IFN-γ dependent process (Goodwin et al., 2011). Co-cultured MSCs with CD4+ cells and found MSCs downregulate Th1/Th17 immune responses in a PGE2-dependent manner (Zhou et al., 2020). Tregs can act through cell-to-cell contact, modulate inhibitory cytokines, such as IL-10, and modulate body immunity by interacting with antigen-presenting cells. Accordingly, they play an essential role in maintaining immune homeostasis (Wing et al., 2019). MSCs are able to induce Tregs production (Zhang et al., 2018). Milad Riazifar and his colleagues used the autoimmune encephalomyelitis (EAE) mouse model to assess the therapeutic effects of exosomes secreted by BMMSCs in multiple sclerosis, and found that the number of CD4+, CD25+, and Forkhead box P3 (FOXP3)+ Tregs on the spinal cord of mice upregulate after intravenous injection of IFN-γ (IFN-γ-Exo)-stimulated MSCs-derived exosomes (Riazifar et al., 2019). In conclusion, MSCs intervene in the activation, proliferation, and phenotypic transformation of T cells, as well as to increase the level of Tregs, thus exerting an immunomodulatory effect.
3.4 B Lymphocytes
B cells are derived from stem cells of BM, mainly located in human lymph nodes and spleen. B cells can involve in regulating the immune response, which is related to their ability to produce antibodies. Mature B cells may become activated B cells after being stimulated by antigen, and then differentiate into plasma cells to secrete and synthesize antibodies, to perform humoral immunity (Mauri and Bosma. 2012; Zhang et al., 2012).
3.4.1 Regulation of the Proliferation of B Lymphocytes
MSCs are capable of affecting the activity of B cells by the immunomodulators such as cytokines, chemokines, and growth factors. In one study, it was found that MSCs from human term placental amniotic membrane (hAMSCs) and conditioned medium-derived mesenchymal stem cells (CM-hAMSCs) generated from their culture had an effect on B cells proliferation and differentiation, hAMSCs and CM-hAMSCs both strongly inhibited the proliferation of CpG-activated B cells. In addition, CM-hAMSCs blocked B-cell differentiation, resulting in an increased proportion of mature B cells and reduced formation of antibody-secreting cells (Magatti et al., 2020). It has been shown that hAMSCs have immunosuppressive effects on B cells and constitutively expresses high levels of the immunosuppressive ligand programmed cell death 1 ligand 1 (PD-L1) in response to IFN-γ (Wang et al., 2016b).
3.4.2 Regulation of the Differentiation and Phenotypic Transformation of B Lymphocytes
The immunomodulatory effect of MSCs on B cells reflect in the inhibition of their differentiation and phenotypic transformation. In a mouse model of colitis, intraperitoneal injection of BMMSCs could regulate the immunomodulatory effects of B cells by upregulating IL-10 expression, induce a regulatory B-cell (Breg) population characterized by CD23 and CD43 phenotypic markers, increase the number of CD23+, CD43+, and Breg cells, reduce the clinical and pathological severity of colitis in mice (Chen et al., 2019a). Researchers studied the interactions of BM-MSCs and placental MSCs (P-MSCs) in the mouse model, found that P-MSCs could inhibit the proliferation and further differentiation of B cells (Lee et al., 2021).
The immunomodulatory effects of MSCs on B cells have been studied in many clinical therapies. In a clinical study on chronic graft-versus-host disease, 38 patients treated with MSCs showed a remarkable increase in the number of CD27+ and memory B cells, while plasma B-cell activating factor (BAFF) levels decreased and BAFF-R (BAFF receptor) expression increased on peripheral B cells (Peng et al., 2014).
Although MSCs play a substantial immunosuppressive role for B cells by inhibiting the proliferation of B cells through inflammatory factors such as IFN-γ and TNF-α. Remarkably, the regulatory role of MSCs on B cells is still under investigation, some studies have found that MSCs have a promotive effect on B-cells proliferation, and BMMSCs of B cells chronic lymphocytic leukemia (B-CCL) patient origin were shown to greatly inhibit B cells proliferation and immunoglobulin G (IgG) secretion compared to normal MSCs (Pontikoglou et al., 2013). Co-cultures of MSCs with lymphocytes showed that MSCs stimulate antibody secretion from B cells, and whether antibody secretion from B cells was inhibited or promoted was determined by the dose of MSCs (Rasmusson et al., 2007). However, in some studies, co-culture of LPS-stimulated B cells with MSCs revealed that the proliferation and differentiation of B cells can be inhibited (Asari et al., 2009). Adipogenic-differentiated MSCs (Adi-MSCs) can stimulate B cells proliferation and activation, and promote the secretion of BAFF in the presence of anti-CD3 and anti-Mu-chain treatment (Wang et al., 2011).
3.5 Natural Killer Cells
NK cells, also known as natural killer cells, are critical immune cells produced by the body, and they are involved in hypersensitivity reactions. NK cells have many receptors that receptors tightly regulate the activity of NK cells, enabling them to distinguish between “normal” and “dangerous” cells (Morvan and Lanier. 2016; Hodgins et al., 2019).
3.5.1 By Regulating the Proliferation and Degranulation Effects of Natural Killer Cells
MSCs could be immunomodulated by NK cells. It has shown that BMMSCs have the ability to promote the degranulation effect of NK cells, which can occur when NK cells are near target cells due to components such as perforin and granzyme, and degranulation is associated with the killing activity of NK cells, which directly kill target cells and exert innate immunity (Bradley et al., 2018). NK cells of peripheral blood mononuclear cells originate from healthy donors incubated with SKO-007 (J3) cells (Human myeloma cells), some of the cells were untreated, and some were incubated with BMSC-CM for 72 h, as target cells for degranulation assay. The results showed that CD107a expression was substantially elevated in the combined BMMSC incubation group, indicated that the degranulation of NK cells was enhanced (Mekhloufi et al., 2020). MSCs inhibit NK cells by inhibiting IL-2 too. In a study by Grazia Maria Spaggiari and his colleagues, they explored the result of possible interactions between NK cells and MSCs, and it was found that MSCs not only inhibited the proliferation of NK cells but also prevented the induction of effector functions, and they also observed that MSCs strongly inhibit interleukin-2 (IL-2)-induced NK-cell proliferation (Spaggiari et al., 2008).
3.5.2 Regulation of Activation and Phenotype of Natural Killer Cells
BMMSCs are able to modulate the immune activity of hepatic NK cells. In the Con A mouse model of liver injury, BMMSCs intervention was performed and BMMSCs transplantation was found to reduce cytotoxic substances and activation of r NK cells in the mouse liver (Qingqing et al., 2014). Studies have shown that activation of MSCs between tissues modulate the immune function of NK cells as well, and MSCs could secrete type I interferon to enhance NK cells effector function, while at subsequent time points, TGF-β and IL-6 could limit NK cells effector function and terminate the inflammatory response by inducing a regulatory senescence-like NK cells phenotype (Petri et al., 2017). Additionally, NK cells and MSCs have a bidirectional role in regulating and influencing NK cells activity. Pre-activated MSCs were found to notably inhibit the expression of activation markers in hepatic NK cells after co-transplantation with pancreatic islets to regulate NK cells activity (Ishida et al., 2019). Therefore, MSCs can interfere with the degranulation of NK cells and have an inhibitory effect on the proliferative killing activity of NK cells, which is associated with cytokines such as IL-2 and IFN-γ.
We have described the way MSCs regulate immunity between different cells. We know that endothelial cells, macrophages, neutrophils, lymphocytes, monocytes, etc. accompany different roles in cardiovascular diseases and whether MSCs can further play a therapeutic role by influencing the immune metabolism of these cells. Therefore, we will focus our discussion on the therapeutic role of MSCs in a variety of cardiovascular diseases (Figure 2).
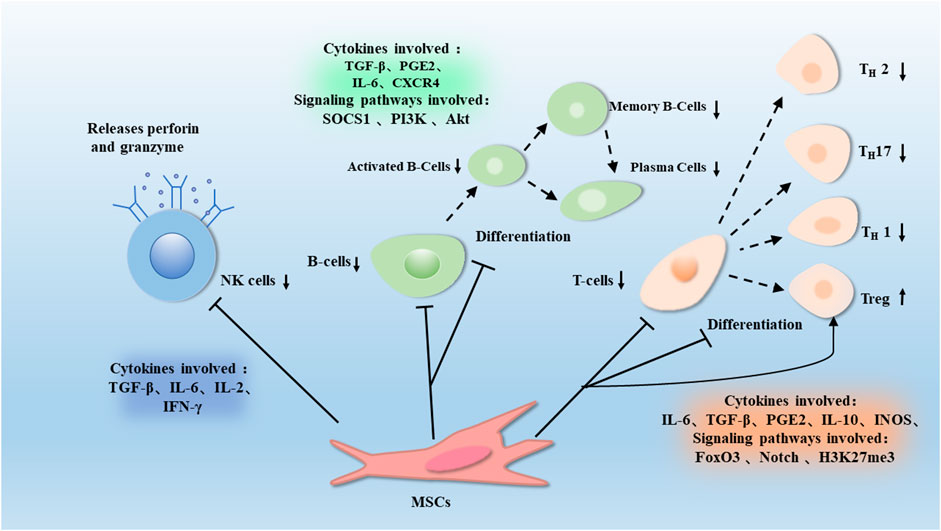
FIGURE 2. T cells can divide into different subpopulations during immune regulation, such as Th1, Th7, Th2, and Tregs. MSCs could inhibit suppression of T cells proliferation and differentiation, and induce Tregs through proteins such as H3K27me3, Notch and FOXP3. This effect is associated with the release of Soluble factors such as TGF-β, IL-10, PGE-2, IL-6, and IDO. MSCs gradually differentiate into B cells, which can differentiate and proliferate into plasma cells. MSCs inhibit the proliferation of B cells and antibody production through proteins such as Socs1, PI3K, and Akt, which are associated with factors such as TGF-β, PGE2, IL-6, and CXCR4. MSCs also promote the release of perforin and granzyme from NK cells, thus promoting the degranulation of NK cells, which is associated with factors such as TGF-β, IL-6, IL-2, and IFN-γ.
4 Immunomodulation of MSCs in Cardiovascular Disease
4.1 Myocardial Infarction
Myocardial infarction (MI) is a disease caused by severe narrowing of the coronary arteries and a dramatic reduction or interruption of the blood supply, which leads to severe acute ischemia in the myocardium and ischemic necrosis. Myocardial ischemia over a long period of time can lead to myocardial cell death, severe angina pectoris, arrhythmias, and other symptoms, which could be life-threatening in severe cases.
MSCs are able to reduce cardiac dysfunction through immunomodulatory effects. The reason why MSCs can treat heart disease may be due to promoting the increase in the number of M2 macrophages. In the mice MI model, MSCs were injected into mice with BM-derived macrophages and found that MSCs could reduce M1 phenotypic markers (IL-6, IL-1β) and increase M2 phenotypic markers (IL-10, IL-4), and facilitated the recovery of cardiac function (Cho et al., 2014). Interleukin-33 (IL-33) is the tissue-derived nuclear cytokine from the IL-1 family, its function is to release alarm signals in case of cell damage or tissue damage. It targets mast cells, Tregs, NK cells, neutrophils, and macrophages in the body, making it a vital important immunomodulatory molecule (Cayrol and Girard. 2018). MSCs transfected with IL-33 were co-cultured with T cells and macrophages, and the proliferation of T cells and polarization of macrophages were observed. The experiments showed that IL33-MSCs decreased T cells proliferation, and enhanced the polarization of M1 phenotype to M2 phenotype, and the myocardial fibrosis, inflammation, and cardiac function recovered better with IL33-MSCs (Chen et al., 2019b).
In addition to IL-33, IL-10 is also an important immunomodulatory factor. IL-10 is a multipotent cytokine with properties that prevents host damage by limiting the immune response of pathogens and exerts immunosuppressive or immunostimulatory effects in various cells (Saraiva and O'Garra. 2010). MSCs are able to perform immunomodulatory functions by regulating IL-10, IL-10-transfected MSCs were injected into MI mice and found that IL-10-transfected MSCs enhanced cell viability and increased IL-10 secretion, the infarct size, myocardial injury and apoptosis were reduced, and systemic and local inflammation were reduced (Meng et al., 2018).
MSCs play a role in tissue repair of cardiovascular diseases. Studies have shown that after MI, MSCs can differentiate into endothelial cells or cardiomyocytes and exert their immunomodulatory effects, such as alleviating tissue damage and promoting tissue repair by inhibiting neutrophil infiltration. Alternatively, tissue repair is achieved by altering the distribution of effective immune cells and recruiting M2 macrophages to the vicinity of damaged tissue. In the murine model of MI, injection of MSCs significantly decreased the M1 phenotype of macrophages, decreased the expression of Interleukin-1β (IL-1β) and IL-6, increased the expression IL-10, and increased the alternating activation of monocytes/macrophages. MSCs may repair the myocardium through IL-10-mediated infiltration of pro-inflammatory macrophages to anti-inflammatory macrophages at the site of infarction (Dayan et al., 2011). MSCs were injected in the porcine model of heart failure combined with MI and were found to exhibit enhanced viability and promote vascular regeneration by activating Tregs and reducing inflammatory cells (Liao et al., 2019).
However, single MSCs therapy tends to have low delivery rates. In the rat model of MI, it was found that combined delivery of MSCs and MSCs-derived exosomes were able to achieve more efficient anti-inflammatory and vascular tissue repair than MSCs therapy by decreasing the expression of inflammatory factors such as IL-6 and TNF-α and increasing the expression of Recombinant Stromal Cell-Derived Factor 1 (SDF-1) (Huang et al., 2019).
MSCs and their derived materials are emerging in the treatment of MI as well. Exosomes of porcine cardiac adipose tissue-derived MSCs (cATMSC) loaded onto a three-dimensional scaffold designed from decellularized heart tissue and injected into pigs after MI, found to reduce macrophage and T cell infiltration in the damaged myocardium and increase vascular density and tissue remodeling (Monguió-Tortajada et al., 2021). Researchers coated the cell membrane of MSCs on PLGA particles loaded with MSCs secretions to create a particle called synMSCs and injected it into mice with acute myocardial infarction (AMI), and found that synMSCs-treated mice were able to express MHC I molecules that allowed them to avoid allogeneic recognition by the immune system, thereby modulating the body’s innate immune response and enhancing their attachment to cardiomyocytes and promoting their survival by secreting adhesion factors (such as SDF1) (Luo et al., 2017). Vineeta Sharma and his colleagues found that 5-Aza loaded protein nanoparticle with MSCs encapsulated hydrogels may support in vitro MSC proliferation, migration and angiogenesis in rat MI model, and the hydrogels could alleviate ventricular remodeling after myocardial infarction by secreting immunoregulatory factors (Sharma et al., 2021). The addition of MSCs -derived exosomes to alginate hydrogel revealed that the delivery of exosomes incorporated in alginate hydrogel (sEVs-Gel) increased residence time in the heart, and promoted the polarization of macrophages from M1 phenotype to M2 phenotype more than exosomes treatment alone, promoted scar tissue repair and vascular regeneration (Lv et al., 2019). Some clinical treatments with MSCs have shown encouraging results. For example, in a clinical study of patients with chronic ischemic heart disease, patients were found to reduce myocardial infarct size, increase LVEF and promote recovery of cardiac function after intravenous administration of MSCs (Kanelidis et al., 2017).
4.2 Heart Failure
Heart failure is a progressive disease, the syndrome that occurs when the cardiovascular diseases progress to a more severe stage. It is usually caused by structural and functional changes in the myocardium due to organic or functional lesions of the heart, which impairs the filling degree and ejection fraction capacity of the ventricles. Furthermore, immune activation and inflammatory responses play an essential role in the course of heart failure.
The therapeutic effect of MSCs on heart failure is mainly based on their ability to regulate the body’s innate and adaptive immunity. Peripheral blood mononuclear cells were isolated from fresh blood samples of patients with end-stage heart failure and co-cultured with MSCs derivatives. The results showed that MSCs had a strong immunosuppressive ability and inhibited lymphocyte proliferation and antibody production in vitro, and plasma cells from patients with end-stage heart failure had high IgG3 production, in vitro experiments showed that MSCs could inhibit IgG3 production, thereby preventing ventricular remodeling after MI and slowing the progression of heart failure (van den Hoogen et al., 2019). CD4 is generally expressed on the surface of human T cells, while Th1 cells in CD4 have a role in humoral immunity (Loo et al., 2018). CD4/CD8 is an important indicator of immune regulation (Overgaard et al., 2015). Co-transplantation of MSCs and pluripotent stem cell-derived cardiomyocytes into mice revealed that MSCs directly affected activated lymphocytes through cell-to-cell contact, thereby decreasing the CD4/CD8 ratio and the proportion of Th1-positive cells among CD4-positive cells and the secretion of various inflammation-related cytokines. Moreover, this pathway could increase the number of cardiomyocytes, enhance myocardial contraction (Yoshida et al., 2020). Denise Philipp and his colleagues studied the effects of bone marrow-derived preconditioned MSCs on hypertrophy-induced pluripotent stem cell-derived cardiomyocytes (iPS-CM), and found that MSCs could participate in immune regulation by secreting cytokines such as IFN-γ and IL-1β, and trigger regression of hypertrophy in iPS-CM in a VEGF-dependent manner, so MSCs therapy may inhibit cardiac hypertrophy (Philipp et al., 2021).
Some clinical studies have shown that MSCs is a new target for the treatment of heart failure. After MSCs injected into patients with chronic heart failure, a momentous decrease in CD4-positive and NK cells was found, a large improvement in left ventricular end systolic volume (LVESV) and LVEF was observed. In this study, MSC therapy was safe, and caused immunomodulatory effects (Butler et al., 2017). Intravenous infusion of UC MSCs in patients with chronic heart failure revealed that UC MSCs could participate in the regulation of adaptive immunity and myocardial remodeling in the body by secreting hepatocyte growth factor (HGF), inhibit T-cell proliferation, and reduce the proliferation of Th 1, Th 2, and cytotoxic T cells (Bartolucci et al., 2017).
MSCs derived materials are also a new target for the treatment of heart failure in recent years. Human amnion-derived stem cells (hAM-MSCs) dressing is a novel MSC-derived material with good potential for clinical application. After administration of hAM-MSCs dressing to mice with ischemic cardiomyopathy, it was found that the dressing could improve myocardial remodeling by promoting the secondary release of paracrine factors from endogenous cells and promoting the polarization of the M1 phenotype to the M2 phenotype of macrophages. So, hAM-MSCs dressing can slow down the development of heart failure (Fields et al., 2021). Pura-Matrix hydrogel with MSCs applied to mouse epicardium after MI, found that it could inhibit the development of inflammation, promote the formation of myocardial capillary, reduce interstitial fibrosis, improve cardiac function and alleviate the progression of heart failure by secreting cytokines such as IL-10 (Ichihara et al., 2018). Researchers seeded MSCs and macrophages into a polycaprolactone scaffold and filled it with MSCs-derived exosomes, found that this novel MSCs-derived material increased the wound healing properties and type I collagen of MSCs and improved the M2 phenotype of macrophages, thus this exosome-rich bio scaffold is an effective strategy to restore contractility of myocardial scars (Chachques et al., 2021). Both MSCs and their derived materials can play a role in the treatment of heart failure by secreting immune factors and regulating immune cells (Figure 3).
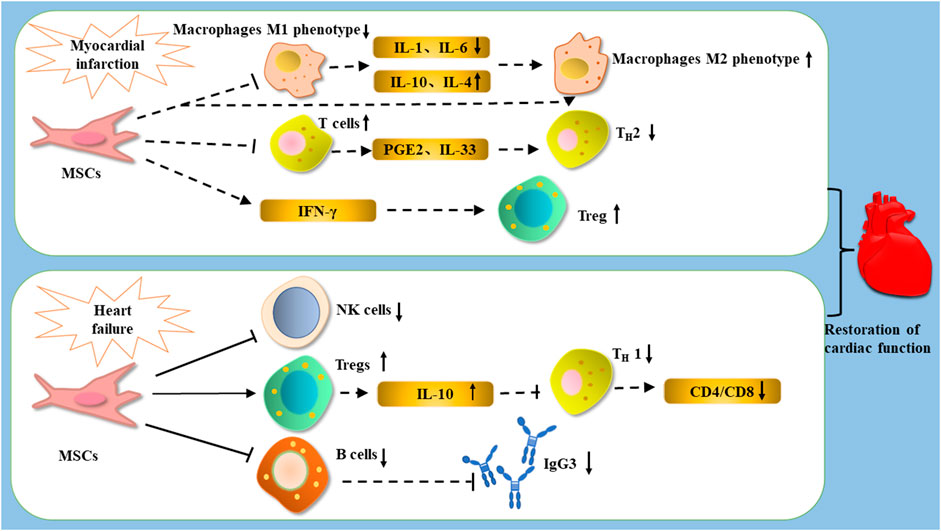
FIGURE 3. After the occurrence of MI, treated with MSCs, MSCs improve cardiac function by decreasing the expression of IL-1β and IL-6, increasing the expression of IL-10 and IL-4, and promoting the polarization of macrophages from M1 phenotype to M2 phenotype; they can improve cardiac function by promoting the expression of Tregs to enhance cardiac function, as well as inhibiting T cells and influencing the differentiation of Th1 cells through PGE2 and IL-33. After the occurrence of heart failure, treatment with MSCs can restore cardiac function by reducing the number of NK cells, inhibiting the proliferation of B cells and the production of the antibody IGg3, as well as increasing the number of Tregs and reducing the CD4/CD8 ratio by reducing Th1 cells through IL-10.
4.3 Atherosclerosis
Atherosclerosis is a chronic vascular inflammatory disease. That is the cause of coronary heart disease. Atherosclerotic lesions are associated with macrophages, T cells and other immune response cells (Hansson and Hermansson. 2011).
Because of their immunomodulatory and tissue regenerative capabilities, MSCs are well-positioned to treat atherosclerosis, And the therapeutic effect of MSCs on atherosclerosis is mainly achieved by regulating macrophages. As we all known, abnormal lipid metabolism can lead to endothelial dysfunction and the secretion of adhesion factors, leading to the recruitment of macrophages to form foam cells, promoting atherosclerotic plaque formation. Macrophages secrete anti-inflammatory factors, such as IL-10, TGF-β, and promote blood vessel regeneration and tissue remodeling and repair (Adutler-Lieber et al., 2013). Therefore, the treatment of atherosclerosis with MSCs is mainly completed by regulating macrophages, and some immune factors also play a role in this process. For example, IL-6 is produced in response to infection and tissue injury and contributes to host defense by stimulating the acute phase response, hematopoiesis, and immune response. Persistent dysregulation of IL-6 synthesis plays a pathological role in chronic inflammation and autoimmunity (Tanaka et al., 2014). Human-induced pluripotent stem cells (iPSC-MSCs) were intravenously administered to ApoE −/−mice on an HFD for 12 weeks, and iPSC-MSCs were found to notably reduce plaque size and make the macrophages in the plaques lower than those in the controls. Furthermore, iPSC-MSCs reduced inflammation by reducing serum levels of inflammatory cytokines, such as TNF-α and IL-6, thereby treating atherosclerosis (Shi et al., 2018b). Skin is an ideal source of MSCs supply, and in the ApoE −/− mice model, skin-derived MSCs(S-MSCs) were found to migrate into atherosclerotic plaques and selectively adhere in the vicinity of macrophages. Furthermore, it reduced the release of the pro-inflammatory cytokine TNF-α and increased the expression of anti-inflammatory factor IL-10 in atherosclerotic plaques, resulted in the regulation of macrophages function and inhibition of atheromatous plaque formation (Li et al., 2015b). MSCs-derived exosomes are considered a new target for the treatment of atherosclerosis. MSCs-derived exosomes injected into ApoE−/− mice and MSCs-derived exosomes were found to promote polarization of the macrophages M2 phenotype and reduce plaque size and macrophages infiltration via miRNA-21a-5p (Ma et al., 2021).
In addition to macrophages, Treg and T cells are also able to play a role in the treatment of MSC. TGF-β1 could produce an immune response when it is activated, and Tregs can suppress proximal immune cells by activating Glycoprotein -A repetitions predominant (GARP) -mediated activation of potential TGF-β1 acting on their surface with integrin αVβ8. Treatment of atherosclerotic mice with MSCs revealed increased secretion of anti-inflammatory cytokines such as TGF-β1 and IL-10 and TGF-β1 could be involved in MSC-mediated changes in the number of CD4+CD25+ forkhead box P3 (FOXP3) +Tregs and NK cells, and reduce their proliferation, this resulted in the regulation of host adaptive immunity (Li et al., 2017; Liénart et al., 2018). Mice were injected with MSCs after low-density lipoprotein (LDL) knockout and fed a high-fat diet (HFD), which induced atherosclerosis, it was found that the number of T cells reduced after MSCs treatment, the serum CCL2 level decreased, the lesion in the aortic root reduced, the number of macrophages at the lesion site decreased, and serum cholesterol reduced. It was shown that treatment with MSCs greatly reduced dyslipidemia and treated atherosclerosis in mice (Wang et al., 2015).
MSCs are capable of exerting immunomodulatory and immunosuppressive effects through some signaling pathways. Culture media for ADMSCs, like MSCs, can reduce the expression of cell adhesion factors by inhibiting the mitogen-activated protein kinase (MAPK) and NF-κB pathways and reduce the accumulation of macrophages in the vessel wall by inhibiting MAPK and NF-κB pathways. The κB pathway decreases LPS-induced M1 marker expression and increases M2 marker expression through activation of the STAT3 signaling pathway (Takafuji et al., 2019) (Figure 4).
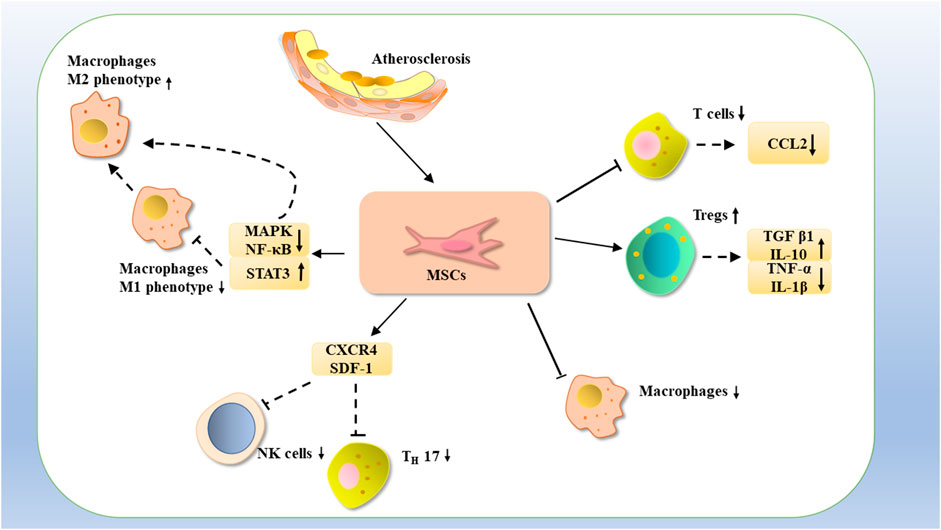
FIGURE 4. MSCs may treat atherosclerosis by inhibiting the proliferation of T cells with CCL2 expression, or by increasing the number of Tregs, increasing the expression of TGF-β1 and IL-10, decreasing the expression of TNF-α, and IL-1β, as well as by reducing the number of macrophages. Atherosclerosis could be treated by inhibiting NK cells with TH17 through CXCR4 and SDF-1 and downregulating MAPK and NF-κB and upregulating STAT3 expression, thereby inhibiting NK cells M1-type macrophages and promoting the phenotypic transformation of M1-type macrophages to M2-type macrophages.
4.4 Myocardial Ischemia Reperfusion Injury
After MI, timely reperfusion treatment can reduce the infarct area, but symptoms such as arrhythmia and myocardial contractile dysfunction often occur after reperfusion therapy, thus leading to myocardial ischemia-reperfusion injury (MIRI) (Cohn et al., 2000). Infiltration of inflammatory cells occurs after myocardial ischemia/reperfusion, allowing the progression of MIRI toward inflammatory injury.
Toll-like receptor 2 (TLR2), the most widely expressed member of the TLR family, is the type I transmembrane protein that acts as a pattern recognition receptor, recognizes and binds to several pathogen-associated molecular patterns, and triggers a cascade of signaling that leads to the release of mediators of inflammation, thereby initiating an innate immune response. TLR2 is associated with inflammation and myocardial dysfunction after MIRI, knockdown of MSCs-derived exosomes of TLR2 could improve myocardial recovery from immunomodulation and promote the release of vascular growth factors, thereby protecting cardiac function (Ma et al., 2018). Neutrophils play an important role in the development of MIRI. MSCs were injected in MIRI model rats and were found to be able to initiate an immune response and improve cardiac function in rats after MIRI by enhancing M2 macrophages-induced efferocytosis of apoptotic neutrophils (Zhang et al., 2020a). CD73 can convert Adenosine monophosphate (AMP) into adenosine, thereby inhibiting T-cell activation. In the rat model of MIRI, MSCs were injected into the damaged myocardium and found to mediate the activity of CD73 and attenuate the infiltration of innate immune cells, thereby protecting cardiac function (Shin et al., 2018).
MSCs injection into mice with AMI revealed that MSCs-derived exosomes had profound immunomodulatory effects on DCs and monocytes or macrophages, and exosomes from highly expressed miRNA-181 MSCs created an anti-inflammatory environment, enhanced Tregs polarization, and significantly improved ischemia-reperfusion through downstream c- Fos proteins cardiac function and infarct size in mice with impaired perfusion (Wei et al., 2019). MSCs-derived exosomes could promote the conversion of macrophages from M1 to M2 phenotype by secreting miRNA 182-a and downregulating TLR4, thereby reducing the infarct size and decreasing the level of inflammation in the ischemic myocardium of mice with MIRI (Zhao et al., 2019). The MSCs-derived exosomes were modified with monocyte mimics to form monocyte mimic-bioinspired MSC-EVs (Mon-Exos), which found to have better targeting to the damaged myocardium. In a rat MIRI model, it promoted vascular endothelial repair and modulated macrophage subsets, thus resulted a significant improvement in cardiac function (Zhang et al., 2020b).
Heart transplantation cause MIRI due to procurement and long transplantation times, and although MSCs are cardioprotective, there are still many limitations to this treatment. To address the limitation that MSCs are difficult to transport and store, the researchers treated mice hearts with ischemic cryopreservation followed by the use of a preservation solution, It was found that MSCs-CMs or MSCs-EVs in the preservation solution reversed the adverse effects of long-term frozen ischemia on the donor heart and achieved protection of the donor heart by inhibiting the secretion of pro-inflammatory factors such as IL-1β and IL-6 (Wang et al., 2020). Therefore, both MSCs and MSCs-derived exosomes can inhibit MIRI by exerting their immunoregulatory functions.
4.5 Cardiomyopathy and Myocarditis
Dilated cardiomyopathy is defined by dilatation and systolic dysfunction of the left ventricle in the absence of severe coronary artery diseases or abnormal loading conditions. And it is one of the causes of heart failure (Japp et al., 2016). The expression of some genes is extremely important for the immunomodulatory process of MSCs, and MSCs are able to modulate the expression of some cytokines by regulating the expression of genes, thus enabling MSCs to play a role in the treatment of diabetic cardiomyopathy. In the rat model of dilated cardiomyopathy, double overexpression of miR-19a and miR-20a in human-induced iPSC -MSCs was able to suppress the inflammatory response and promote the recovery of cardiac function in rats with dilated cardiomyopathy by inhibiting the secretion of TNF-α/IL-1ß (Sheu et al., 2021). MSCs were injected intravenously into rats with diabetic cardiomyopathy and found to secrete cytokine PGE2 through paracrine action, thereby immunomodulating diabetic cardiomyopathy rats, reducing myocardial fibrosis and alleviating cardiac function (Jin et al., 2020). MSCs-derived exosomes were able to improve the myocardial inflammatory microenvironment in mice with dilated cardiomyopathy by significantly reducing M1 macrophages in the blood and heart and promoting the conversion of macrophages from the M1 phenotype to the M2 phenotype, promoting the recovery of cardiac function (Sun et al., 2018).
Chronic chagas disease cardiomyopathy, a highly fatal inflammatory cardiomyopathy associated with the patient’s innate immune response, mainly characterized by high expression of the pro-inflammatory cytokine Th1 T cells, hypertrophy of cardiomyocytes and prominent fibrosis in the cardiac lesion region (Cunha-Neto and Chevillard. 2014). MSCs may treat Chronic chagas disease cardiomyopathy by regulating the secretion of inflammatory factors. The study showed that MSCs caused inhibition of cardiac inflammation and fibrosis and reduced the expression levels of TNF α, IL-1β, IL-6, and IFN-γ in the mice model of chronic chagas disease cardiomyopathy, and indicated that MSCs had rich potential to treat chronic Chagas disease cardiomyopathy (Souza et al., 2017). MSCs that overexpress granulocyte colony-stimulating factor (MSC G-CSF) could modulate the adaptive immunity of the body by regulating Tregs, leading to the treatment of inflammatory cardiomyopathy caused by chronic Chagas disease (Silva et al., 2018).
Myocarditis is an inflammatory disease of the myocardium associated with immune dysfunction that causes cardiogenic shock and death (Cooper. 2009). Researchers found that FM-MSCs-treated mice with experimental immune myocarditis had significantly fewer infiltrating Th17 cells and a significantly lower proportion of Th1 cells. It was found that FM-MSCs could improve the body’s immunity by suppressing Th1/Th17, thereby treating experimental immune myocarditis (Ohshima et al., 2012). In addition, MSCs could reduce the severity of experimental immune myocarditis by releasing HGF and inhibiting the expression of IL-2, IL-6 in the myocardium (Okada et al., 2007). Injected MSCs overexpressing IL-10 in a mouse model of autoimmune myocarditis and found that MSCs were able to reduce the inflammatory level of the heart and the degree of myocardial fibrosis by delivering IL-10, thereby preventing and treating overactivated immune system from attacking cardiomyocytes (Shao et al., 2020). Coxsackievirus B3(CVB3) can induce myocarditis. Co-cultured of MSCs with CVB3-infected HL-1 cardiomyocytes revealed that MSCs reduced the CVB3-induced CD4+ and CD8+ T cells activation in a nitric oxide (NO)-dependent way and required IFN-γ priming (Van Linthout et al., 2011) (Figure 5; Table 2).
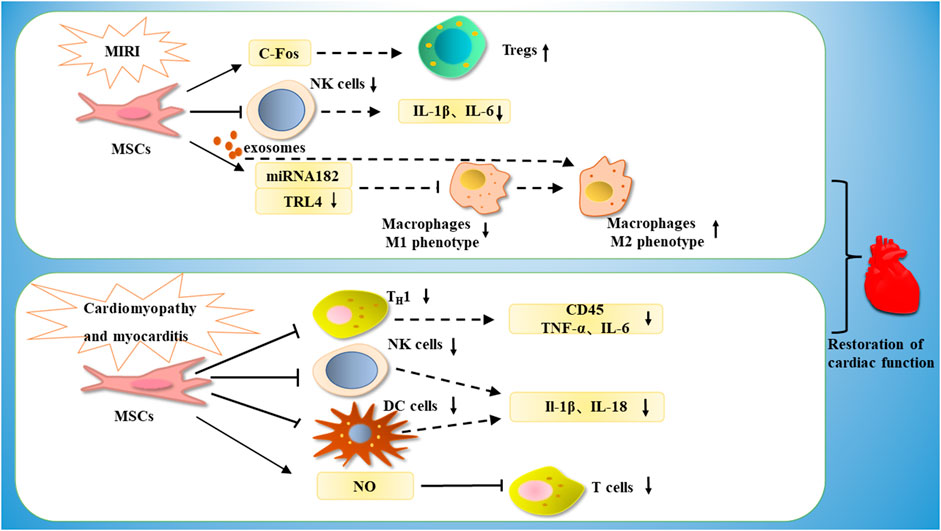
FIGURE 5. MSCs are able to treat cardiomyopathy by increasing the number of Tregs through c-Fos, or by inhibiting NK cells, decreasing the expression of IL-1β and IL-6, or by releasing miRNA182-a through exosomes, thereby downregulating TRL4, inhibiting M1-type macrophages and promoting phenotypic conversion of M1-type macrophages to M2-type macrophages. In the treatment of myocarditis, MSCs are able to exert immunomodulatory effects by downregulating Th1, thereby downregulating CD45, TNF-α, IL-6, or by inhibiting NK cells and DC cells, thereby downregulating IL-1β and IL-18 expression, or by inhibiting T cell proliferation through NO.
5 Conclusion
MSCs are multipotent stem cells with self-renewal capacity and multidirectional differentiation. They represent the most promising direction for the treatment of diseases such as inflammatory, neurological, cardiovascular, and autoimmune diseases because of their multilineage differentiation potential, immunomodulatory properties, and pro-angiogenic properties (Harrell et al., 2019b). Moreover, its secretory products, such as exosomes, play an essential role in multilineage differentiation function. MSCs have potent immunomodulatory and anti-inflammatory activities, which are mediated by paracrine function and contribute to tissue repair (Zhang et al., 2019). Therefore, MSCs have remarkable efficacy in the treatment of cardiovascular diseases. For example, MSCs mediate cardiomyocytes immune responses through STAT3 to improve cardiac function, regulate c-Fos protein by secreting exosomes and enhance Tregs polarization to improve cardiac function in ischemia-reperfusion injured mice, reduce inflammatory response in the MI area by increasing IL-10 levels, and treat atherosclerosis by regulating the phenotypic transition between macrophage inflammation and phenotype. MSCs have a wide potential for clinical application, which may be related to the alternation of cytokines/cell lineages profiles by lived MSCs surrounding the damaged heart, and both autologous hAM-MSCs and autologous BMMSCs transplantation have also shown good myocardial protection. The immunomodulation of MSCs has a vital role in the repair of myocardial tissue, in addition, the regulation of innate and acquired immunity by MSCs and their derived materials has a place in the treatment of cardiovascular diseases.
In experimental and clinical treatments, MSCs have been studied for immunomodulation mostly by in vitro culture and intravenous infusion (currently, there are also clinical cases of nebulized inhalation of MSCs to treat COVID-19, and immunomodulation by MSCs is also a new direction for future research on COVID-19) (Yen et al., 2020; Hong and Hashimoto. 2021). Different MSCs preparation methods also have an impact on the immunomodulatory effects of MSCs. The preparation methods of MSCs include flat plate preparation method, stirred bioreactor preparation method (Eibes et al., 2010), perfusion bioreactor preparation method, etc. (Cho et al., 2008). However, the stirred bioreactor preparation method may lead to cells destruction and thus affect the immunomodulatory activity of MSCs. MSCs exert immune regulation mostly through direct cell-to-cell contact, for example, promoting phenotypic transformation of macrophages through direct cell-to-cell contact (Terai et al., 2021) or by releasing cytokines through paracrine effect, such as secretion, IDO, TGF-β, and PGE2 (Di Trapani et al., 2016). It can function as an immunomodulatory agent by secreting exosomes, such as the inhibition of IL-6 and TNF-α secretion through exosomes, thereby regulating the inflammatory microenvironment (Lu et al., 2021). Nevertheless, MSCs still have many immunomodulatory potentials and require our findings.
According to the current study, there are still some limitations in the clinical application of MSCs. For example, unexpected differentiation, low in vivo survival rate of transplanted, and calcification of the infarcted area affect the long-term outcome of MI. The storage and transportation of MSCs is also the reason for the limited clinical application. Studies have shown that the cryopreservation of MSCs can lead to the damage of their immunoregulatory characteristics (Namba. 2019). Therefore, although the immunomodulatory function of MSCs may be a breakthrough and promising therapeutic approach for the treatment of cardiovascular diseases, it is still in a validated state, and further basic research and clinical data are needed to ensure the feasibility of MSCs in the treatment of cardiovascular diseases.
Author Contributions
YW and ZQ analyzed the current literature and wrote the manuscript. ZY, NJ, and XY designed and created the figure. DG, LH, and HL edited the article. JZ and ML designed the manuscript. All authors read and approved the final version of the article.
Funding
We thank the financial support from National Natural Science Foundation of China (Grant No. 81804046).
Conflict of Interest
The authors declare that the research was conducted in the absence of any commercial or financial relationships that could be construed as a potential conflict of interest.
Publisher’s Note
All claims expressed in this article are solely those of the authors and do not necessarily represent those of their affiliated organizations, or those of the publisher, the editors and the reviewers. Any product that may be evaluated in this article, or claim that may be made by its manufacturer, is not guaranteed or endorsed by the publisher.
Acknowledgments
We thank all the members of the Zhang laboratory for their help and constructive discussions.
References
Adutler-Lieber, S., Ben-Mordechai, T., Naftali-Shani, N., Asher, E., Loberman, D., Raanani, E., et al. (2013). Human Macrophage Regulation via Interaction with Cardiac Adipose Tissue-Derived Mesenchymal Stromal Cells. J. Cardiovasc. Pharmacol. Ther. 18, 78–86. doi:10.1177/1074248412453875
Albany, C. J., Trevelin, S. C., Giganti, G., Lombardi, G., and Scottà, C. (2019). Getting to the Heart of the Matter: The Role of Regulatory T-Cells (Tregs) in Cardiovascular Disease (CVD) and Atherosclerosis. Front. Immunol. 10, 2795. doi:10.3389/fimmu.2019.02795
Angioni, R., Calì, B., Vigneswara, V., Crescenzi, M., Merino, A., Sánchez-Rodríguez, R., et al. (2020). Administration of Human MSC-Derived Extracellular Vesicles for the Treatment of Primary Sclerosing Cholangitis: Preclinical Data in MDR2 Knockout Mice. Ijms 21, 8874. doi:10.3390/ijms21228874
Asari, S., Itakura, S., Ferreri, K., Liu, C.-P., Kuroda, Y., Kandeel, F., et al. (2009). Mesenchymal Stem Cells Suppress B-Cell Terminal Differentiation. Exp. Hematol. 37, 604–615. doi:10.1016/j.exphem.2009.01.005
Bartolucci, J., Verdugo, F. J., González, P. L., Larrea, R. E., Abarzua, E., Goset, C., et al. (2017). Safety and Efficacy of the Intravenous Infusion of Umbilical Cord Mesenchymal Stem Cells in Patients with Heart Failure. Circ. Res. 121, 1192–1204. doi:10.1161/circresaha.117.310712
Bradley, T., Peppa, D., Pedroza-Pacheco, I., Li, D., Cain, D. W., Henao, R., et al. (2018). RAB11FIP5 Expression and Altered Natural Killer Cell Function Are Associated with Induction of HIV Broadly Neutralizing Antibody Responses. Cell 175, 387–399. doi:10.1016/j.cell.2018.08.064
Briseño, C. G., Murphy, T. L., and Murphy, K. M. (2014). Complementary Diversification of Dendritic Cells and Innate Lymphoid Cells. Curr. Opin. Immunol. 29, 69–78. doi:10.1016/j.coi.2014.04.006
Butler, J., Epstein, S. E., Greene, S. J., Quyyumi, A. A., Sikora, S., Kim, R. J., et al. (2017). Intravenous Allogeneic Mesenchymal Stem Cells for Nonischemic Cardiomyopathy. Circ. Res. 120, 332–340. doi:10.1161/circresaha.116.309717
Cayrol, C., and Girard, J.-P. (2018). Interleukin-33 (IL-33): A Nuclear Cytokine from the IL-1 Family. Immunol. Rev. 281, 154–168. doi:10.1111/imr.12619
Chachques, J. C., Gardin, C., Lila, N., Ferroni, L., Migonney, V., Falentin-Daudre, C., et al. (2021). Elastomeric Cardiowrap Scaffolds Functionalized with Mesenchymal Stem Cells-Derived Exosomes Induce a Positive Modulation in the Inflammatory and Wound Healing Response of Mesenchymal Stem Cell and Macrophage. Biomedicines 9, 824. doi:10.3390/biomedicines9070824
Chen, X., Cai, C., Xu, D., Liu, Q., Zheng, S., Liu, L., et al. (2019a). Human Mesenchymal Stem Cell-Treated Regulatory CD23+CD43+ B Cells Alleviate Intestinal Inflammation. Theranostics 9, 4633–4647. doi:10.7150/thno.32260
Chen, Y., Zuo, J., Chen, W., Yang, Z., Zhang, Y., Hua, F., et al. (2019b). The Enhanced Effect and Underlying Mechanisms of Mesenchymal Stem Cells with IL-33 Overexpression on Myocardial Infarction. Stem Cel Res Ther 10, 295. doi:10.1186/s13287-019-1392-9
Cho, C. H., Eliason, J. F., and Matthew, H. W. T. (2008). Application of Porous Glycosaminoglycan-Based Scaffolds for Expansion of Human Cord Blood Stem Cells in Perfusion Culture. J. Biomed. Mater. Res. 86A, 98–107. doi:10.1002/jbm.a.31614
Cho, D.-I., Kim, M. R., Jeong, H.-y., Jeong, H. C., Jeong, M. H., Yoon, S. H., et al. (2014). Mesenchymal Stem Cells Reciprocally Regulate the M1/M2 Balance in Mouse Bone Marrow-Derived Macrophages. Exp. Mol. Med. 46, e70. doi:10.1038/emm.2013.135
Clark, G. J., Silveira, P. A., Hogarth, P. M., and Hart, D. N. J. (2019). The Cell Surface Phenotype of Human Dendritic Cells. Semin. Cel Develop. Biol. 86, 3–14. doi:10.1016/j.semcdb.2018.02.013
Cohn, J. N., Ferrari, R., and Sharpe, N. (2000). Cardiac Remodeling-Concepts and Clinical Implications: a Consensus Paper from an International Forum on Cardiac Remodeling. J. Am. Coll. Cardiol. 35, 569–582. doi:10.1016/s0735-1097(99)00630-0
Consentius, C., Akyüz, L., Schmidt‐Lucke, J. A., Tschöpe, C., Pinzur, L., Ofir, R., et al. (2015). Mesenchymal Stromal Cells Prevent Allostimulation In Vivo and Control Checkpoints of Th1 Priming: Migration of Human DC to Lymph Nodes and NK Cell Activation. Stem Cells 33, 3087–3099. doi:10.1002/stem.2104
Cui, R., Rekasi, H., Hepner-Schefczyk, M., Fessmann, K., Petri, R. M., Bruderek, K., et al. (2016). Human Mesenchymal Stromal/stem Cells Acquire Immunostimulatory Capacity upon Cross-Talk with Natural Killer Cells and Might Improve the NK Cell Function of Immunocompromised Patients. Stem Cel Res Ther 7, 88. doi:10.1186/s13287-016-0353-9
Cunha-Neto, E., and Chevillard, C. (2014). Chagas Disease Cardiomyopathy: Immunopathology and Genetics. Mediators Inflamm. 2014, 1–11. doi:10.1155/2014/683230
Daneshmandi, S., Karimi, M. H., and Pourfathollah, A. A. (2017). TGF-β1 Transduced Mesenchymal Stem Cells Have Profound Modulatory Effects on DCs and T Cells. Iran J. Immunol. 14, 13–23.
Dayan, V., Yannarelli, G., Billia, F., Filomeno, P., Wang, X.-H., Davies, J. E., et al. (2011). Mesenchymal Stromal Cells Mediate a Switch to Alternatively Activated Monocytes/macrophages after Acute Myocardial Infarction. Basic Res. Cardiol. 106, 1299–1310. doi:10.1007/s00395-011-0221-9
Di Trapani, M., Bassi, G., Midolo, M., Gatti, A., Takam Kamga, P., Cassaro, A., et al. (2016). Differential and Transferable Modulatory Effects of Mesenchymal Stromal Cell-Derived Extracellular Vesicles on T, B and NK Cell Functions. Sci. Rep. 6, 24120. doi:10.1038/srep24120
Ding, D.-C., Shyu, W.-C., and Lin, S.-Z. (2011). Mesenchymal Stem Cells. Cel Transpl. 20, 5–14. doi:10.3727/096368910x
Dong, B., Wang, C., Zhang, J., Zhang, J., Gu, Y., Guo, X., et al. (2021). Exosomes from Human Umbilical Cord Mesenchymal Stem Cells Attenuate the Inflammation of Severe Steroid-Resistant Asthma by Reshaping Macrophage Polarization. Stem Cel Res Ther 12, 204. doi:10.1186/s13287-021-02244-6
Eibes, G., dos Santos, F., Andrade, P. Z., Boura, J. S., Abecasis, M. M. A., da Silva, C. L., et al. (2010). Maximizing the Ex Vivo Expansion of Human Mesenchymal Stem Cells Using a Microcarrier-Based Stirred Culture System. J. Biotechnol. 146, 194–197. doi:10.1016/j.jbiotec.2010.02.015
Feng, Y., Xu, Q., Yang, Y., Shi, W., Meng, W., Zhang, H., et al. (2019). The Therapeutic Effects of Bone Marrow-Derived Mesenchymal Stromal Cells in the Acute Lung Injury Induced by Sulfur Mustard. Stem Cel Res Ther 10, 90. doi:10.1186/s13287-019-1189-x
Fields, L., Ito, T., Kobayashi, K., Ichihara, Y., Podaru, M.-N., Hussain, M., et al. (2021). Epicardial Placement of Human MSC-Loaded Fibrin Sealant Films for Heart Failure: Preclinical Efficacy and Mechanistic Data. Mol. Ther. 29, 2554–2570. doi:10.1016/j.ymthe.2021.04.018
Ghosh, T., Barik, S., Bhuniya, A., Dhar, J., Dasgupta, S., Ghosh, S., et al. (2016). Tumor-associated Mesenchymal Stem Cells Inhibit Naïve T Cell Expansion by Blocking Cysteine export from Dendritic Cells. Int. J. Cancer 139, 2068–2081. doi:10.1002/ijc.30265
Giri, J., Das, R., Nylen, E., Chinnadurai, R., and Galipeau, J. (2020). CCL2 and CXCL12 Derived from Mesenchymal Stromal Cells Cooperatively Polarize IL-10+ Tissue Macrophages to Mitigate Gut Injury. Cel Rep. 30, 1923–1934. doi:10.1016/j.celrep.2020.01.047
Gomzikova, M., Kletukhina, S., Kurbangaleeva, S., Neustroeva, O., Vasileva, O., Garanina, E., et al. (2020). Mesenchymal Stem Cell Derived Biocompatible Membrane Vesicles Demonstrate Immunomodulatory Activity Inhibiting Activation and Proliferation of Human Mononuclear Cells. Pharmaceutics 12, 577. doi:10.3390/pharmaceutics12060577
Goodwin, M., Sueblinvong, V., Eisenhauer, P., Ziats, N. P., LeClair, L., Poynter, M. E., et al. (2011). Bone Marrow‐Derived Mesenchymal Stromal Cells Inhibit Th2‐Mediated Allergic Airways Inflammation in Mice. Stem Cells 29, 1137–1148. doi:10.1002/stem.656
Gupte, K. S., Vanikar, A. V., Trivedi, H. L., Patel, C. N., and Patel, J. V. (2017). In-vitro Generation of Interleukin-10 Secreting B-Regulatory Cells from Donor Adipose Tissue Derived Mesenchymal Stem Cells and Recipient Peripheral Blood Mononuclear Cells for Potential Cell Therapy. Biomed. J. 40, 49–54. doi:10.1016/j.bj.2017.01.003
Hansson, G. K., and Hermansson, A. (2011). The Immune System in Atherosclerosis. Nat. Immunol. 12, 204–212. doi:10.1038/ni.2001
Harrell, C., Fellabaum, C., Jovicic, N., Djonov, V., Arsenijevic, N., and Volarevic, V. (2019b). Molecular Mechanisms Responsible for Therapeutic Potential of Mesenchymal Stem Cell-Derived Secretome. Cells 8, 467. doi:10.3390/cells8050467
Harrell, C. R., Jovicic, N., Djonov, V., Arsenijevic, N., and Volarevic, V. (2019a). Mesenchymal Stem Cell-Derived Exosomes and Other Extracellular Vesicles as New Remedies in the Therapy of Inflammatory Diseases. Cells 8, 1605. doi:10.3390/cells8121605
He, H., Liu, X., Peng, L., Gao, Z., Ye, Y., Su, Y., et al. (2013). Promotion of Hepatic Differentiation of Bone Marrow Mesenchymal Stem Cells on Decellularized Cell-Deposited Extracellular Matrix. Biomed. Res. Int. 2013, 1–11. doi:10.1155/2013/406871
Hodgins, J. J., Khan, S. T., Park, M. M., Auer, R. C., and Ardolino, M. (2019). Killers 2.0: NK Cell Therapies at the Forefront of Cancer Control. J. Clin. Invest. 129, 3499–3510. doi:10.1172/jci129338
Hong, Y., and Hashimoto, M. (2021). I Will Get Myself Vaccinated for Others: The Interplay of Message Frame, Reference Point, and Perceived Risk on Intention for COVID-19 Vaccine. Health Commun., 1–11. doi:10.1080/10410236.2021.1978668
Huang, P., Wang, L., Li, Q., Xu, J., Xu, J., Xiong, Y., et al. (2019). Combinatorial Treatment of Acute Myocardial Infarction Using Stem Cells and Their Derived Exosomes Resulted in Improved Heart Performance. Stem Cel Res Ther 10, 300. doi:10.1186/s13287-019-1353-3
Ichihara, Y., Kaneko, M., Yamahara, K., Koulouroudias, M., Sato, N., Uppal, R., et al. (2018). Self-assembling Peptide Hydrogel Enables Instant Epicardial Coating of the Heart with Mesenchymal Stromal Cells for the Treatment of Heart Failure. Biomaterials 154, 12–23. doi:10.1016/j.biomaterials.2017.10.050
Ishida, N., Ishiyama, K., Saeki, Y., Tanaka, Y., and Ohdan, H. (2019). Cotransplantation of Preactivated Mesenchymal Stem Cells Improves Intraportal Engraftment of Islets by Inhibiting Liver Natural Killer Cells in Mice. Am. J. Transpl. 19, 2732–2745. doi:10.1111/ajt.15347
J, M. P., and Debabrata, B. (2017). Activation and Differentiation of Mesenchymal Stem Cells. J Methods Mol. Biol. (Clifton, N.J.) 1554.
Japp, A. G., Gulati, A., Cook, S. A., Cowie, M. R., and Prasad, S. K. (2016). The Diagnosis and Evaluation of Dilated Cardiomyopathy. J. Am. Coll. Cardiol. 67, 2996–3010. doi:10.1016/j.jacc.2016.03.590
Jin, L., Deng, Z., Zhang, J., Yang, C., Liu, J., Han, W., et al. (2019). Mesenchymal Stem Cells Promote Type 2 Macrophage Polarization to Ameliorate the Myocardial Injury Caused by Diabetic Cardiomyopathy. J. Transl Med. 17, 251. doi:10.1186/s12967-019-1999-8
Jin, L., Zhang, J., Deng, Z., Liu, J., Han, W., Chen, G., et al. (2020). Mesenchymal Stem Cells Ameliorate Myocardial Fibrosis in Diabetic Cardiomyopathy via the Secretion of Prostaglandin E2. Stem Cel Res Ther 11, 122. doi:10.1186/s13287-020-01633-7
Jo, H., Eom, Y. W., Kim, H.-S., Park, H. J., Kim, H. M., and Cho, M.-Y. (2018). Regulatory Dendritic Cells Induced by Mesenchymal Stem Cells Ameliorate Dextran Sodium Sulfate-Induced Chronic Colitis in Mice. Gut and Liver 12, 664–673. doi:10.5009/gnl18072
Kanelidis, A. J., Premer, C., Lopez, J., Balkan, W., and Hare, J. M. (2017). Route of Delivery Modulates the Efficacy of Mesenchymal Stem Cell Therapy for Myocardial Infarction. Circ. Res. 120, 1139–1150. doi:10.1161/circresaha.116.309819
Koelwyn, G. J., Corr, E. M., Erbay, E., and Moore, K. J. (2018). Regulation of Macrophage Immunometabolism in Atherosclerosis. Nat. Immunol. 19, 526–537. doi:10.1038/s41590-018-0113-3
Kumar, B. V., Connors, T. J., and Farber, D. L. (2018). Human T Cell Development, Localization, and Function throughout Life. Immunity 48, 202–213. doi:10.1016/j.immuni.2018.01.007
Lee, W., Wang, L. T., Yen, M. L., Hsu, P. J., Lee, Y. W., Liu, K. J., et al. (2021). Resident vs Nonresident Multipotent Mesenchymal Stromal Cell Interactions with B Lymphocytes Result in Disparate Outcomes. Stem Cell Transl Med 10, 711–724. doi:10.1002/sctm.20-0289
Lee, Y., El Andaloussi, S., and Wood, M. J. A. (2012). Exosomes and Microvesicles: Extracellular Vesicles for Genetic Information Transfer and Gene Therapy. Hum. Mol. Genet. 21, R125–R134. doi:10.1093/hmg/dds317
Lee, Y. S., and Radford, K. J. (2019). The Role of Dendritic Cells in Cancer. Int. Rev. Cel Mol Biol 348, 123–178. doi:10.1016/bs.ircmb.2019.07.006
Li, C., Jin, Y., Wei, S., Sun, Y., Jiang, L., Zhu, Q., et al. (2019). Hippo Signaling Controls NLR Family Pyrin Domain Containing 3 Activation and Governs Immunoregulation of Mesenchymal Stem Cells in Mouse Liver Injury. Hepatology 70, 1714–1731. doi:10.1002/hep.30700
Li, F., Guo, X., and Chen, S.-Y. (2017). Function and Therapeutic Potential of Mesenchymal Stem Cells in Atherosclerosis. Front. Cardiovasc. Med. 4, 32. doi:10.3389/fcvm.2017.00032
Li, Q., Sun, W., Wang, X., Zhang, K., Xi, W., and Gao, P. (2015b). Skin-Derived Mesenchymal Stem Cells Alleviate Atherosclerosis via Modulating Macrophage Function. Stem Cell Transl Med 4, 1294–1301. doi:10.5966/sctm.2015-0020
Li, T., Xia, M., Gao, Y., Chen, Y., and Xu, Y. (2015a). Human Umbilical Cord Mesenchymal Stem Cells: an Overview of Their Potential in Cell-Based Therapy. Expert Opin. Biol. Ther. 15, 1293–1306. doi:10.1517/14712598.2015.1051528
Liao, S., Zhang, Y., Ting, S., Zhen, Z., Luo, F., Zhu, Z., et al. (2019). Potent Immunomodulation and Angiogenic Effects of Mesenchymal Stem Cells versus Cardiomyocytes Derived from Pluripotent Stem Cells for Treatment of Heart Failure. Stem Cel Res Ther 10, 78. doi:10.1186/s13287-019-1183-3
Liénart, S., Merceron, R., Vanderaa, C., Lambert, F., Colau, D., Stockis, J., et al. (2018). Structural Basis of Latent TGF-Β1 Presentation and Activation by GARP on Human Regulatory T Cells. Science 362, 952–956. doi:10.1126/science.aau2909
Liu, F., Qiu, H., Xue, M., Zhang, S., Zhang, X., Xu, J., et al. (2019). MSC-secreted TGF-β Regulates Lipopolysaccharide-Stimulated Macrophage M2-like Polarization via the Akt/FoxO1 Pathway. Stem Cel Res Ther 10, 345. doi:10.1186/s13287-019-1447-y
Liu, Z., Mikrani, R., Zubair, H. M., Taleb, A., Naveed, M., Baig, M. M. F. A., et al. (2020). Systemic and Local Delivery of Mesenchymal Stem Cells for Heart Renovation: Challenges and Innovations. Eur. J. Pharmacol. 876, 173049. doi:10.1016/j.ejphar.2020.173049
Loo, T. T., Gao, Y., and Lazarevic, V. (2018). Transcriptional Regulation of CD4+TH Cells that Mediate Tissue Inflammation. J. Leukoc. Biol. 104, 1069–1085. doi:10.1002/jlb.1ri0418-152rr
Lopatina, T., Kalinina, N., Karagyaur, M., Stambolsky, D., Rubina, K., Revischin, A., et al. (2019). Correction: Adipose-Derived Stem Cells Stimulate Regeneration of Peripheral Nerves: BDNF Secreted by These Cells Promotes Nerve Healing and Axon Growth De Novo. PLoS One 14, e0219946. doi:10.1371/journal.pone.0219946
Lu, H., Zhang, Y., Xiong, S., Zhou, Y., Xiao, L., Ma, Y., et al. (2021). Modulatory Role of Silver Nanoparticles and Mesenchymal Stem Cell-Derived Exosome-Modified Barrier Membrane on Macrophages and Osteogenesis. Front. Chem. 9, 699802. doi:10.3389/fchem.2021.699802
Luger, D., Lipinski, M. J., Westman, P. C., Glover, D. K., Dimastromatteo, J., Frias, J. C., et al. (2017). Intravenously Delivered Mesenchymal Stem Cells. Circ. Res. 120, 1598–1613. doi:10.1161/circresaha.117.310599
Luo, L., Tang, J., Nishi, K., Yan, C., Dinh, P.-U., Cores, J., et al. (2017). Fabrication of Synthetic Mesenchymal Stem Cells for the Treatment of Acute Myocardial Infarction in Mice. Circ. Res. 120, 1768–1775. doi:10.1161/circresaha.116.310374
Lv, K., Li, Q., Zhang, L., Wang, Y., Zhong, Z., Zhao, J., et al. (2019). Incorporation of Small Extracellular Vesicles in Sodium Alginate Hydrogel as a Novel Therapeutic Strategy for Myocardial Infarction. Theranostics 9, 7403–7416. doi:10.7150/thno.32637
Ma, J., Chen, L., Zhu, X., Li, Q., Hu, L., and Li, H. (2021). Mesenchymal Stem Cell-Derived Exosomal miR-21a-5p Promotes M2 Macrophage Polarization and Reduces Macrophage Infiltration to Attenuate Atherosclerosis. Acta Biochim. Biophys. Sin (Shanghai) 53, 1227–1236. doi:10.1093/abbs/gmab102
Ma, S. Q., Wei, H. L., and Zhang, X. (2018). TLR2 Regulates Allergic Airway Inflammation through NF-Κb and MAPK Signaling Pathways in Asthmatic Mice. Eur. Rev. Med. Pharmacol. Sci. 22, 3138–3146. doi:10.26355/eurrev_201805_15073
Magatti, M., Masserdotti, A., Bonassi Signoroni, P., Vertua, E., Stefani, F. R., Silini, A. R., et al. (2020). B Lymphocytes as Targets of the Immunomodulatory Properties of Human Amniotic Mesenchymal Stromal Cells. Front. Immunol. 11, 1156. doi:10.3389/fimmu.2020.01156
Martinez, V. G., Ontoria-Oviedo, I., Ricardo, C. P., Harding, S. E., Sacedon, R., Varas, A., et al. (2017). Overexpression of Hypoxia-Inducible Factor 1 Alpha Improves Immunomodulation by Dental Mesenchymal Stem Cells. Stem Cel Res Ther 8, 208. doi:10.1186/s13287-017-0659-2
Mauri, C., and Bosma, A. (2012). Immune Regulatory Function of B Cells. Annu. Rev. Immunol. 30, 221–241. doi:10.1146/annurev-immunol-020711-074934
Mekhloufi, A., Kosta, A., Stabile, H., Molfetta, R., Zingoni, A., Soriani, A., et al. (2020). Bone Marrow Stromal Cell-Derived IL-8 Upregulates PVR Expression on Multiple Myeloma Cells via NF-kB Transcription Factor. Cancers 12, 440. doi:10.3390/cancers12020440
Meng, X., Li, J., Yu, M., Yang, J., Zheng, M., Zhang, J., et al. (2018). Transplantation of Mesenchymal Stem Cells Overexpressing IL10 Attenuates Cardiac Impairments in Rats with Myocardial Infarction. J. Cel Physiol 233, 587–595. doi:10.1002/jcp.25919
Monguió-Tortajada, M., Prat-Vidal, C., Moron-Font, M., Clos-Sansalvador, M., Calle, A., Gastelurrutia, P., et al. (2021). Local Administration of Porcine Immunomodulatory, Chemotactic and Angiogenic Extracellular Vesicles Using Engineered Cardiac Scaffolds for Myocardial Infarction. Bioactive Mater. 6, 3314–3327. doi:10.1016/j.bioactmat.2021.02.026
Morvan, M. G., and Lanier, L. L. (2016). NK Cells and Cancer: You Can Teach Innate Cells New Tricks. Nat. Rev. Cancer 16, 7–19. doi:10.1038/nrc.2015.5
Namba, F. (2019). Mesenchymal Stem Cells for the Prevention of Bronchopulmonary Dysplasia. Pediatr. Int. 61, 945–950. doi:10.1111/ped.14001
Ohshima, M., Yamahara, K., Ishikane, S., Harada, K., Tsuda, H., Otani, K., et al. (2012). Systemic Transplantation of Allogenic Fetal Membrane-Derived Mesenchymal Stem Cells Suppresses Th1 and Th17 T Cell Responses in Experimental Autoimmune Myocarditis. J. Mol. Cell Cardiol. 53, 420–428. doi:10.1016/j.yjmcc.2012.06.020
Okada, H., Suzuki, J.-i., Futamatsu, H., Maejima, Y., Hirao, K., and Isobe, M. (2007). Attenuation of Autoimmune Myocarditis in Rats by Mesenchymal Stem Cell Transplantation through Enhanced Expression of Hepatocyte Growth Factor. Int. Heart J. 48, 649–661. doi:10.1536/ihj.48.649
Overgaard, N. H., Jung, J.-W., Steptoe, R. J., and Wells, J. W. (2015). CD4+/CD8+double-positive T Cells: More Than Just a Developmental Stage? J. Leukoc. Biol. 97, 31–38. doi:10.1189/jlb.1RU0814-382
Özgül Özdemir, R. B., Özdemir, A. T., Kırmaz, C., Eker Sarıboyacı, A., Karaöz, E., Erman, G., et al. (2021). Age-related Changes in the Immunomodulatory Effects of Human Dental Pulp Derived Mesenchymal Stem Cells on the CD4+ T Cell Subsets. Cytokine 138, 155367. doi:10.1016/j.cyto.2020.155367
P. De Miguel, M., Fuentes-Julian, S., Blazquez-Martinez, A., Y. Pascual, C., A. Aller, M., Arias, J., et al. (2012). Immunosuppressive Properties of Mesenchymal Stem Cells: Advances and Applications. Cmm 12, 574–591. doi:10.2174/156652412800619950
Peng, Y., Chen, X., Liu, Q., Xu, D., Zheng, H., Liu, L., et al. (2014). Alteration of Naïve and Memory B-Cell Subset in Chronic Graft-Versus-Host Disease Patients after Treatment with Mesenchymal Stromal Cells. Stem Cell Transl Med 3, 1023–1031. doi:10.5966/sctm.2014-0001
Peng, Y., Pan, W., Ou, Y., Xu, W., Kaelber, S., Borlongan, C. V., et al. (2016). Extracardiac-Lodged Mesenchymal Stromal Cells Propel an Inflammatory Response against Myocardial Infarction via Paracrine Effects. Cel Transpl. 25, 929–935. doi:10.3727/096368915x689758
Petri, R. M., Hackel, A., Hahnel, K., Dumitru, C. A., Bruderek, K., Flohe, S. B., et al. (2017). Activated Tissue-Resident Mesenchymal Stromal Cells Regulate Natural Killer Cell Immune and Tissue-Regenerative Function. Stem Cel Rep. 9, 985–998. doi:10.1016/j.stemcr.2017.06.020
Philipp, D., Holthaus, M., Basoah, V., Pfannkuche, K., Suhr, L., Wahlers, T., et al. (2021). VEGF Contributes to Mesenchymal Stem Cell-Mediated Reversion of Nor1-dependent Hypertrophy in iPS Cell-Derived Cardiomyocytes. Stem Cell Int. 2021, 1–19. doi:10.1155/2021/8888575
Pontikoglou, C., Kastrinaki, M.-C., Klaus, M., Kalpadakis, C., Katonis, P., Alpantaki, K., et al. (2013). Study of the Quantitative, Functional, Cytogenetic, and Immunoregulatory Properties of Bone Marrow Mesenchymal Stem Cells in Patients with B-Cell Chronic Lymphocytic Leukemia. Stem Cell Develop. 22, 1329–1341. doi:10.1089/scd.2012.0255
Poynter, J. A., Herrmann, J. L., Manukyan, M. C., Wang, Y., Abarbanell, A. M., Weil, B. R., et al. (2011). Intracoronary Mesenchymal Stem Cells Promote Postischemic Myocardial Functional Recovery, Decrease Inflammation, and Reduce Apoptosis via a Signal Transducer and Activator of Transcription 3 Mechanism. J. Am. Coll. Surgeons 213, 253–260. doi:10.1016/j.jamcollsurg.2011.04.005
Qin, Y., Zhou, Z., Zhang, F., Wang, Y., Shen, B., Liu, Y., et al. (2015). Induction of Regulatory B-Cells by Mesenchymal Stem Cells Is Affected by SDF-1α-CXCR7. Cell Physiol Biochem 37, 117–130. doi:10.1159/000430338
Qingqing, M., Xin, Z., and Meizhong, S. (2014). Bone Marrow Mesenchymal Stem Cells Altered the Immunoregulatory Activities of Hepatic Natural Killer Cells. Clin. Res. Hepatol. Gastroenterol. 38, 689–698. doi:10.1016/j.clinre.2014.06.001
Rasmusson, I., Le Blanc, K., Sundberg, B., and Ringdén, O. (2007). Mesenchymal Stem Cells Stimulate Antibody Secretion in Human B Cells. Scand. J. Immunol. 65, 336–343. doi:10.1111/j.1365-3083.2007.01905.x
Riazifar, M., Mohammadi, M. R., Pone, E. J., Yeri, A., Lässer, C., Segaliny, A. I., et al. (2019). Stem Cell-Derived Exosomes as Nanotherapeutics for Autoimmune and Neurodegenerative Disorders. ACS Nano 13, 6670–6688. doi:10.1021/acsnano.9b01004
Saraiva, M., and O'Garra, A. (2010). The Regulation of IL-10 Production by Immune Cells. Nat. Rev. Immunol. 10, 170–181. doi:10.1038/nri2711
Sava, R., Pepine, C., and March, K. (2020). Immune Dysregulation in HFpEF: A Target for Mesenchymal Stem/Stromal Cell Therapy. Jcm 9, 241. doi:10.3390/jcm9010241
Shao, M., Wang, D., Zhou, Y., Du, K., and Liu, W. (2020). Interleukin-10 Delivered by Mesenchymal Stem Cells Attenuates Experimental Autoimmune Myocarditis. Int. Immunopharmacology 81, 106212. doi:10.1016/j.intimp.2020.106212
Sharma, V., Dash, S. K., Manhas, A., Radhakrishnan, J., Jagavelu, K., and Verma, R. S. (2021). Injectable Hydrogel for Co-delivery of 5-azacytidine in Zein Protein Nanoparticles with Stem Cells for Cardiac Function Restoration. Int. J. Pharmaceutics 603, 120673. doi:10.1016/j.ijpharm.2021.120673
Sheu, J.-J., Chai, H.-T., Sung, P.-H., Chiang, J. Y., Huang, T.-H., Shao, P.-L., et al. (2021). Double Overexpression of miR-19a and miR-20a in Induced Pluripotent Stem Cell-Derived Mesenchymal Stem Cells Effectively Preserves the Left Ventricular Function in Dilated Cardiomyopathic Rat. Stem Cel Res Ther 12, 371. doi:10.1186/s13287-021-02440-4
Shi, H., Liang, M., Chen, W., Sun, X., Wang, X., Li, C., et al. (2018b). Human Induced Pluripotent Stem Cell-derived M-esenchymal S-tem C-ells A-lleviate A-therosclerosis by M-odulating I-nflammatory R-esponses. Mol. Med. Rep. 17, 1461–1468. doi:10.3892/mmr.2017.8075
Shi, Y., Wang, Y., Li, Q., Liu, K., Hou, J., Shao, C., et al. (2018a). Immunoregulatory Mechanisms of Mesenchymal Stem and Stromal Cells in Inflammatory Diseases. Nat. Rev. Nephrol. 14, 493–507. doi:10.1038/s41581-018-0023-5
Shin, E. Y., Wang, L., Zemskova, M., Deppen, J., Xu, K., Strobel, F., et al. (2018). Adenosine Production by Biomaterial‐Supported Mesenchymal Stromal Cells Reduces the Innate Inflammatory Response in Myocardial Ischemia/Reperfusion Injury. Jaha 7. doi:10.1161/jaha.117.006949
Shou, P., Chen, Q., Jiang, J., Xu, C., Zhang, J., Zheng, C., et al. (2016). Type I Interferons Exert Anti-tumor Effect via Reversing Immunosuppression Mediated by Mesenchymal Stromal Cells. Oncogene 35, 5953–5962. doi:10.1038/onc.2016.128
Silva, D. N., Souza, B. S. F., Vasconcelos, J. F., Azevedo, C. M., Valim, C. X. R., Paredes, B. D., et al. (2018). Granulocyte-Colony Stimulating Factor-Overexpressing Mesenchymal Stem Cells Exhibit Enhanced Immunomodulatory Actions through the Recruitment of Suppressor Cells in Experimental Chagas Disease Cardiomyopathy. Front. Immunol. 9, 1449. doi:10.3389/fimmu.2018.01449
Souza, B. S. d. F., Silva, K. N. d., Silva, D. N., Rocha, V. P. C., Paredes, B. D., Azevedo, C. M., et al. (2017). Galectin-3 Knockdown Impairs Survival, Migration, and Immunomodulatory Actions of Mesenchymal Stromal Cells in a Mouse Model of Chagas Disease Cardiomyopathy. Stem Cell Int. 2017, 1–13. doi:10.1155/2017/3282656
Spaggiari, G. M., Abdelrazik, H., Becchetti, F., and Moretta, L. (2009). MSCs Inhibit Monocyte-Derived DC Maturation and Function by Selectively Interfering with the Generation of Immature DCs: central Role of MSC-Derived Prostaglandin E2. Blood 113, 6576–6583. doi:10.1182/blood-2009-02-203943
Spaggiari, G. M., Capobianco, A., Abdelrazik, H., Becchetti, F., Mingari, M. C., and Moretta, L. (2008). Mesenchymal Stem Cells Inhibit Natural Killer-Cell Proliferation, Cytotoxicity, and Cytokine Production: Role of Indoleamine 2,3-dioxygenase and Prostaglandin E2. Blood 111, 1327–1333. doi:10.1182/blood-2007-02-074997
Spaggiari, G. M., Capobianco, A., Becchetti, S., Mingari, M. C., and Moretta, L. (2006). Mesenchymal Stem Cell-Natural Killer Cell Interactions: Evidence that Activated NK Cells Are Capable of Killing MSCs, whereas MSCs Can Inhibit IL-2-induced NK-Cell Proliferation. Blood 107, 1484–1490. doi:10.1182/blood-2005-07-2775
Spees, J. L., Lee, R. H., and Gregory, C. A. (2016). Mechanisms of Mesenchymal Stem/stromal Cell Function. Stem Cel Res Ther 7, 125. doi:10.1186/s13287-016-0363-7
Sun, X., Shan, A., Wei, Z., and Xu, B. (2018). Intravenous Mesenchymal Stem Cell-Derived Exosomes Ameliorate Myocardial Inflammation in the Dilated Cardiomyopathy. Biochem. Biophysical Res. Commun. 503, 2611–2618. doi:10.1016/j.bbrc.2018.08.012
Takafuji, Y., Hori, M., Mizuno, T., and Harada-Shiba, M. (2019). Humoral Factors Secreted from Adipose Tissue-Derived Mesenchymal Stem Cells Ameliorate Atherosclerosis in Ldlr−/− Mice. Cardiovasc. Res. 115, 1041–1051. doi:10.1093/cvr/cvy271
Tanaka, T., Narazaki, M., and Kishimoto, T. (2014). IL-6 in Inflammation, Immunity, and Disease. Cold Spring Harbor Perspect. Biol. 6, a016295. doi:10.1101/cshperspect.a016295
Terai, S., Tsuchiya, A., Watanabe, Y., and Takeuchi, S. (2021). Transition of Clinical and Basic Studies on Liver Cirrhosis Treatment Using Cells to Seek the Best Treatment. Inflamm. Regener 41, 27. doi:10.1186/s41232-021-00178-3
Uccelli, A., Moretta, L., and Pistoia, V. (2008). Mesenchymal Stem Cells in Health and Disease. Nat. Rev. Immunol. 8, 726–736. doi:10.1038/nri2395
van den Hoogen, P., de Jager, S. C. A., Mol, E. A., Schoneveld, A. S., Huibers, M. M. H., Vink, A., et al. (2019). Potential of Mesenchymal- and Cardiac Progenitor Cells for Therapeutic Targeting of B-Cells and Antibody Responses in End-Stage Heart Failure. PLoS One 14, e0227283. doi:10.1371/journal.pone.0227283
Van Linthout, S., Savvatis, K., Miteva, K., Peng, J., Ringe, J., Warstat, K., et al. (2011). Mesenchymal Stem Cells Improve Murine Acute Coxsackievirus B3-Induced Myocarditis. Eur. Heart J. 32, 2168–2178. doi:10.1093/eurheartj/ehq467
Wang, H., Chen, T., Ding, T., Zhu, P., Xu, X., Yu, L., et al. (2011). Adipogenic Differentiation Alters the Immunoregulatory Property of Mesenchymal Stem Cells through BAFF Secretion. Hematology 16, 313–323. doi:10.1179/102453311x13085644679944
Wang, M., Yan, L., Li, Q., Yang, Y., Turrentine, M., March, K., et al. (2020). Mesenchymal Stem Cell Secretions Improve Donor Heart Function Following Ex Vivo Cold Storage. J. Thorac. Cardiovasc. Surg. doi:10.1016/j.jtcvs.2020.08.095
Wang, S.-s., Hu, S.-w., Zhang, Q.-h., Xia, A.-x., Jiang, Z.-x., and Chen, X.-m. (2015). Mesenchymal Stem Cells Stabilize Atherosclerotic Vulnerable Plaque by Anti-inflammatory Properties. PLoS One 10, e0136026. doi:10.1371/journal.pone.0136026
Wang, S., Zhu, R., Li, H., Li, J., Han, Q., and Zhao, R. C. (2019). Mesenchymal Stem Cells and Immune Disorders: from Basic Science to Clinical Transition. Front. Med. 13, 138–151. doi:10.1007/s11684-018-0627-y
Wang, X., Lazorchak, A. S., Song, L., Li, E., Zhang, Z., Jiang, B., et al. (2016b). Immune Modulatory Mesenchymal Stem Cells Derived from Human Embryonic Stem Cells through a Trophoblast‐like Stage. Stem Cells 34, 380–391. doi:10.1002/stem.2242
Wang, Y.-C., Ma, H.-D., Yin, X.-Y., Wang, Y.-H., Liu, Q.-Z., Yang, J.-B., et al. (2016a). Forkhead Box O1 Regulates Macrophage Polarization Following Staphylococcus aureus Infection: Experimental Murine Data and Review of the Literature. Clinic Rev. Allerg Immunol. 51, 353–369. doi:10.1007/s12016-016-8531-1
Wang, Z., and Zhao, Y. (2018). Gut Microbiota Derived Metabolites in Cardiovascular Health and Disease. Protein Cell 9, 416–431. doi:10.1007/s13238-018-0549-0
Wei, Z., Qiao, S., Zhao, J., Liu, Y., Li, Q., Wei, Z., et al. (2019). miRNA-181a Over-expression in Mesenchymal Stem Cell-Derived Exosomes Influenced Inflammatory Response after Myocardial Ischemia-Reperfusion Injury. Life Sci. 232, 116632. doi:10.1016/j.lfs.2019.116632
Wing, J. B., Tanaka, A., and Sakaguchi, S. (2019). Human FOXP3+ Regulatory T Cell Heterogeneity and Function in Autoimmunity and Cancer. Immunity 50, 302–316. doi:10.1016/j.immuni.2019.01.020
Wynn, T. A., Chawla, A., and Pollard, J. W. (2013). Macrophage Biology in Development, Homeostasis and Disease. Nature 496, 445–455. doi:10.1038/nature12034
Xu, R., Zhang, F., Chai, R., Zhou, W., Hu, M., Liu, B., et al. (2019). Exosomes Derived from Pro‐inflammatory Bone Marrow‐derived Mesenchymal Stem Cells Reduce Inflammation and Myocardial Injury via Mediating Macrophage Polarization. J. Cel Mol Med 23, 7617–7631. doi:10.1111/jcmm.14635
Yang, F. Y., Chen, R., Zhang, X., Huang, B., Tsang, L. L., Li, X., et al. (2018). Preconditioning Enhances the Therapeutic Effects of Mesenchymal Stem Cells on Colitis through PGE2-Mediated T-Cell Modulation. Cel Transpl. 27, 1352–1367. doi:10.1177/0963689718780304
Yen, B. L., Yen, M. L., Wang, L. T., Liu, K. J., and Sytwu, H. K. (2020). Current Status of Mesenchymal Stem Cell Therapy for Immune/inflammatory Lung Disorders: Gleaning Insights for Possible Use in COVID ‐19. Stem Cell Transl Med 9, 1163–1173. doi:10.1002/sctm.20-0186
Yoshida, S., Miyagawa, S., Toyofuku, T., Fukushima, S., Kawamura, T., Kawamura, A., et al. (2020). Syngeneic Mesenchymal Stem Cells Reduce Immune Rejection after Induced Pluripotent Stem Cell-Derived Allogeneic Cardiomyocyte Transplantation. Sci. Rep. 10, 4593. doi:10.1038/s41598-020-58126-z
Zhang, N., Song, Y., Huang, Z., Chen, J., Tan, H., Yang, H., et al. (2020b). Monocyte Mimics Improve Mesenchymal Stem Cell-Derived Extracellular Vesicle Homing in a Mouse MI/RI Model. Biomaterials 255, 120168. doi:10.1016/j.biomaterials.2020.120168
Zhang, Q., Fu, L., Liang, Y., Guo, Z., Wang, L., Ma, C., et al. (2018). Exosomes Originating from MSCs Stimulated with TGF‐β and IFN‐γ Promote Treg Differentiation. J. Cel Physiol 233, 6832–6840. doi:10.1002/jcp.26436
Zhang, Q., Iida, R., Shimazu, T., and Kincade, P. W. (2012). Replenishing B Lymphocytes in Health and Disease. Curr. Opin. Immunol. 24, 196–203. doi:10.1016/j.coi.2011.12.007
Zhang, R., Ma, J., Han, J., Zhang, W., and Ma, J. (2019). Mesenchymal Stem Cell Related Therapies for Cartilage Lesions and Osteoarthritis. Am. J. Transl Res. 11, 6275–6289.
Zhang, Y., Ge, X.-h., Guo, X.-J., Guan, S.-b., Li, X.-m., Gu, W., et al. (2017). Bone Marrow Mesenchymal Stem Cells Inhibit the Function of Dendritic Cells by Secreting Galectin-1. Biomed. Res. Int. 2017, 1–19. doi:10.1155/2017/3248605
Zhang, Z., Tian, H., Yang, C., Liu, J., Zhang, H., Wang, J., et al. (2020a). Mesenchymal Stem Cells Promote the Resolution of Cardiac Inflammation after Ischemia Reperfusion via Enhancing Efferocytosis of Neutrophils. Jaha 9, e014397. doi:10.1161/jaha.119.014397
Zhao, J., Li, X., Hu, J., Chen, F., Qiao, S., Sun, X., et al. (2019). Mesenchymal Stromal Cell-Derived Exosomes Attenuate Myocardial Ischaemia-Reperfusion Injury through miR-182-Regulated Macrophage Polarization. Cardiovasc. Res. 115, 1205–1216. doi:10.1093/cvr/cvz040
Zhou, C., Wu, X.-R., Liu, H.-S., Liu, X.-H., Liu, G.-H., Zheng, X.-B., et al. (2020). Immunomodulatory Effect of Urine-Derived Stem Cells on Inflammatory Bowel Diseases via Downregulating Th1/Th17 Immune Responses in a PGE2-dependent Manner. J. Crohns Colitis 14, 654–668. doi:10.1093/ecco-jcc/jjz200
Keywords: cardiovascular disease, immune regulation, inflammation, mesenchymal stem cells, immune cells
Citation: Wang Y, Qi Z, Yan Z, Ji N, Yang X, Gao D, Hu L, Lv H, Zhang J and Li M (2022) Mesenchymal Stem Cell Immunomodulation: A Novel Intervention Mechanism in Cardiovascular Disease. Front. Cell Dev. Biol. 9:742088. doi: 10.3389/fcell.2021.742088
Received: 15 July 2021; Accepted: 14 December 2021;
Published: 12 January 2022.
Edited by:
Ming Li, Osaka University, JapanReviewed by:
Men-Luh Yen, National Taiwan University, TaiwanRuili Yang, Peking University Hospital of Stomatology, China
Copyright © 2022 Wang, Qi, Yan, Ji, Yang, Gao, Hu, Lv, Zhang and Li. This is an open-access article distributed under the terms of the Creative Commons Attribution License (CC BY). The use, distribution or reproduction in other forums is permitted, provided the original author(s) and the copyright owner(s) are credited and that the original publication in this journal is cited, in accordance with accepted academic practice. No use, distribution or reproduction is permitted which does not comply with these terms.
*Correspondence: Junping Zhang, tjzhtcm@163.com; Meng Li, zhongyilimeng@163.com
†These authors have contributed equally to this work