g-C3N4 Modified by meso-Tetrahydroxyphenylchlorin for Photocatalytic Hydrogen Evolution Under Visible/Near-Infrared Light
- 1Shanghai Key Laboratory of Atmospheric Particle Pollution and Prevention (LAP3), Department of Environmental Science and Engineering, Fudan University, Shanghai, China
- 2Shanghai Institute of Pollution Control and Ecological Security, Shanghai, China
A new photocatalyst denoted as mTHPC/pCN was prepared by modifying protonated graphitic carbon nitride (pCN) by meso-tetrahydroxyphenylchlorin (mTHPC). Relevant samples were characterized via various methods including zeta potential measurements, X-ray diffraction, Fourier transform infrared spectroscopy, X-ray photoelectron spectroscopy, N2 adsorption–desorption, transmission electron microscopy, ultraviolet-visible–near-infrared spectroscopy, electrochemical impedance spectroscopy, photocurrent response measurements, electron spin resonance spectroscopy, and phosphorescence spectroscopy. Compared with pCN, mTHPC/pCN shows enhanced absorption in the visible and near-infrared regions and thus higher photocatalytic activity in hydrogen evolution. A possible mechanism for mTHPC/pCN is proposed.
Introduction
Graphitic carbon nitride (g-C3N4) is a new type of photocatalyst with unique physicochemical characteristics (Zheng et al., 2015). The π-conjugated system of g-C3N4 allows for the transfer of charge carriers, and a band gap of around 2.7 eV allows it to work under visible (VIS) light (Wang et al., 2009; Ong et al., 2017). In addition, g-C3N4 is thermally and chemically stable. It can be prepared by the thermal polycondensation of inexpensive nitrogen-containing carbon-based precursors such as thiourea, melamine, urea, cyanamide, and dicyandiamide without difficulty (Panneri et al., 2017).
However, the low efficiency in VIS light absorption, high recombination rate of photogenerated electrons and holes, low conductivity, and low specific surface areas (SSAs) of g-C3N4 may limit its photocatalytic performance (Zou et al., 2016; Mishra et al., 2019). g-C3N4 nanorods/nanotubes (Li et al., 2015; Liu et al., 2017), nanasheets (Zhang J. S. et al., 2015; Murugesan et al., 2019), and porous structures (Zeng et al., 2016; Liu M. J. et al., 2019) have been developed. Metal elements (e.g., Ag; Ge et al., 2011, Cu; Fan et al., 2016, Au; Caux et al., 2019, Pt; Zhou et al., 2019) and non-metal elements (e.g., C; Zhao et al., 2015, N; Fang et al., 2015, P; Ran et al., 2015, Br; Lan et al., 2016, O; Wei et al., 2018, S; Xiao et al., 2020) have been doped into g-C3N4. In addition, g-C3N4-based heterojunctions (e.g., Bi2O2CO3/g-C3N4; Wang Z. Y. et al., 2016, CoTiO3/g-C3N4; Ye et al., 2016, Ag2MoO4/g-C3N4; Zhang and Ma, 2017b, C/g-C3N4; Shen et al., 2017, Ag6Mo10O33/g-C3N4; Zhang and Ma, 2017a, MoS2/g-C3N4; Liu Y. Z. et al., 2018, Bi3O4Cl/g-C3N4; Che et al., 2018, TiO2/g-C3N4; Tao et al., 2019, WO3/g-C3N4; Fu et al., 2019, CdS/g-C3N4; Qiu et al., 2020, ZnO/g-C3N4; Gao et al., 2020, Ba5Nb4O15/g-C3N4; Wang et al., 2020, Co3(PO4)2/g-C3N4; Shi et al., 2020, Cs3Bi2I9/g-C3N4; Bresolin et al., 2020) have been developed to enhance the photocatalytic performance. However, few studies have aimed at extending the light absorption range of g-C3N4 to the near-infrared (NIR) region.
In the total solar spectrum, the ultraviolet (UV) light (λ < 400 nm), VIS light (400 < λ < 700 nm), and NIR light (λ > 700 nm) account for ~5, 43, and 52%, respectively (Li et al., 2016). Therefore, the development of g-C3N4-based photocatalysts that can absorb NIR light is important. Photosensitizers are the general term of molecules that can absorb light and transfer energy to other materials. Some researchers modified g-C3N4 with photosensitizers such as phthalocyanine (Zhang et al., 2014), a combination of organic dye and zinc phthalocyanine derivative (Zhang X. H. et al., 2015), μ-oxo dimeric iron (III) porphyrin (Wang D. H. et al., 2016), zinc phthalocyanine (Liu Q. W. et al., 2018), mesotetrakis (carboxyphenyl) porphyrins (Da Silva et al., 2018), copper octacarboxyphthalocyanine (Ouedraogo et al., 2018), zinc phthalocyanine derivative (Zeng et al., 2019), multiporphyrin (Yang et al., 2019), zinc (II) 1, 8(11), 15(18), 22(25)-tetrakis (4-carboxylphenoxy) phthalocyanine (α-ZnTcPc) (He et al., 2019), porphyrin (Tian et al., 2019), Chlorin e6 (Ce6) (Liu et al., 2020a), 3,4,9,10-perylenetetracarboxylic acid anhydride (PTCDA) (Yuan et al., 2020), tetra (4-carboxyphenyl) porphyrin iron (III) chloride (FeTCPP) (Zhang et al., 2020), protoporphyrin (Pp) (Liu et al., 2020b), and naphthalimide-porphyrin (Li L. L. et al., 2020). However, more examples in this regard are needed because this is a very interesting topic.
meso-Tetrahydroxyphenylchlorin (mTHPC or temoporfin) is an NIR light–absorbing photosensitizer used for clinical applications and photodynamic therapy (Navarro et al., 2014). In addition, mTHPC is a second-generation photosensitizer that shows some favorable characteristics under NIR light irradiation (Hinger et al., 2016). For example, multiwalled carbon nanotubes were modified with mTHPC for cancer treatment (Marangon et al., 2016). Polymeric micelles were modified with mTHPC for treating cardiovascular diseases (Wennink et al., 2017). Gold nanoparticles were modified with mTHPC for cancer therapeutic (Haimov et al., 2018). Poly(D,L-lactide-co-glycolide) acid nanoparticles were modified with mTHPC for in vitro photodynamic therapy (Boeuf-Muraille et al., 2019). However, to the best of our knowledge, g-C3N4 was not modified by mTHPC for photocatalysis.
Supplementary Figure 1 shows the chemical structures of mTHPC and bulk graphitic carbon nitride (bCN). mTHPC has many hydroxyl (–OH) groups, and bCN has many –C–N– groups, making the surfaces of both materials negatively charged (Supplementary Figure 2) and thus difficult to combine with each other. Herein, protonated graphitic carbon nitride (pCN) was obtained by treating bCN with hydrochloric acid (HCl) solution (Xie et al., 2018). The surface zeta potential of pCN is positively charged (Supplementary Figure 2), so the negatively charged mTHPC may be combined with pCN to yield a composite photocatalyst (Figure 1) that can work more efficiently under VIS light and NIR light.
Experimental Section
Synthesis of Bulk Graphitic Carbon Nitride (bCN)
bCN was prepared by high-temperature calcination in a muffle furnace. The details are as follows: 12 g melamine was placed in a 50 mL quartz crucible with a lid, and then the quartz crucible was placed in a muffle furnace. The heating rate was set to be 5°C · min−1. The muffle furnace was heated to 550°C, and the temperature was held for 2 h (Cui et al., 2018a). After the muffle furnace was cooled down, the remaining powders (bCN) were collected and ground for further use.
Preparation of pCN
pCN was obtained by treating bCN with HCl solution. The details are as follows: 1 g bCN was placed in 200 mL HCl solution (1 mol · L−1), and the slurry was magnetically stirred at room temperature for 4 h (Cui et al., 2018b). Subsequently, the sediment was collected by high-speed centrifugation, washed with deionized water three times, and dried at 80°C for 12 h. The obtained powders (pCN) were ground for further use.
Preparation of mTHPC/pCN and mTHPC/bCN
mTHPC/pCN was synthesized as follows: 0.5 g pCN was put into 200 mL deionized water, and then 0.05 g mTHPC was added, and the slurry was subjected to magnetic stirring for 2 h at room temperature. Subsequently, the sediment was collected by high-speed centrifugation, washed three times by deionized water, and dried at 80°C for 12 h. The obtained powders (mTHPC/pCN) were collected. In addition, a reference sample denoted as mTHPC/bCN was prepared under the same experimental conditions.
Characterization
The surface zeta potential data were obtained from a Zetasizer Nano ZS device (Malvern Instruments). X-ray diffraction (XRD) data were recorded using a Bruker Advanced D8 (Bruker Corp., Germany) instrument. Fourier transform infrared (FTIR) spectra were recorded by a Nicolet Nexus 470 instrument (Nicolet Instrument Corp., USA). X-ray photoelectron spectra (XPS) were analyzed by an ESCALAB 250 XPS instrument. Nitrogen (N2) adsorption–desorption were determined by a Tristar 3000 analyzer. Transmission electron microscopy (TEM) images were taken using a JEM-2100F microscope (JEOL, Japan). UV-VIS-NIR absorption spectra were measured by a Cary 5000 spectrophotometer. Electrochemical impedance spectra (EIS) and photocurrent response curves were obtained through a CHI660C electrochemical workstation. Electron spin resonance (ESR) spectra were recorded by a Bruker model A300 spectrometer at room temperature. Phosphorescence spectra were tested on a Hitachi F-4600 spectrometer under an excitation wavelength of 808 nm.
Photocatalytic Hydrogen Evolution
Photocatalytic hydrogen generation experiments were carried out as follows: 10 mg photocatalyst was placed in a 150 mL quartz reactor, 18 mL H2PtCl6 solution (0.045 mg · mL−1) was added, and then 2 mL triethanolamine (TEOA) was added. Subsequently, the mixed system was sonicated in an ultrasonic machine for 10 min to allow for the even dispersion of the photocatalyst and then purged with N2 for 30 min to remove air from the solution and the reactor. During photocatalytic process, the temperature of the photocatalytic reaction was controlled at about 12°C by using circulating cooling water, and a 300-W xenon (Xe) lamp was used as light source. A filter was used to get VIS-NIR light (λ > 420 nm). Another filter was used to get NIR light (λ > 780 nm).
The distance from the light source to the reaction system was 4 cm. The optical power density of the light source was 120 mW · cm−2 under VIS-NIR light irradiation and 10 mW · cm−2 under NIR light irradiation. The mixed gas composed of H2 produced by photocatalysis and N2 in the quartz reactor was automatically collected and analyzed using a gas chromatograph (GC7600, Tian Mei) every 1 h.
Results and Discussion
XRD and FTIR Spectra
Figure 2 shows the XRD data. The peak of bCN at 13.4° is attributed to the (100) plane (Inagaki et al., 2019; Qi et al., 2019), and the peak at 27.3° is attributed to the (002) plane (Liu L. et al., 2018; Lee et al., 2019). pCN has the same characteristic peaks as bCN, but the intensity of these peaks decreases to some extent, probably due to the delamination of bCN following the treatment of bCN by HCl solution (Liang et al., 2015; Prabavathi and Muthuraj, 2019). mTHPC shows some weak peaks in the range of 12 to 26° (Yuan et al., 2015a). The peaks of mTHPC are not observed in mTHPC/bCN. However, the peaks of mTHPC can be observed in mTHPC/pCN, indicating the uptake of mTHPC on pCN. In any case, bCN, pCN, mTHPC/bCN, and mTHPC/pCN all show typical g-C3N4 patterns.
Figure 3 shows the FTIR spectra of samples. The characteristic peaks of bCN at 800 cm−1 is ascribed to the tri-s-triazine ring units (Zhang et al., 2010; Wang et al., 2019); the broadband peaks at 1,200 to 1,700 nm−1 are attributed to the C–N heterocycles (Liu Q. et al., 2016), and the peaks at 3,000 to 3,500 nm−1 are attributed to the hydroxyl groups (O–H) and free amino groups (N–H) (Hang et al., 2017; He et al., 2020). pCN has the same characteristic peaks as bCN, but the intensities of these peaks increase to some extent. mTHPC shows peaks at 700 to 1,700 nm−1, corresponding to (N–H), (C–H), (C=C), and (C=N) (Da Silva et al., 2018). The peaks at 3,250 to 3,600 nm−1 of mTHPC are attributed to the N–H bonds (Yuan et al., 2015b). Although the peaks of mTHPC are not clearly observed in mTHPC/bCN and mTHPC/pCN, all the characteristic peaks of g-C3N4 in mTHPC/pCN are the strongest. This may be because mTHPC is loaded on the surface of pCN, which enhances the infrared absorption. A similar trend (enhanced IR absorption) is also seen in the Ce6/pCN (Liu et al., 2020a) and Pp/pGCN (Liu et al., 2020b) systems.
XPS Spectra
Figure 4 shows the XPS spectra of samples. In the survey XPS spectra of bCN and mTHPC/pCN (Figure 4A), the peaks of C 1s, N 1s, and O 1s can be obviously observed (Naseri et al., 2017; Zada et al., 2019). Figure 4B shows the high-resolution XPS spectra of C 1s. The two peaks at 284.5 and 287.9 eV are assigned to (C–C) and (N–C=N), respectively (Jiang et al., 2017; Liu X. C. et al., 2020). Figure 4C shows the high-resolution N 1s XPS spectra. The three peaks at 398.5, 399.6, and 400.6 eV are assigned to (C–N=C), (N–(C)3), and (C–N–H), respectively (Sun and Liang, 2017; Sun et al., 2020). Figure 4D shows the high-resolution O 1s XPS spectra. The three peaks at 531.1, 532.2, and 533.2 eV are due to O–C=O, C=O, and O–H, respectively (Teng et al., 2017; Zhang et al., 2017). Compared with bCN, the O 1s peaks (especially at 532.2 eV) of mTHPC/pCN are significantly enhanced, probably due to the oxygen-containing group in mTHPC.
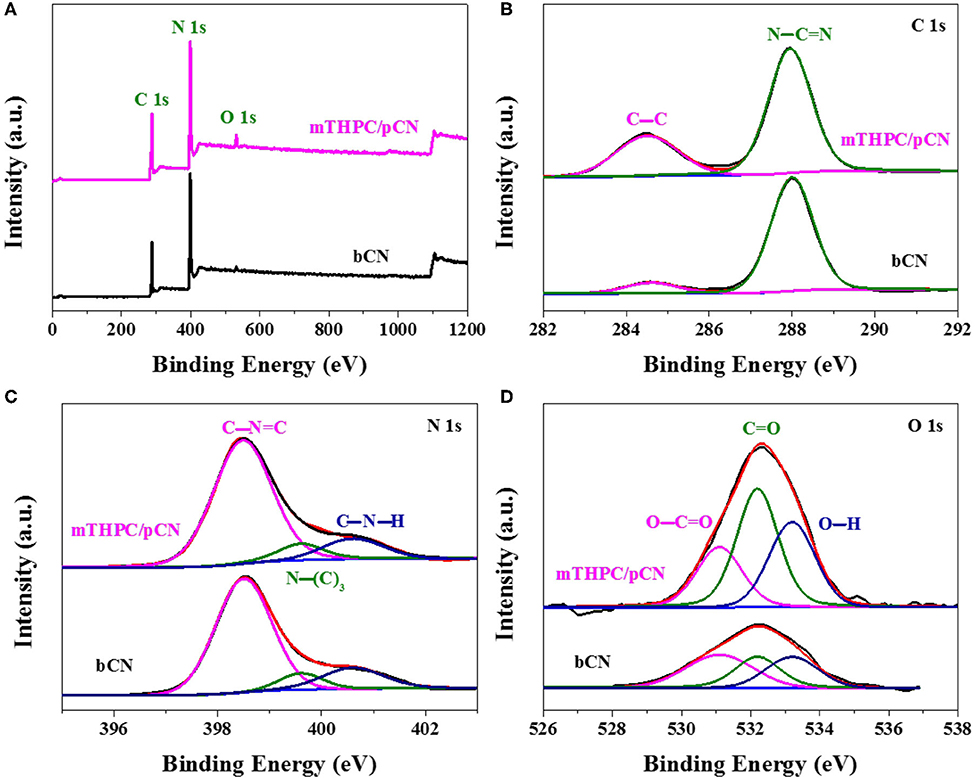
Figure 4. The survey XPS spectra (A), the high-resolution XPS spectra of C 1s (B), N 1s (C), and O 1s (D) for bCN and mTHPC/pCN.
N2 Adsorption–Desorption and TEM Analysis
Supplementary Figure 3 shows the N2 adsorption–desorption data. The N2 adsorption and desorption isotherms of samples (Supplementary Figure 3A) can be classified as type IV isotherms, signifying the presence of mesopores (2–50 nm) (Qin and Zeng, 2017). The hysteresis loops of samples belong to H3 type, indicating the existence of slit-type mesopores formed by the irregular accumulation of g-C3N4 nanosheets (Ding et al., 2017). Compared with bCN, the adsorption volume of pCN appears significantly enhanced and this trend may be due to the nanosheet structure caused by the delamination treatment of bCN in HCl solution. mTHPC/pCN well maintains the enhanced adsorption volume.
Supplementary Figure 3B shows that the four samples have the wide pore size distribution (2–70 nm), further indicating the presence of mesopores (Qiu et al., 2017). It is worth noting that the pore size distribution curve of pCN has a clear peak at 2 to 5 nm, indicating that the porous structure may be caused by HCl solution, and mTHPC/pCN well maintains the porous structure.
Supplementary Table 1 shows the SSA and pore volume of samples. The SSA and pore volume of bCN are 12.5 m2 · g−1 and 0.063 cm3 · g−1, respectively. pCN has the largest SSA and pore volume of 40.3 m2 · g−1 and 0.163 cm3 · g−1, probably due to the delamination treatment of bCN by HCl solution. mTHPC/pCN well maintains a larger SSA and pore volume of 30.8 m2 · g−1 and 0.126 cm3 · g−1, respectively.
Supplementary Figure 4 shows the TEM images of samples. bCN has the irregularly thick bulk structure (Yu et al., 2017). However, pCN shows the typical two-dimensional nanoflakes and porous structure, consistent with the pore size distribution curve (Mamba and Mishra, 2016). mTHPC/pCN well maintains the ultrathin holey nanosheet structure of pCN.
UV-VIS-NIR Absorption Spectra
Figure 5A shows the UV-VIS-NIR absorption spectra of photocatalysts. bCN and pCN only show obvious absorption capacity in the UV light and VIS light regions (Liu S. Z. et al., 2016) and have almost no absorption capacity in the NIR light region (λ > 780 nm). mTHPC has obvious absorption capacity of the UV light and VIS light and also has obvious absorption capacity in the NIR light range (λ > 780 nm). mTHPC/bCN and mTHPC/pCN not only maintain the absorption performance of bCN and pCN in the UV light and VIS light range, but also retain the absorption capacity of mTHPC in the NIR light range (λ > 780 nm) to some extent. Furthermore, the light absorption capacity of mTHPC/pCN is significantly better than that of mTHPC/bCN.
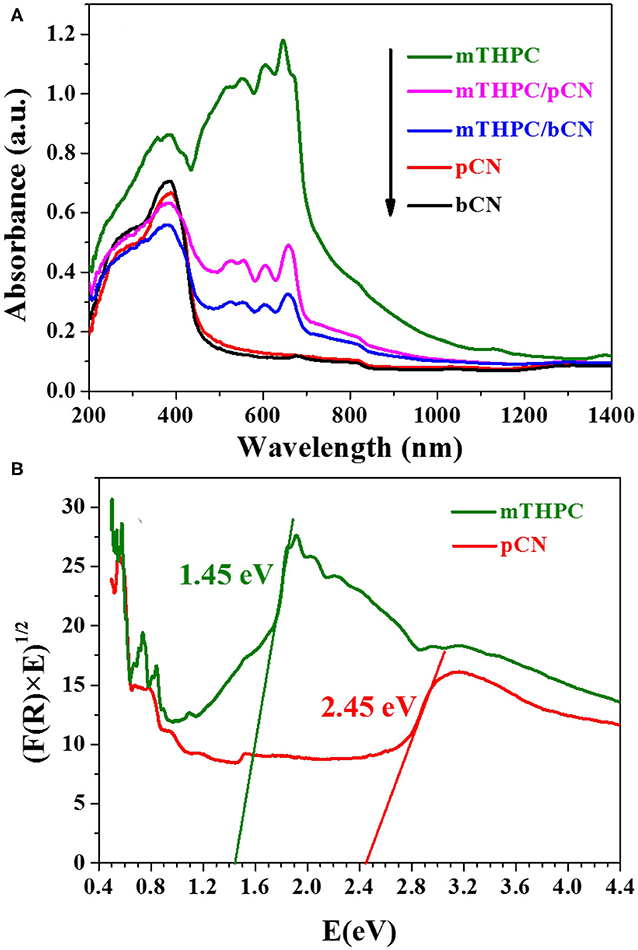
Figure 5. The UV-VIS-NIR absorption spectra of photocatalysts (A) and the calculated energy band gaps (Eg) of mTHPC and pCN (B).
The calculated energy band gaps (Eg) of mTHPC and pCN by the absorption spectra and the equation of αhν = A(hν – Eg)n/2 (Li et al., 2018, 2019) are 1.45 and 2.45 eV in Figure 5B, respectively. The α, h, ν, A, Eg, and n stand for the absorption coefficient, the Planck's constant, the light frequency, the proportionality constant, the energy band gap, and n = 1 for a direct band gap transition, respectively (Adhikari et al., 2017).
Photocatalytic Hydrogen Evolution
Photocatalytic hydrogen generation was performed under a 300-W xenon (Xe) lamp as the light source. Figure 6A shows the photocatalytic hydrogen production performance of samples under VIS-NIR light irradiation. A filter was used to get VIS-NIR light source (λ > 420 nm), 3 wt.% Pt was used as the co-catalyst, and TEOA was used as the sacrificial reagent. bCN and mTHPC show low hydrogen production performance; the average hydrogen evolution rates (HERs) are 120.6 and 87.4 μmol · h−1 · g−1, respectively.
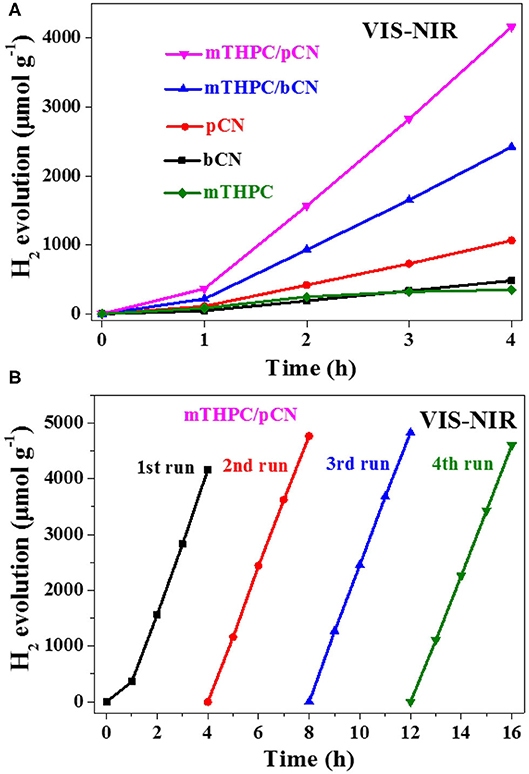
Figure 6. Photocatalytic hydrogen evolution activity from water of the five photocatalysts (A) and the cycle stability test of mTHPC/pCN (B) under VIS-NIR (λ > 420 nm) irradiation.
The average HER of pCN and mTHPC/bCN are 266.0 and 605.8 μmol · h−1 · g−1, respectively. mTHPC/pCN shows the highest average HER of 1,041.4 μmol · h−1 · g−1, somewhat lower than the average HER obtained by Ce6/pCN (1,275.6 μmol · h−1 · g−1) (Liu et al., 2020a) and Pp/pGCN (1,153.8 μmol · h−1 · g−1) (Liu et al., 2020b). Furthermore, mTHPC/pCN has good cycle stability under VIS-NIR irradiation in Figure 6B. The XRD patterns of the fresh mTHPC/pCN and the used mTHPC/pCN are shown in Supplementary Figure 5. Compared with the fresh mTHPC/pCN, the peak intensities of the used mTHPC/pCN decrease to some extent, and the used mTHPC/pCN well maintains the crystal structure of the fresh mTHPC/pCN.
Figure 7A shows the photocatalytic hydrogen production activity of samples under NIR light irradiation. A filter was used to get NIR light source (λ > 780 nm), Pt was used as the cocatalyst, and TEOA was used as the sacrificial reagent. bCN and pCN show trace amounts of hydrogen production, while mTHPC shows the low average HER of 25.1 μmol·h−1 · g−1.
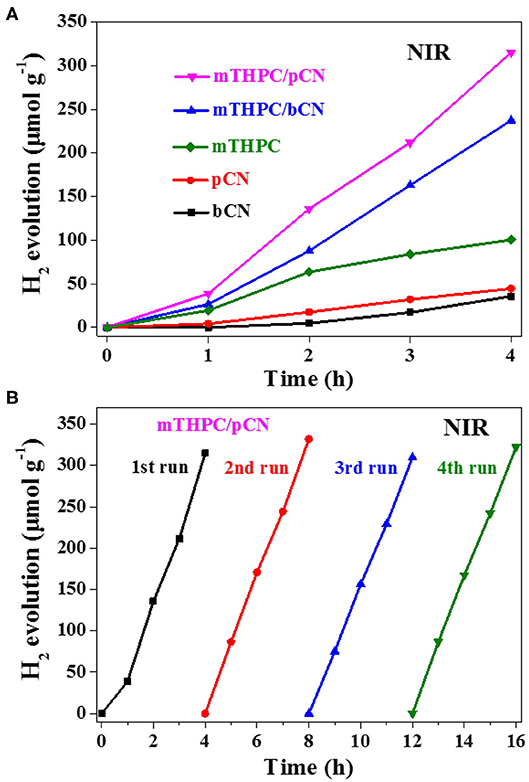
Figure 7. Photocatalytic hydrogen evolution activity from water of the five photocatalysts (A) and the cycle stability test of mTHPC/pCN (B) under NIR light (λ > 780 nm) irradiation.
The average HER of mTHPC/bCN is 59.3 μmol · h−1 · g−1. mTHPC/pCN shows the highest average HER of 78.8 μmol · h−1 · g−1, lower than the rate obtained by Ce6/pCN (312.6 μmol · h−1 · g−1) (Liu et al., 2020a) and Pp/pGCN (307.8 μmol · h−1 · g−1) (Liu et al., 2020b). This may be because the UV-VIS-NIR absorption capacity of mTHPC is lower than that of Ce6 and Pp (Supplementary Figure 6). In addition, mTHPC/pCN has good cycle stability under NIR irradiation in Figure 7B.
EIS, Photocurrent Response Curves, ESR, and Phosphorescence Spectra
Figure 8 shows the EIS comparison of bCN and mTHPC/pCN. The arc size in the high-frequency region of the Nyquist diagram is consistent with the electron transfer restriction mechanism, and the arc diameter is equal to the resistance of the electron transfer (She et al., 2016; Li S. J. et al., 2020). Obviously, the arc radius of mTHPC/pCN is significantly smaller than bCN, indicating that mTHPC/pCN can significantly retard the recombination of photogenerated electrons and holes and accelerate electron transfer (Li et al., 2017).
Figure 9 shows the transient photocurrent response curves comparison of bCN and mTHPC/pCN under VIS-NIR (λ > 420 nm) irradiation. When turning on the light source, the photocurrents of the two samples rise immediately (Shen et al., 2020a). Conversely, when turning off the light source, the photocurrents drop quickly (Luo et al., 2018; Li et al., 2021). The pattern can be repeated, indicating that photogenerated electrons can be transferred to the contact interface through the sample under light irradiation (Tian et al., 2017; Shen et al., 2020b). Further observation found that the photocurrent value of mTHPC/pCN is higher than bCN, indicating that the charge separation efficiency of mTHPC/pCN has significantly enhanced (An et al., 2017).
Figure 10 exhibits the ESR characterization of bCN and mTHPC/pCN under NIR light (λ > 780 nm) at room temperature. bCN and mTHPC/pCN both exhibit one single Lorentz line (g = 2.0034) from 3,200 to 3,800 G magnetic field (Liu G. et al., 2019; Jia et al., 2020). However, compared with bCN, the ESR intensity of mTHPC/pCN is much stronger, indicating that the concentration of unpaired electrons is much higher.
The phosphorescence spectra of mTHPC and mTHPC/pCN were tested at an excitation wavelength of 808 nm at room temperature in Figure 11. Obviously, the emission wavelength of mTHPC is in the range of VIS light (400–600 nm), indicating that the mTHPC has obvious up-conversion behavior (Wang et al., 2018). Compared with mTHPC, the emission peak intensity of mTHPC/pCN is significantly decreased, indicating the energy transfer from mTHPC to pCN.
Photocatalytic Mechanism
The energy band gaps (Eg) of mTHPC and pCN are 1.45 and 2.45 eV in Figure 5B, respectively. The XPS valence band (VB) top position of mTHPC and pCN are 0.62 and 1.29 V in Supplementary Figure 7, respectively. Thus, contrasting to the standard hydrogen electrode potential, the conduction bands (CBs) of mTHPC and pCN are −0.83 and −1.16 V, respectively.
A possible mechanism for mTHPC/pCN is proposed in Figure 12. When mTHPC/pCN is irradiated under VIS light, pCN is excited to generate electrons (e−) on CB and holes (h+) on VB. Because the CB edge potential of pCN (−1.16 V) is more negative than that of mTHPC (−0.83 V), the e− on pCN could transfer to the CB of mTHPC. Pt was used as the cocatalyst (Figure 13 and Supplementary Figure 8) obtained from the Pt precursor (H2PtCl6 · 6H2O) by the in situ photoreduction (Sui et al., 2013; Pan et al., 2017). Pt nanoparticles may slightly enhance absorption intensity in NIR region (Supplementary Figure 9) due to the light scattering phenomenon of Pt (Shiraishi et al., 2014; Chen et al., 2020). Pt can quickly transfer e− which can reduce H+ to H2 (Xing et al., 2014; Zhou et al., 2019). Indeed, the photocatalytic hydrogen evolution performance is significantly enhanced (Supplementary Figure 10). In addition, because the VB of pCN (1.29 V) is more positive than that of mTHPC (0.62 V), the h+ on pCN could transfer to the VB of mTHPC. As the sacrificial agent, TEOA can quickly transfer h+ and be used as TEOA to TEOA+ (Xing et al., 2014; Zhou et al., 2019).
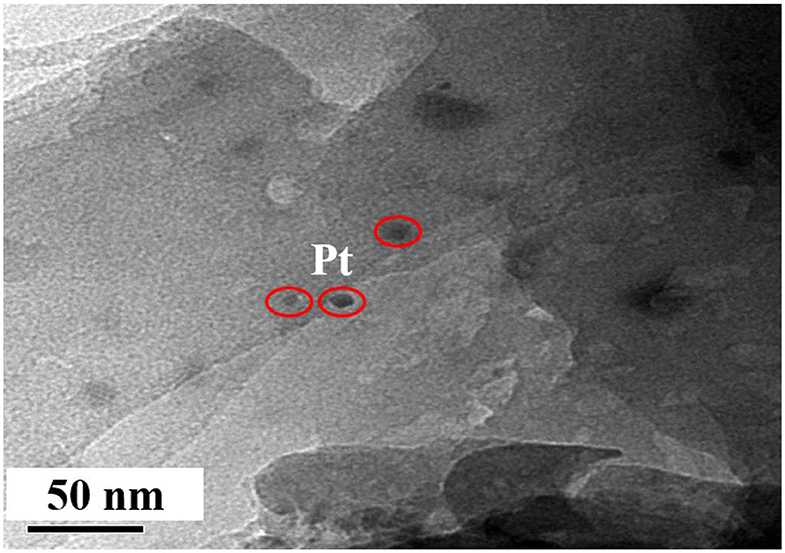
Figure 13. The TEM image of mTHPC/pCN collected after conducting photocatalytic reaction for one time.
When mTHPC/pCN is irradiated under NIR light, mTHPC has an up-conversion behavior (Figure 11); i.e., the irradiation of mTHPC under NIR light can generate VIS light for pCN to work. Actually, the ESR characterization showed the concentration of unpaired electrons is much higher in mTHPC/pCN than bCN under NIR light irradiation (Figure 10). In addition, although under NIR light irradiation only, mTHPC/pCN still works because of its NIR absorption capacity (Figure 5A) and the up-conversion behavior of mTHPC (Figure 11).
Conclusions
mTHPC/pCN prepared by positively charged pCN was modified by negatively charged mTHPC for the first time. mTHPC/pCN can allow for efficient charge separation and transfer and retard the recombination of photogenerated electrons and holes. In addition, mTHPC/pCN has a wide range of VIS light and NIR light absorption capabilities and thus the enhanced photocatalytic hydrogen evolution performance and good stability. The current results show that using a photosensitizer can enhance the light absorption intensity of the VIS light–driven g-C3N4 system and expand the absorption and utilization of the solar spectrum range. This work provides some new insights and directions for the realization of photocatalytic hydrogen evolution under VIS/NIR light region.
Data Availability Statement
The raw data supporting the conclusions of this article will be made available by the authors, without undue reservation.
Author Contributions
YL came up with the idea, designed the experiments, analyzed the data, and wrote the paper. ZM guided the research and revised the paper. Both authors contributed to the article and approved the submitted version.
Conflict of Interest
The authors declare that the research was conducted in the absence of any commercial or financial relationships that could be construed as a potential conflict of interest.
Supplementary Material
The Supplementary Material for this article can be found online at: https://www.frontiersin.org/articles/10.3389/fchem.2020.605343/full#supplementary-material
References
Adhikari, S. P., Hood, Z. D., Wang, H., Peng, R., Krall, A., Li, H., et al. (2017). Enhanced visible light photocatalytic water reduction from a g-C3N4/SrTa2O6 heterojunction. Appl. Catal. B Environ. 217, 448–458. doi: 10.1016/j.apcatb.2017.05.092
An, X. H., Cao, Y. Q., Liu, Q., Chen, L., Lin, Z. G., Zhou, Y. G., et al. (2017). Graphitic carbon/carbon nitride hybrid as metal-free photocatalyst for enhancing hydrogen evolution. Appl. Catal. A Gen. 546, 30–35. doi: 10.1016/j.apcata.2017.07.046
Boeuf-Muraille, G., Rigaux, G., Callewaert, M., Zambrano, N., Van Gulick, L., Roullin, V. G., et al. (2019). Evaluation of mTHPC-loaded PLGA nanoparticles for in vitro photodynamic therapy on C6 glioma cell line. Photodiagn. Photodyn. Ther. 25, 448–455. doi: 10.1016/j.pdpdt.2019.01.026
Bresolin, B. M., Sgarbossa, P., Bahnemann, D. W., and Sillanpää, M. (2020). Cs3Bi2I9/g-C3N4 as a new binary photocatalyst for efficient visible-light photocatalytic processes. Sep. Purif. Technol. 251:117320. doi: 10.1016/j.seppur.2020.117320
Caux, M., Menard, H., AlSalik, Y. M., Irvine, J. T. S., and Idriss, H. (2019). Photocatalytic hydrogen production over Au/g-C3N4, effect of gold particle dispersion and morphology. Phys. Chem. Chem. Phys. 21, 15974–15987. doi: 10.1039/C9CP02241D
Che, H. N., Che, G. B., Dong, H. J., Hu, W., Hu, H., Liu, C. B., et al. (2018). Fabrication of Z-scheme Bi3O4Cl/g-C3N4 2D/2D heterojunctions with enhanced interfacial charge separation and photocatalytic degradation various organic pollutants activity. Appl. Surf. Sci. 55, 705–716. doi: 10.1016/j.apsusc.2018.06.038
Chen, Y. J., Ji, S. F., Sun, W. M., Lei, Y. P., Wang, Q. C., Li, A., et al. (2020). Engineering the atomic interface with single Platinum atoms for enhanced photocatalytic hydrogen production. Angew Chem. Int. Ed. 59, 1295–1301. doi: 10.1002/anie.201912439
Cui, L. F., Liu, Y. F., Fang, X. Y., Yin, C. C., Li, S. S., Sun, D., et al. (2018a). Scalable and clean exfoliation of graphitic carbon nitride in NaClO solution: enriched surface active sites for enhanced photocatalytic H2 evolution. Green Chem. 20, 1354–1361. doi: 10.1039/C7GC03704J
Cui, L. F., Liu, Y. F., Wang, Y. T., Fang, X. Y., Yin, C. C., Kang, S. F., et al. (2018b). Constructing ultrathin g-C3N4 nanosheets with hierarchical pores by NaClO induced wet etching for efficient photocatalytic Cr(VI) detoxification under visible light irradiation. Diam. Relat. Mater. 88, 51–59. doi: 10.1016/j.diamond.2018.06.016
Da Silva, E. S., Moura, N. M. M., Neves, M. G. P. M. S., Coutinho, A., Prieto, M., Silva, C. G., et al. (2018). Novel hybrids of graphitic carbon nitride sensitized with free-base meso-tetrakis(carboxyphenyl) porphyrins for efficient visible light photocatalytic hydrogen production. Appl. Catal. B Environ. 221, 56–69. doi: 10.1016/j.apcatb.2017.08.079
Ding, F., Yang, D., Tong, Z. W., Nan, Y. H., Wang, Y. J., Zou, X. Y., et al. (2017). Graphitic carbon nitride-based nanocomposites as visible-light driven photocatalysts for environmental purification. Environ. Sci. Nano 4, 1455–1469. doi: 10.1039/C7EN00255F
Fan, M. S., Song, C. J., Chen, T. J., Yan, X., Xu, D. B., Gu, W., et al. (2016). Visible-light-drived high photocatalytic activities of Cu/g-C3N4 photocatalysts for hydrogen production. RSC Adv. 6, 34633–34640. doi: 10.1039/C5RA27755H
Fang, J. W., Fan, H. Q., Li, M. M., and Long, C. Nitrogen self-doped graphitic carbon nitride as efficient visible light photocatalyst for hydrogen evolution. J. Mater. Chem. A. (2015) 3, 13819–13826. doi: 10.1039/C5TA02257F
Fu, J. W., Xu, Q. L., Low, J. X., Jiang, C. J., and Yu, J. G. (2019). Ultrathin 2D/2D WO3/g-C3N4 step-scheme H2 production photocatalyst. Appl. Catal. B Environ. 243, 556–565. doi: 10.1016/j.apcatb.2018.11.011
Gao, X. X., Yang, B. Z., Yao, W. Q., Wang, Y. J., Zong, R. L., Wang, J., et al. (2020). Enhanced photocatalytic activity of ZnO/g-C3N4 composites by regulating stacked thickness of g-C3N4 nanosheets. Environ. Pollut. 257:113577. doi: 10.1016/j.envpol.2019.113577
Ge, L., Han, C. C., Liu, J., and Li, Y. F. (2011). Enhanced visible light photocatalytic activity of novel polymeric g-C3N4 loaded with Ag nanoparticles. Appl. Catal. A Gen. 409, 215–222. doi: 10.1016/j.apcata.2011.10.006
Haimov, E., Weitman, H., Polani, S., Schori, H., Zitoun, D., and Shefi, O. (2018). meso-Tetrahydroxyphenylchlorin-conjugated gold nanoparticles as a tool to improve photodynamic therapy. ACS Appl. Mater. Inter. 10, 2319–2327. doi: 10.1021/acsami.7b16455
Hang, N. T., Zhang, S. L., and Yang, W. (2017). Efficient exfoliation of g-C3N4 and NO2 sensing behavior of graphene/g-C3N4 nanocomposite. Sensor Actuat. B Chem. 248, 940–948. doi: 10.1016/j.snb.2017.01.199
He, R. F., Liang, H. O., Li, C. P., and Bai, J. (2020). Enhanced photocatalytic hydrogen production over Co3O4@g-C3N4 p-n junction adhering on one-dimensional carbon fiber. Colloid. Surf. A 586:124200. doi: 10.1016/j.colsurfa.2019.124200
He, Y. Q., Huang, Z. R., Ma, Z. Y., Yao, B. H., Liu, H. Y., Hu, L. L., et al. (2019). Highly efficient photocatalytic performance and mechanism of α-ZnTcPc/g-C3N4 composites for methylene blue and tetracycline degradation under visible light irradiation. Appl. Surf. Sci. 498:143834. doi: 10.1016/j.apsusc.2019.143834
Hinger, D., Navarro, F., Kach, A., Thomann, J. S., Mittler, F., Couffin, A. C., et al. (2016). Photoinduced effects of m-tetrahydroxyphenylchlorin loaded lipid nanoemulsions on multicellular tumor spheroids. J. Nanobiotechnol. 14, 68–81. doi: 10.1186/s12951-016-0221-x
Inagaki, M., Tsumura, T., Kinumoto, T., and Toyoda, M. (2019). Graphitic carbon nitrides (g-C3N4) with comparative discussion to carbon materials. Carbon 141, 580–607. doi: 10.1016/j.carbon.2018.09.082
Jia, J., Sun, W. J., Zhang, Q. Q., Zhang, X. Z., Hu, X. Y., Liu, E. Z., et al. (2020). Inter-plane heterojunctions within 2D/2D FeSe2/g-C3N4 nanosheet semiconductors for photocatalytic hydrogen generation. Appl. Catal. B Environ. 261:118249. doi: 10.1016/j.apcatb.2019.118249
Jiang, L. B., Yuan, X. Z., Pan, Y., Liang, J., Zeng, G. M., Wu, Z. B., et al. (2017). Doping of graphitic carbon nitride for photocatalysis: a reveiw. Appl. Catal. B Environ. 217, 388–406. doi: 10.1016/j.apcatb.2017.06.003
Lan, Z. A., Zhang, G., and Wang, X. (2016). A facile synthesis of Br-modified g-C3N4 semiconductors for photoredox water splitting. Appl. Catal. B Environ. 192, 116–125. doi: 10.1016/j.apcatb.2016.03.062
Lee, A. H., Wang, Y. C., and Chen, C. C. (2019). Composite photocatalyst, tetragonal lead bismuth oxyiodide/bismuth oxyiodide/graphitic carbon nitride: Synthesis, characterization, photocatalytic activity. J. Colloid Interf. Sci. 533, 319–332. doi: 10.1016/j.jcis.2018.08.008
Li, G. L., Guo, C. S., Yan, M., and Liu, S. Q. (2016). CsxWO3 nanorods: realization of full-spectrum-responsive photocatalytic activities from UV, visible to near-infrared region. Appl. Catal. B Environ. 183, 142–148. doi: 10.1016/j.apcatb.2015.10.039
Li, H. J., Qian, D. J., and Chen, M. (2015). Templateless infrared heating process for fabricating carbon nitride nanorods with efficient photocatalytic H2 evolution. ACS Appl. Mater. Inter. 7, 25162–25170. doi: 10.1021/acsami.5b06627
Li, J. Y., Cui, W., Sun, Y. J., Chu, Y. H., Cen, W. L., and Dong, F. (2017). Directional electron delivery via a vertical channel between g-C3N4 layers promotes photocatalytic efficiency. J. Mater. Chem. A 5, 9358–9364. doi: 10.1039/C7TA02183F
Li, L. L., Bodedla, G. B., Liu, Z. T., and Zhu, X. J. (2020). Naphthalimide-porphyrin hybridized graphitic carbon nitride for enhanced photocatalytic hydrogen production. Appl. Surf. Sci. 499:143755. doi: 10.1016/j.apsusc.2019.143755
Li, S. J., Chen, J. L., Hu, S. W., Wang, H. L., Jiang, W., and Chen, X. B. (2020). Facile construction of novel Bi2WO6/Ta3N5 Z-scheme heterojunction nanofibers for efficient degradation of harmful pharmaceutical pollutants. Chem. Eng. J. 402:126165. doi: 10.1016/j.cej.2020.126165
Li, S. J., Hu, S. W., Jiang, W., Liu, Y., Zhou, Y. T., Liu, J. S., et al. (2018). Facile synthesis of cerium oxide nanoparticles decorated flower-like bismuth molybdate for enhanced photocatalytic activity toward organic pollutant degradation. J. Colloid Interf. Sci. 530, 171–178. doi: 10.1016/j.jcis.2018.06.084
Li, S. J., Hu, S. W., Jiang, W., Zhang, J. L., Xu, K. B., and Wang, Z. H. (2019). In situ construction of WO3 nanoparticles decorated Bi2MoO6 microspheres for boosting photocatalytic degradation of refractory pollutants. J. Colloid Interf. Sci. 556, 335–344. doi: 10.1016/j.jcis.2019.08.077
Li, S. J., Xue, B., Chen, J. L., Liu, Y. P., Zhang, J. L., Wang, H. W., et al. (2021). Constructing a plasmonic p-n heterojunction photocatalyst of 3D Ag/Ag6Si2O7/Bi2MoO6 for efficiently removing broad-spectrum antibiotics. Sep. Purif. Technol. 254:117579. doi: 10.1016/j.seppur.2020.117579
Liang, Q. H., Li, Z., Huang, Z. H., Kang, F. Y., and Yang, Q. H. (2015). Holey graphitic carbon nitride nanosheets with carbon vacancies for highly improved photocatalytic hydrogen production. Adv. Funct. Mater. 25, 6885–6892. doi: 10.1002/adfm.201503221
Liu, C. Y., Huang, H. W., Ye, L. Q., Yu, S. X., Tian, N., Du, X., et al. (2017). Itermediate-mediated strategy to horn-like hollow mesoporous ultrathin g-C3N4 tube with spatial anisotropic charge separation for superior photocatalytic H2 evolution. Nano Energy 41, 738–748. doi: 10.1016/j.nanoen.2017.10.031
Liu, G., Yan, S., Shi, L., and Yao, L. Z. (2019). The improvement of photocatalysis H2 evolution over g-C3N4 with Na and cyano-group co-modification. Front. Chem. 7, 639–645. doi: 10.3389/fchem.2019.00639
Liu, L., Luo, X., Li, Y. Z., Xu, F., Gao, Z. B., Zhang, X. N., et al. (2018). Facile synthesis of few-layer g-C3N4/ZnO composite photocatalyst for enhancing visible light photocatalytic performance of pollutants removal. Colloid. Surf. A 537, 516–523. doi: 10.1016/j.colsurfa.2017.09.051
Liu, M. J., Wageh, S., Al-Ghamdi, A. A., Xia, P. F., Cheng, B., Zhang, L. Y., et al. (2019). Quenching induced hierarchical 3D porous g-C3N4 with enhanced photocatalytic CO2 reduction activity. Chem. Commun. 55, 14023–14026. doi: 10.1039/C9CC07647F
Liu, Q., Chen, T. X., Guo, Y. R., Zhang, Z. G., and Fang, X. M. (2016). Ultrathin g-C3N4 nanosheets coupled with carbon nanodots as 2D/0D composites for efficient photocatalytic H2 evolution. Appl. Catal. B Environ. 193, 248–258. doi: 10.1016/j.apcatb.2016.04.034
Liu, Q. W., Wang, J. M., Liu, D., Li, R. J., and Peng, T. Y. (2018). Photosensitization of zinc phthalocyanine bearing 15-crown-5 ether moieties on carbon nitride for H2 production: Effect of co-existing alkali metal ions. J. Power Sources 396, 57–63. doi: 10.1016/j.jpowsour.2018.05.098
Liu, S. Z., Sun, H. Q., Ang, H. M., Tade, M. O., and Wang, S. (2016). Integrated oxygen-doping and dye sensitization of graphitic carbon nitride for enhanced visible light photodegradation. J Colloid Interf. Sci. 476, 193–199. doi: 10.1016/j.jcis.2016.05.026
Liu, X. C., Liu, L., Yao, Z. R., Yang, Z. H., and Xu, H. (2020). Enhanced visible-light-driven photocatalytic hydrogen evolution and NO photo-oxidation capacity of ZnO/g-C3N4 with N dopant. Colloid. Surf. A 599:124869. doi: 10.1016/j.colsurfa.2020.124869
Liu, Y. F., He, M. F., Guo, R., Fang, Z. R., Kang, S. F. Z., et al. (2020a). Ultrastable metal-free near-infrared-driven photocatalysts for H2 production based on protonated 2D g-C3N4 sensitized with Chlorin e6. Appl. Catal. B Environ. 260:118137. doi: 10.1016/j.apcatb.2019.118137
Liu, Y. F., Kang, S. F., Cui, L. F., and Ma, Z. (2020b). Boosting near-infrared-driven photocatalytic H2 evolution using protoporphyrin-sensitized g-C3N4. J. Photochem. Photobiol. A 396:112517. doi: 10.1016/j.jphotochem.2020.112517
Liu, Y. Z., Zhang, H. Y., Ke, J., Zhang, J. Q., Tian, W. J., Xu, X. Y., et al. (2018). 0D (MoS2)/2D (g-C3N4) heterojunctions in Z-scheme for enhanced photocatalytic and electrochemical hydrogen evolution. Appl. Catal. B Environ. 228, 64–74. doi: 10.1016/j.apcatb.2018.01.067
Luo, B., Song, R., Geng, J. F., Jing, D. W., and Zhang, Y. Z. (2018). Facile preparation with high yield of a 3D porous graphitic carbon nitride for dramatically enhanced photocatalytic H2 evolution under visible light. Appl. Catal. B Environ. 238, 294–301. doi: 10.1016/j.apcatb.2018.07.039
Mamba, G., and Mishra, A. K. (2016). Graphitic carbon nitride (g-C3N4) nanocomposites: a new and exciting generation of visible light driven photocatalysts for environmental pollution remediation. Appl. Catal. B Environ. 198, 347–377. doi: 10.1016/j.apcatb.2016.05.052
Marangon, I., Ménard-Moyon, C., Silva, A. K. A., Bianco, A., Luciani, N., and Gazeau, F. (2016). Synergic mechanisms of photothermal and photodynamic therapies mediated by photosensitizer/carbon nanotube complexes. Carbon 97, 110–123. doi: 10.1016/j.carbon.2015.08.023
Mishra, A., Mehta, A., Basu, S., Shetti, N. P., Reddy, K. R., and Aminabhavi, T. M. (2019). Graphitic carbon nitride (g–C3N4)–based metal-free photocatalysts for water splitting: a review. Carbon 149, 693–721. doi: 10.1016/j.carbon.2019.04.104
Murugesan, P., Moses, J. A., and Anandharamakrishnan, C. (2019). Photocatalytic disinfection efficiency of 2D structure graphitic carbon nitride-based nanocomposites: a review. J. Mater. Sci. 54, 12206–12235. doi: 10.1007/s10853-019-03695-2
Naseri, A., Samadi, M., Pourjavadi, A., Moshfegh, A. Z., and Ramakrishna, S. (2017). Graphitic carbon nitride (g-C3N4)-based photocatalysts for solar hydrogen generation: recent advances and future development directions. J. Mater. Chem. A 5, 23406–23433. doi: 10.1039/C7TA05131J
Navarro, F. P., Creusat, G., Frochot, C., Moussaron, A., Verhille, M., Vanderesse, R., et al. (2014). Preparation and characterization of mTHPC-loaded solid lipid nanoparticles for photodynamic therapy. J. Photochem. Photobiol. B. Biol. 130, 161–169. doi: 10.1016/j.jphotobiol.2013.11.007
Ong, W. J., Putri, L. K., Tan, Y. C., Tan, L. L., Li, N., Ng, Y. H., et al. (2017). Unravelling charge carrier dynamics in protonated g-C3N4 interfaced with carbon nanodots as co-catalysts toward enhanced photocatalytic CO2 reduction: a combined experimental and first-principles DFT study. Nano Res. 10, 1673–1696. doi: 10.1007/s12274-016-1391-4
Ouedraogo, S., Chouchene, B., Desmarets, C., Gries, T., Balan, L., Fournet, R., et al. (2018). Copper octacarboxyphthalocyanine as sensitizer of graphitic carbon nitride for efficient dye degradation under visible light irradiation. Appl. Catal. A Gen. 563, 127–136. doi: 10.1016/j.apcata.2018.06.036
Pan, Z. M., Zheng, Y., Guo, F. S., Niu, P. P., and Wang, X. C. (2017). Decorating CoP and Pt nanoparticles on graphitic carbon nitride nanosheets to promote overall water splitting by conjugated polymers. ChemSusChem 10, 87–90. doi: 10.1002/cssc.201600850
Panneri, S., Ganguly, P., Nair, B. N., Mohamed, A. A. P., Warrier, K. G. K., and Hareesh, U.N.S. (2017). Role of precursors on the photophysical properties of carbon nitride and its application for antibiotic degradation. Environ. Sci. Pollut. Control. Ser. 24, 8609–8618. doi: 10.1007/s11356-017-8538-z
Prabavathi, S., and Muthuraj, V. (2019). Superior visible light driven photocatalytic degradation of fluoroquinolone drug norfloxacin over novel NiWO4 nanorods anchored on g-C3N4 nanosheets. Colloid. Surf. A 567, 43–54. doi: 10.1016/j.colsurfa.2019.01.040
Qi, K. Z., Li, Y., Xie, Y. B., Liu, S. Y., Zheng, K., and Chen, Z., et al. (2019) Ag loading enhanced photocatalytic activity of g-C3N4 porous nanosheets for decomposition of organic pollutants. Front. Chem. 7, 91–100. doi: 10.3389/fchem.2019.00091
Qin, J. Y., and Zeng, H. P. (2017). Photocatalysts fabricated by depositing plasmonic Ag nanoparticles on carbon quantum dots/graphitic carbon nitride for broad spectrum photocatalytic hydrogen generation. Appl. Catal. B Environ. 209, 161–173. doi: 10.1016/j.apcatb.2017.03.005
Qiu, L. F., Qiu, X. B., Li, P., Ma, M. F., Chen, X. S., and Duo, S. W. (2020). In-situ self-assembly synthesis of 2D/2D CdS/g-C3N4 heterojunction for efficient visible-light photocatalytic performance. Mater. Lett. 268:127566. doi: 10.1016/j.matlet.2020.127566
Qiu, P. X., Xu, C. M., Chen, H. Q., Jiang, F., Wang, X., Lu, R. F., et al. (2017). One step synthesis of oxygen doped porous graphitic carbon nitride with remarkable improvement of photo-oxidation activity: role of oxygen on visible light photocatalytic activity. Appl. Catal. B Environ. 206, 319–327. doi: 10.1016/j.apcatb.2017.01.058
Ran, J., Ma, T. Y., Gao, G., Du, X. W. and S. Z, Qiao, C. B. (2015). Porous P-doped graphitic carbon nitride nanosheets for synergistically enhanced visible-light photocatalytic H2 production. Energy Environ. Sci. 8, 3708–3717. doi: 10.1039/C5EE02650D
She, X. J., Liu, L., Ji, H. Y., Mo, Z., Li, Y. P., Huang, L. Y., et al. (2016). Template-free synthesis of 2D porous ultrathin nonmetal-doped g-C3N4 nanosheets with highly efficient photocatalytic H2 evolution from water under visible light. Appl. Catal. B Environ. 187, 144–153. doi: 10.1016/j.apcatb.2015.12.046
Shen, X. F., Yang, J. Y., Zheng, T., Wang, Q., Zhuang, H. F., Zheng, R. N., et al. (2020a). Plasmonic p-n heterojunction of Ag/Ag2S/Ag2MoO4 with enhanced Vis-NIR photocatalytic activity for purifying wastewater. Sep. Purif. Technol. 251:117347. doi: 10.1016/j.seppur.2020.117347
Shen, X. F., Zhang, T. Y., Xu, P. F., Zhang, L. S., Liu, J. S., and Chen, Z. G. (2017). Growth of C3N4 nanosheets on carbon-fiber cloth as flexible and macroscale filter-membrane-shaped photocatalyst for degrading the flowing wastewater. Appl. Catal. B Environ. 219, 425–431. doi: 10.1016/j.apcatb.2017.07.059
Shen, X. F., Zheng, T., Yang, J. Y., Shi, Z., Xue, Q. Q., Liu, W. P., et al. (2020b). Removal of Cr(VI) from acid wastewater by BC/ZnFe2O4 magnetic nanocomposite via the synergy of absorption-photocatalysis. ChemCatChem 12, 4121–4131. doi: 10.1002/cctc.202000619
Shi, W. L., Li, M. Y., Huang, X. L., Ren, H. J., Yan, C., and Guo, F. (2020). Facile synthesis of 2D/2D Co3(PO4)2/g-C3N4 heterojunction for highly photocatalytic overall water splitting under visible light. Chem. Eng. J. 382:122960. doi: 10.1016/j.cej.2019.122960
Shiraishi, Y., Kofuji, Y., Kanazawa, S., Sakamoto, H., Ichikawa, S., Tanaka, S., et al. (2014). Platinum nanoparticles strongly associated with graphitic carbon nitride as efficient co-catalysts for photocatalytic hydrogen evolution under visible light. Chem. Commun. 50, 15255–15258. doi: 10.1039/C4CC06960A
Sui, Y., Liu, J. H., Zhang, Y. W., Tian, X. K., and Chen, W. (2013). Dispersed conductive polymer nanoparticles on graphitic carbon nitride for enhanced solar-driven hydrogen evolution from pure water. Nanoscale 5, 9150–9155. doi: 10.1039/c3nr02413j
Sun, S. D., and Liang, S. H. (2017). Recent advances in functional mesoporous graphitic carbon nitride (mpg-C3N4) polymers. Nanoscale 9, 10544–10578. doi: 10.1039/C7NR03656F
Sun, X. Y., Zhang, F. J., Kong, C. (2020). Porous g-C3N4/WO3 photocatalyst prepared by simple calcination for efficient hydrogen generation under visible light. Colloid. Surf. A. 594:124653. doi: 10.1016/j.colsurfa.2020.124653
Tao, W., Wang, M. K., Ali, R., Nie, S., Zeng, Q. L., Yang, R. Q., et al. (2019). Multi-layered porous hierarchical TiO2/g-C3N4 hybrid coating for enhanced visible light photocatalysis. Appl. Surf. Sci. 495:143435. doi: 10.1016/j.apsusc.2019.07.177
Teng, Z. Y., Lv, H. Y., Wang, C. Y., Xue, H. G., Pang, H., and Wang, G. X. (2017). Bandgap engineering of ultrathin graphene-like carbon nitride nanosheets with controllable oxygenous functionalization. Carbon 113, 63–75. doi: 10.1016/j.carbon.2016.11.030
Tian, N., Zhang, Y. H., Li, X. W., Xiao, K., Du, X., Dong, F., et al. (2017). Precursor-reforming protocol to 3D mesoporous g-C3N4 established by ultrathin self-doped nanosheets for superior hydrogen evolution. Nano Energy 38, 72–81. doi: 10.1016/j.nanoen.2017.05.038
Tian, S. F., Chen, S. D., Ren, X. T., Cao, R. H., Hu, H. Y., and Bai, F. (2019). Bottom-up fabrication of graphitic carbon nitride nanosheets modified with porphyrin via covalent bonding for photocatalytic H2 evolution. Nano Res. 12, 3109–3115. doi: 10.1007/s12274-019-2562-x
Wang, D. H., Pan, J. N., Li, H. H., Liu, J. J., Wang, Y. B., Kang, L. T., et al. (2016). A pure organic heterostructure of μ-oxo dimeric iron(III) porphyrin and graphitic-C3N4 for solar H2 roduction from water. J Mater Chem A 4, 290–296. doi: 10.1039/C5TA07278F
Wang, F. L., Wang, Y. F., Feng, Y. P., Zeng, Y. Q., Xie, Z. J., Zhang, Q. X., et al. (2018). Novel ternary photocatalyst of single atom-dispersed silver and carbon quantum dots co-loaded with ultrathin g-C3N4 for broad spectrum photocatalytic degradation of naproxen. Appl. Catal. B Environ. 221, 510–520. doi: 10.1016/j.apcatb.2017.09.055
Wang, K., Li, Y., Li, J., and Zhang, G. K. (2020). Boosting interfacial charge separation of Ba5Nb4O15/g-C3N4 photocatalysts by 2D/2D nanojunction towards efficient visible-light driven H2 generation. Appl. Catal. B Environ. 263:117730. doi: 10.1016/j.apcatb.2019.05.032
Wang, N., Han, B., Wen, J. B., Liu, M. W., and Li, X. J. (2019). Synthesis of novel Mn-doped Fe2O3 nanocube supported g-C3N4 photocatalyst for overall visible-light driven water splitting. Colloid. Surf. A 567, 313–318. doi: 10.1016/j.colsurfa.2019.01.053
Wang, X. C., Maeda, K., Thomas, A., Takanabe, K., Xin, G., Carlsson, J. M., et al. (2009). A metal-free polymeric photocatalyst for hydrogen production from water under visible light. Nat. Mater. 8, 76–80. doi: 10.1038/nmat2317
Wang, Z. Y., Huang, Y., Ho, W. K., Cao, J. J., Shen, Z. X., and Lee, S. C. (2016). Fabrication of Bi2O2CO3/g-C3N4 heterojunctions for efficiently photocatalytic NO in air removal: in-situ self-sacrificial synthesis, characterizations and mechanistic study. Appl. Catal. B Environ. 199, 123–133. doi: 10.1016/j.apcatb.2016.06.027
Wei, F., Liu, Y., Zhao, H., Ren, X., Liu, J., Hasan, T., et al. (2018). Oxygen self-doped g-C3N4 with tunable electronic band structure for unprecedentedly enhanced photocatalytic performance. Nanoscale 10, 4515–4522. doi: 10.1039/C7NR09660G
Wennink, J. W. H., Liu, Y. N., Makinen, P. I., Setaro, F., Escosura, A., Bourajjaj, M., et al. (2017). Macrophage selective photodynamic therapy by meta-tetra(hydroxyphenyl)chlorin loaded polymeric micelles: a possible treatment for cardiovascular diseases. Eur. J. Pharm. Sci. 107, 112–125. doi: 10.1016/j.ejps.2017.06.038
Xiao, X., Wang, Y. H., Bo, Q., Xu, X. Y., and Zhang, D. G. (2020). One-step preparation of sulfur-doped porous g-C3N4 for enhanced visible light photocatalytic performance. Dalton Trans. 49, 8041–8050. doi: 10.1039/D0DT00299B
Xie, L. F., Ni, J., Tang, B., He, G. Y., and Chen, H. Q. (2018). A self-assembled 2D/2D-type protonated carbon nitride-modified graphene oxide nanocomposite with improved photocatalytic activity. Appl. Surf. Sci. 434, 456–463. doi: 10.1016/j.apsusc.2017.10.193
Xing, Z., Chen, Z. G., Zong, X., and L., and Wang, Z. (2014). A new type of carbon nitride-based polymer composite for enhanced photocatalytic hydrogen production. Chem. Commun. 50, 6762–6764. doi: 10.1039/C4CC00397G
Yang, Y. J., Sun, B. W., Qian, D. J., and Chen, M. (2019). Fabrication of multiporphyrin@g-C3N4 nanocomposites via Pd(II)-directed layer-by-layer assembly for enhanced visible-light photocatalytic activity. Appl. Surf. Sci 478, 1027–1036. doi: 10.1016/j.apsusc.2019.02.028
Ye, R. Q., Fang, H. B., Zheng, Y. Z., Li, N., Wang, Y., and Tao, X. (2016). Fabrication of CoTiO3/g-C3N4 hybrid photocatalysts with enhanced H2 evolution: Z-scheme photocatalytic mechanism insight. ACS Appl. Mater. Interfaces 8, 13879–13889. doi: 10.1021/acsami.6b01850
Yu, H. J., Shi, R., Zhao, Y. X., Bian, T., Zhao, Y. F., Zhou, C., et al. (2017). Alkali-assisted synthesis of nitrogen deficient graphitic carbon nitride with tunable band structures for efficient visible-light-driven hydrogen evolution. Adv. Mater. 29:1605148. doi: 10.1002/adma.201605148
Yuan, Y. J., Lu, H. W., Ji, Z. G., Zhong, J. S., Ding, M. Y., Chen, D. Q., et al. (2015a). Enhanced visible-light-induced hydrogen evolution from water in a noble-metal-free system catalyzed by ZnTCPP-MoS2/TiO2 assembly. Chem. Eng. J 275, 8–16. doi: 10.1016/j.cej.2015.04.015
Yuan, Y. J., Shen, Z. K., Wang, P., Li, Z. J., Pei, L., Zhong, J. S., et al. (2020). Metal-free broad-spectrum PTCDA/g-C3N4 Z-scheme photocatalysts for enhanced photocatalytic water oxidation. Appl. Catal. B Environ. 260:118179. doi: 10.1016/j.apcatb.2019.118179
Yuan, Y. J., Tu, J. R., Ye, Z. J., Lu, H. W., Ji, Z. G., Hu, B., et al. (2015b). Visible-light-driven hydrogen production from water in a noble-metal-free system catalyzed by zinc porphyrin sensitized MoS2/ZnO. Dyes Pigments 123, 285–292. doi: 10.1016/j.dyepig.2015.08.014
Zada, A., Khan, M., Qureshi, M. N., Liu, S. Y., and Wang, R. D. (2019). Accelerating photocatalytic hydrogen production and pollutant degradation by functionalizing g-C3N4 with SnO2. Front. Chem. 7, 941–949. doi: 10.3389/fchem.2019.00941
Zeng, P., Wang, J. M., Guo, Y. Y., Li, R. J., Mei, G. Q., and Peng, T. Y. (2019). Synthesis of an A2BC-type asymmetric zinc phthalocyanine derivative for efficient visible/near-infrared-driven H2 evolution on g-C3N4. Chem. Eng. J. 373, 651–659. doi: 10.1016/j.cej.2019.05.073
Zeng, Y. X., Liu, C. B., Wang, L. L., Zhang, S. Q., Ding, Y. B., Xu, Y. Z., et al. (2016). A three-dimensional graphitic carbon nitride belt network for enhanced visible light photocatalytic hydrogen evolution. J. Mater. Chem. A 4, 19003–19010. doi: 10.1039/C6TA07397B
Zhang, G., Lan, Z. A., and Wang, X. (2017). Surface engineering of graphitic carbon nitride polymers with cocatalysts for photocatalytic overall water splitting. Chem. Sci. 8, 5261–5274. doi: 10.1039/C7SC01747B
Zhang, J. L., and Ma, Z. (2017a). Ag6Mo10O33/g-C3N4 1D-2D hybridized heterojunction as an efficient visible-light-driven photocatalyst. Mol. Catal. 432, 285–291. doi: 10.1016/j.mcat.2017.02.024
Zhang, J. L., and Ma, Z. (2017b). Novel β-Ag2MoO4/g-C3N4 heterojunction catalysts with highly enhanced visible-light-driven photocatalytic activity. RSC Adv. 7, 2163–2171. doi: 10.1039/C6RA26352F
Zhang, J. S., Chen, X. F., Takanabe, K., Maeda, K., Domen, K., Epping, J. D., et al. (2010). Synthesis of a carbon nitride structure for visible-light catalysis by copolymerization. Angew. Chem. Int. Ed. 49, 441–444. doi: 10.1002/anie.200903886
Zhang, J. S., Chen, Y., and Wang, X. C. (2015). Two-dimensional covalent carbon nitride nanosheets: synthesis, functionalization, and applications. Energy Environ. Sci. 8, 3092–3108. doi: 10.1039/C5EE01895A
Zhang, X. H., Lin, L., Qu, D., Yang, J. G., Weng, Y. X., Wang, Z., et al. (2020). Boosting visible-light driven solar-fuel production over g-C3N4/tetra(4-carboxyphenyl)porphyrin iron(III) chloride hybrid photocatalyst via incorporation with carbon dots. Appl. Catal. B Environ. 265:118595. doi: 10.1016/j.apcatb.2020.118595
Zhang, X. H., Peng, T. Y., Yu, L. J., Li, R. J., Li, Q. Q., and Li, Z. (2015). Visible/near-infrared-light-induced H2 production over g-C3N4 co-sensitized by organic dye and zinc phthalocyanine derivative. ACS Catal. 5, 504–510. doi: 10.1021/cs5016468
Zhang, X. H., Yu, L. J., Zhuang, C. S., Peng, T. Y., Li, R. J., and Li, X. G. (2014). Highly asymmetric phthalocyanine as a sensitizer of graphitic carbon nitride for extremely efficient photocatalytic H2 production under near-infrared light. ACS Catal. 4, 162–170. doi: 10.1021/cs400863c
Zhao, Z. W., Sun, Y. J., Dong, F., Zhang, Y. X., and Zhao, H. (2015). Template synthesis of carbon self-doped g-C3N4 with enhanced visible to near-infrared absorption and photocatalytic performance. RSC Adv. 5, 39549–39556. doi: 10.1039/C5RA03433G
Zheng, Y., Lin, L. H., Wang, B., and Wang, X. C. (2015). Graphitic carbon nitride polymers toward sustainable photoredox catalysis. Angew. Chem. Int. Ed. 54, 12868–12884. doi: 10.1002/anie.201501788
Zhou, X. B., Li, Y. F., Xing, Y., Li, J. S., and Jiang, X. (2019). Effects of the preparation method of Pt/g-C3N4 photocatalysts on their efficiency for visible-light hydrogen production. Dalton Trans. 48, 15068–15073. doi: 10.1039/C9DT02938A
Keywords: g-C3N4, meso-tetrahydroxyphenylchlorin, photocatalytic, hydrogen evolution, visible/near-infrared light
Citation: Liu Y and Ma Z (2020) g-C3N4 Modified by meso-Tetrahydroxyphenylchlorin for Photocatalytic Hydrogen Evolution Under Visible/Near-Infrared Light. Front. Chem. 8:605343. doi: 10.3389/fchem.2020.605343
Received: 12 September 2020; Accepted: 06 October 2020;
Published: 06 November 2020.
Edited by:
Shijie Li, Zhejiang Ocean University, ChinaReviewed by:
Fa-tang Li, Hebei University of Science and Technology, ChinaXiaofeng Shen, Zhejiang University of Science and Technology, China
Copyright © 2020 Liu and Ma. This is an open-access article distributed under the terms of the Creative Commons Attribution License (CC BY). The use, distribution or reproduction in other forums is permitted, provided the original author(s) and the copyright owner(s) are credited and that the original publication in this journal is cited, in accordance with accepted academic practice. No use, distribution or reproduction is permitted which does not comply with these terms.
*Correspondence: Zhen Ma, zhenma@fudan.edu.cn