Nanomaterials-Mediated Immunomodulation for Cancer Therapeutics
- 1School of Biotechnology, Jawaharlal Nehru University, New Delhi, India
- 2Flowcytometry Facility, Modern Biology Department, University of Calcutta, Kolkata, India
- 3Department of Clinical Neurosciences, University of Cambridge, Cambridge, United Kingdom
- 4Clare Hall, University of Cambridge, Cambridge, United Kingdom
- 5Charles River Laboratories, Cambridge Biomedical Campus, Cambridge, United Kingdom
Immunotherapy holds great promise in overcoming the limitations of conventional regimens for cancer therapeutics. There is growing interest among researchers and clinicians to develop novel immune-strategies for cancer diagnosis and treatment with better specificity and lesser adversity. Immunomodulation-based cancer therapies are rapidly emerging as an alternative approach that employs the host’s own defense mechanisms to recognize and selectively eliminate cancerous cells. Recent advances in nanotechnology have pioneered a revolution in the field of cancer therapy. Several nanomaterials (NMs) have been utilized to surmount the challenges of conventional anti-cancer treatments like cytotoxic chemotherapy, radiation, and surgery. NMs offer a plethora of exceptional features such as a large surface area to volume ratio, effective loading, and controlled release of active drugs, tunable dimensions, and high stability. Moreover, they also possess the inherent property of interacting with living cells and altering the immune responses. However, the interaction between NMs and the immune system can give rise to unanticipated adverse reactions such as inflammation, necrosis, and hypersensitivity. Therefore, to ensure a successful and safe clinical application of immunomodulatory nanomaterials, it is imperative to acquire in-depth knowledge and a clear understanding of the complex nature of the interactions between NMs and the immune system. This review is aimed at providing an overview of the recent developments, achievements, and challenges in the application of immunomodulatory nanomaterials (iNMs) for cancer therapeutics with a focus on elucidating the mechanisms involved in the interplay between NMs and the host’s immune system.
Introduction
The emergence of cancer and its advancement are manifestations of the inadequacy of the host's immune system to recognize and execute an effective response to the tumor antigens. The cells of the host’s immune system are responsible for distinguishing and eliminating the cancer cells during the immune surveillance process (Houghton, 1994; de Visser et al., 2006; Lakshmi Narendra et al., 2013). Tumor cells escape the immunosurveillance by evading the anti-tumor measures of the immune system (Liu and Cao, 2016; Muenst et al., 2016). Tumor-derived signals within the tumor microenvironment (TME) downregulates the anti-cancer functions of the infiltrating immune cells. Moreover, they are modulated to promote tumor growth and progression (Whiteside, 2008; Lei et al., 2020). Thus, the immune system plays a paradoxical role by creating an immunosuppressive microenvironment that facilitates cancer progression (de Visser et al., 2006; Kitamura et al., 2015). Immunosuppression can be defined as the diminished ability of the body to induce an immune response due to a lack of immune cells such as T and/or B lymphocytes (Lakshmi Narendra et al., 2013) (For definitions refer Glossary). Chemotherapy is a common treatment for several types of cancers; however, this form of therapy often leads to immunosuppression due to a decrease in the population of immune cells such as T and B lymphocytes (Muenst et al., 2016), and dendritic cells (DCs) (Ferrari et al., 2003; Wertel et al., 2007). However, several chemotherapeutic agents are reported to weaken the immune system by inhibiting the production of blood cells at the bone marrow, resulting in a drastic decrease in the total cell count and impaired immunity (Peng et al., 2015; Liu and Cao, 2016; Muenst et al., 2016). Traditional strategies to combat cancer include cytotoxic chemotherapy, radiation therapy, and surgical interventions. Besides having several adverse side-effects, these approaches also lack the cardinal ability to specifically target the cancer cells while causing minimum damage to healthy cells (Chircop and Speidel, 2014; Pearce et al., 2017). Deleterious side-effects, immunosuppression, multi-drug resistance, and lack of specificity of the existing treatments have necessitated exploration into alternative therapies for cancer. Recently, there has been a paradigm shift in cancer therapeutics towards alternate immunotherapies (Kazemi et al., 2016).
Immunotherapy involves enhancing or suppressing the host’s immune system for the treatment of disease without the utilization of cytotoxic drugs. When applied for cancer treatment, the goal of cancer immunotherapy is to harness the inherent ability of the host’s immune system to eradicate cancer cells in a minimally invasive manner without causing serious side effects, unlike its conventional counterparts (Restifo et al., 2012). There is growing interest among researchers and clinicians aimed at developing novel strategies with better specificity in targeting cancer cells while instigating the least amount of adverse reactions. Recently, some of the alternate approaches to treat cancer are based on immunomodulation which employs the host's own natural defense mechanisms to recognize and selectively eliminate the cancer cells by inducing the immune system (Liu, Z. et al., 2018; Tan et al., 2017; Zhang et al., 2016). Immunomodulation is the process of altering the immune system and its responses. This readjustment of the immune system can either occur naturally, as a part of homeostatic regulation or it can be induced by medical intervention to achieve a therapeutic effect. Besides, this regulation process can be assistive or suppressive in nature to either amplify or abate the immune responses, respectively. In recent years, the technique of induced immunomodulation has gained popularity as a tool for cancer therapeutics.
Nanomaterials can be defined as a class of materials that possess at least one dimension within the range of 1–100 nm (Bleeker et al., 2013). Advances in the field of nanotechnology and material sciences have opened new avenues in the synthesis and development of various nanomaterials (NMs composed of DNA, organic/inorganic polymers, carbon, metals, liposomes, etc. for cancer therapy. The nanostructures can be fabricated in various shapes, sizes, and along with versatile surface functionalities. NMs, when introduced in vivo, are recognized by the immune cells as foreign entities therefore generate an immune response that can either be immunostimulatory or immunosuppressive (Yoshioka et al., 2017; Feng et al., 2019). While for most of the applications, these effects are undesirable, but in the case of cancer immunotherapy, the unique immunomodulatory action of NMs can be exploited to target cancer cells.
Recent advances in the field of nanomaterial fabrication and their functionalization has led to the emergence of a new class of multifunctional nanotherapeutic materials that have the potential to revolutionize the field of cancer therapy (Roy Chowdhury et al., 2016). These nanotherapeutic agents exploit several unique properties of NMs such as their ability to specifically target cancer cells, localize at the tumor site, stay in circulation for a longer time period by avoiding clearance, encapsulate and co-deliver multiple active agents, the stimuli-responsive release of cargo, et cetera (Baetke et al., 2015; Chen et al., 2017). Moreover, NM based cancer therapeutic strategies hold great promise for application of personalized medicine as targeted cancer treatments (Diou et al., 2012; Jo et al., 2016).
Interestingly, recent breakthroughs in cancer therapeutics have been for NMs such as gold nanostructures, carbon dots, and carbon nanotubes which possess the ability to cross the blood-brain barrier (BBB). This unique property of NMs creates an opportunity to deliver therapeutic and imaging agents across the BBB and has stimulated widespread research in exploiting their application in brain-cancer treatment and diagnostics (Tang et al., 2019). Additionally, attractive properties of nanoparticles such as a large surface area to volume ratio, tunable dimensions, and, high stability have also imparted beneficial features to the cancer therapeutic regimens. The ability of porous and hollow nanoparticles to load hydrophobic cancer drugs as cargo, protect them from degradation and delivery at the target site in a controlled manner, have revolutionized the designing of cancer nanomedicines (Qin et al., 2017). NMs offer a dual aspect of immunomodulation therapies. They can themselves act as immunomodulatory agents by interacting with the immune system after administration or they can merely act as delivery platforms for targeted delivery of other immunomodulating agents (Kubackova et al., 2020).
The immunomodulatory action of NMs can have positive as well as negative impacts (Figure 1). Therefore, the need of the moment is to acquire in-depth knowledge and a clear understanding of the interactions between NMs and the immune system. These insights are vital for the safe application of immunomodulatory nanomaterials (iNMs) for cancer therapeutic applications. In this review, we have focused on the immunomodulatory effects of various NMs and their application towards cancer therapy. The article highlights the role of the immune system in cancer and the interaction of NMs with the immune system to target and eliminate cancer cells either actively or passively. The recent advances in iNMs and their application in cancer therapy are also discussed with a focus on elucidating their mode of action to achieve a better understanding of the nanomaterial-immune system interactions.
Immune System and Cancer
The Immune System
Immunity can be defined as the inherent ability of an organism to avert pathogens and diseases by recognition and subsequent neutralization or elimination of foreign entities by the organism’s immune system. These protective mechanisms involve various organs, tissues, cells, and chemical mediators of the immune system and are largely based on the ability to differentiate between the “self” and “non-self”. The innate (natural) and the adaptive (acquired) immunity are the two major components of the immune system that orchestrate the overall immune responses against foreign pathogens and transformed cells. Therefore, functions of the immune system along with the immune cells acts as a defense barrier against a plethora of invading pathogens as well as cancer in order to restore and maintain homeostasis (Parkin and Cohen, 2001).
Innate immunity consists of pre-deployed molecular and cellular barriers that act against the pathogen within hours of initial contact and therefore serve as the first line of defense of the immune system. Physical barriers include skin, mucosal linings, hair, cilia, etc. that serve as the first line of defense of the immune system. When these physical barriers are breached, the protective role of the soluble mediators and the effectors of the innate system comes into play. The chemical barriers consist of chemical agents present in the antimicrobial peptides, lysozyme, gastric secretions, glandular secretions, small intestine, and blood. Various cell types such as phagocytes (e.g., macrophages, neutrophils, dendritic (DC) cells), and leukocytes (e.g., natural killer (NK) cells, mast cells, eosinophils, basophils) also participate in the innate immune responses as a second line of defense. Another important innate cellular population is that of keratinocytes which are actively involved in recognizing and responding to the pathogen-associated molecular patterns (PAMPs) present on pathogens and danger molecules such as damaged-associated molecular patterns (DAMPs) expressed by the abnormal host cells (Moser and Leo, 2010; Takeuchi and Akira, 2010). Upon recognition by the pattern recognition receptors (PRRs) and subsequent activation of the keratinocytes, cascades involving the release of various soluble mediators (e.g., cytokines, chemokines, complement proteins, collectins, etc.) and antimicrobial peptides (e.g., histatins, cathelicidins, defensins, etc.) are initiated. Therefore, soluble mediators, antimicrobial peptides, and cellular receptors (e.g. Toll-like receptors) are an integral part of the innate immunity that recognizes and responds to the chemical makeup of the pathogens (Braff and Gallo, 2006; Coates et al., 2018). The barriers and the cells of the innate system are the first to encounter and respond to an antigen. Such responses lack specificity but act as a fast and effective primary response against foreign particles (Turvey and Broide, 2010; Iwasaki and Medzhitov, 2015). Moreover, the innate immune system also plays a crucial role in activating the subsequent adaptive immune responses.
On the contrary, the adaptive immune responses are not immediate; they take several days to develop but are highly specific in neutralizing the antigen. Such responses serve as the third line of defense and are acquired by the host’s immune system after encountering an antigen. The T and B lymphocytes orchestrate the two key features of the host's adaptive immune responses; 1) The vast and specific range of T and B cell receptors generated via the process of somatic recombination, and; 2) The ability to develop immunological “memory” upon encountering pathogens leading to a more rapid and potent immune response in case of subsequent encounters (Bonilla and Oettgen, 2010). The cell-mediated adaptive immunity involves cytotoxic T cells that can recognize affected cells and induce cell death (Wing and Remington, 1977). The humoral adaptive immunity is established by helper T cells via activation of B cells which produce specific antibodies against the pathogen. The barriers of adaptive immunity include lymph nodes, lymphoid tissues, and the spleen. The random generation of a wide range of antigen receptors via somatic recombination confers the adaptive immune system with the diverse ability to recognize a plethora of pathogens. However, this diversity comes at a price and can often lead to ineffectiveness in distinguishing between the self and non-self and subsequent attack on the host’s own cells giving rise to autoimmune diseases. Consequently, the immune system has several mechanisms and checkpoints in place for recognition, regulation, and elimination of self-reactive immune cells. Another unique feature of adaptive immunity is the ability to generate custom-tailored antigen-specific T and B lymphocytes upon initial encounter with the pathogen. This ensures a potent and highly specific immune response that is effective in neutralization or elimination of the pathogen. Unfortunately, the process takes a significant period of time (5–6 days) leading to a considerable delay in the elicitation of the adaptive immune responses (Tomar and De, 2014).
In the context of the evolutionary timeline, innate immunity was developed earlier and therefore is found in all multicellular living beings. Whereas, adaptive immunity was an outcome of subsequent evolutionary processes and thus is present only in higher vertebrates (Pancer and Cooper, 2006). Therefore, rather than clear distinct systems, there exists an intertwined network of regulatory mechanisms between the two systems and the overall host defense is a result of a highly complex crosstalk and coordination between the innate and adaptive immunity (Cooper, 2016; Symowski and Voehringer, 2017). Certain cells, proteins, and receptors such as NK cells (Fehniger et al., 2003; Sun and Lanier, 2009), DCs (Palucka and Banchereau, 1999; Chan et al., 2006), cytokines (Belardelli and Ferrantini, 2002; Schön and Erpenbeck, 2018), Toll-like Receptors (TLRs) (Pasare and Medzhitov, 2004), platelets (Elzey et al., 2003; Elzey et al., 2005), are involved in this crosstalk by acting as crucial links between the innate and adaptive immune systems.
Besides acting as a defense barrier against pathogens and eliminating/neutralizing foreign antigens, the immune system also plays an important role in maintaining tolerance towards self and clearing abnormal or damaged host cells that can lead to cancer and various autoimmune disorders (Knochelmann et al., 2018). A diseased condition arises when the immune system is either 1) weak or inactive (immunodeficiency); 2) overactive (hypersensitivity); 3) loses the inherent capacity to distinguish between self and non-self which results in an immune attack towards the host itself (autoimmunity); and/or 4) the antigen/pathogen evades all the existing immune defenses of the host’s immune system leading to the manifestation of the disease (infection) (Kelly and Leonard, 2003; Martinon and Tschopp, 2004; Finlay and McFadden, 2006; Gregersen and Behrens, 2006; Valenta et al., 2009). As the functions of the immune system are impaired in such diseases, positive or negative modulation of the immune responses by activating or suppressing the immune system with the help of immunomodulatory agents serves as an effective mode of treatment. Immuno-suppressive therapies and anti-inflammatory agents are common treatments for diseases related to autoimmunity, inflammation, or hypersensitivities (Ephrem et al., 2005; Broide, 2009; Musette and Bouaziz, 2018; Immunotherapies for Autoimmune Diseases, 2019). Similarly, in the case of immune-deficiencies, upregulation of immune responses via immuno-stimulatory therapies have shown promising outcomes. Immunostimulatory treatments are also effective in treating bacterial and viral infections (Drews, 1980; Dickneite et al., 1984; Smalley Rumfield et al., 2020). Recently, studies are being conducted towards the application of immunomodulation therapies in combating severe acute respiratory syndrome coronavirus 2 (SARS-CoV-2) infection in patients suffering from coronavirus disease 2019 (COVID-19) (Hall et al., 2020; Ingraham et al., 2020; Tay et al., 2020). These therapies are aimed at enhancing the patient’s innate and adaptive immune mechanisms to overcome the pathogenesis of SARS-CoV-2. Additionally, several in vivo studies have demonstrated that an impaired immune system leads to higher incidences of clinical manifestations leading to malignant tumors suggesting the importance of immunosurveillance by the immune system in preventing cancer (Shankaran et al., 2001). Therefore, the immune system and its modulation play a critical role in oncogenesis as well as its treatment.
Role of Immune System in Cancer
Cancer cells can be categorized as altered host cells that evade the growth regulation mechanisms, override the homeostasis maintenance pathways, and undergo uncontrolled growth (Li and Neaves, 2006). The cellular components and biochemical mediators of both innate and adaptive immunity are involved in a complex crosstalk to give rise to an effective anti-tumor response consisting of processes such as immunoediting and the cancer immunity cycle (Swann and Smyth, 2007; Calì et al., 2017). Adaptive immunity is responsible for the generation of antigen-specific immune responses; hence, it plays a central role in eliciting anti-cancer responses against the cancer tumor antigens.
Anti-Cancer Measures by the Immune System
The host’s immune system coordinates a highly complex series of sequential steps to eliminate the cancer cells. These events lead to the development of effective anti-cancer responses which altogether constitute the “cancer immunity cycle” (Chen and Mellman, 2013). During the early stages of cancer development, the tumor/cancer immunity is established when tumor antigens are recognized by dendritic cells (DCs) of the host’s immune system and the antigens are processed and presented on the surface of antigen-presenting cells (APCs) via major histocompatibility complex (MHC) I or II. DC cells are a crucial link between innate and adaptive immunity and orchestrate the response to antigen by processing and presenting it to the cells of the adaptive immune system. They are responsible for priming and activating the naïve T cells located within the draining lymph nodes (DLNs) via interaction with MHC complex and co-stimulatory signals. This leads to the maturation of T cells to effector T cells that overexpress certain receptors. Regulatory T cells (Tregs) play a key role in modulation of the immune system to maintain tolerance towards self-antigens. Tregs have immunosuppressive effects as they usually downregulate the functioning of T effector cells. Studies have shown that the depletion of the Tregs population at the tumor site helps to overcome the immunosuppressive TME and significantly improves the efficacy of cancer immunotherapies (Curiel, 2007; Wang et al., 2017). Once activated, the circulating T effector (Te) cells reach the tumor site and are responsible for evoking an immune response against the cancerous cells. The cytotoxic T lymphocytes (CTLs) recognize tumor cells via MHC-I and eliminate them by releasing chemical cues such as granzyme B and perforins. The macrophages and mast cells release soluble chemical mediators such as cytokines, histamine, chemokines, and reactive oxygen species (ROS) when they encounter the tumor antigen. The chemical mediators initiate inflammation and tissue repair. The obliteration of tumor cells, in turn, releases more tumor-derived antigens that lead to the establishment of a more robust cancer-immunity. Therefore, the key players in establishing an effect on tumor immunity are macrophages, DCs, cytotoxic T lymphocytes (CTLs), and regulatory (Tregs) T cells (Chen and Mellman, 2017). Additionally, for specific and effective elimination of cancerous cells, the activity of CTLs and macrophages should be stimulated while the function of Tregs inhibited (Ruter et al., 2009).
Recognition and Elimination by the Immune System
Cancer immunoediting comprises of three phases (Dunn et al., 2004). The first is the elimination phase which involves immunosurveillance by the innate and adaptive immune systems to detect and eliminate the abnormal cells that are either malignant or have the potential to become malignant. The elimination phase involves several cell populations such as NK cells, macrophages, DCs, cytotoxic T cells, and B cells (Dunn et al., 2006). This stage marks the undetectable phase of early tumor development. The tumor cells that have escaped the initial stage of elimination enter the next phase of equilibrium where the tumor cells coexist with the host’s immune system. This is the longest phase and marks a temporary state of equilibrium between the host’s immune system and the tumor. Moreover, during this period, the tumor cells can either remain dormant or can accumulate mutations giving rise to new tumor variants that in turn increases the overall resistance of the tumor to the host’s immune mechanisms (Mittal et al., 2014). The growth and expansion of tumor cells may eventually lead to the escape phase where the cancer cells can metastasize and give rise to malignancies (Dunn et al., 2002; Smyth et al., 2006).
Escape and Evasion by the Tumor Cells
As the tumor growth progresses, some of the tumor cells may evade the immune system’s measures and escape. Most of the evasion mechanisms of the tumor cells facilitate the development of a TME that can protect the cancer cells by suppressing the host’s immune responses (Schreiber et al., 2011). Therefore, immunoediting is a continuous process involving both immunosurveillance to eliminate cancer cells and the evasion by tumor cells that leads to tumor progression. The escape of tumor cells from various stages of immunoediting is mediated by numerous immunosuppressive strategies. Some of these evading mechanisms include downregulation or absence of MHC class I expression on cancer cells preventing T cell-mediated responses, production of cytokines that induce anti-apoptotic pathways, upregulation of non-classical MHC class I expression preventing NK mediated cell killing and tumor cell-induced immunosuppression (Waldhauer and Steinle, 2008; Al-Tameemi et al., 2012; Mohme et al., 2017). Escape and evasion of tumor cells often leads to cancer malignancies, creating the need for clinical interventions in the form of various cancer therapies.
Emergence of Cancer Immunotherapies
Cancer is one of the major causes of death around the world. Figure 2 depicts the prevalence of cancer and cancer-related mortalities across major countries based on the latest Globocan 2020 report. In the year 2020 alone, cancer was responsible for approximately 9.9 million deaths globally out of which about 65% of the deaths were attributed to under-developed and developing countries (Figure 2 (inset)).
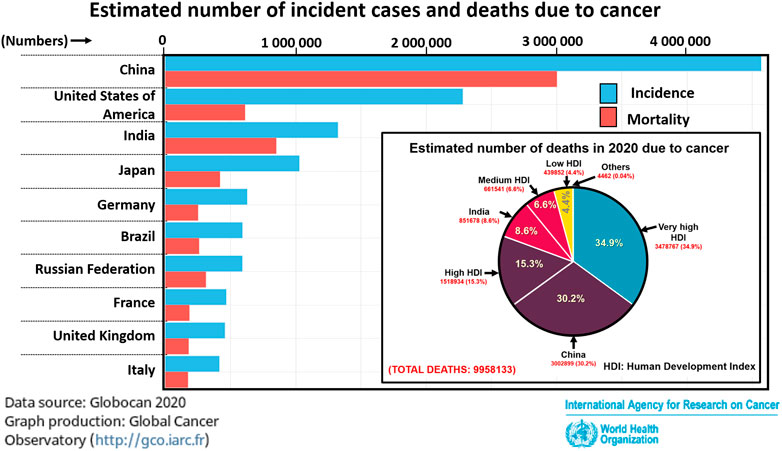
FIGURE 2. Cases of cancer prevalence and mortality in the year 2020. In inset: Estimation of mortalities due to cancer in 2020 among countries based on human development index (HDI). Statistics provided by Globocan 2020, World Health Organization.
The conventional cancer treatments are based on surgical intervention, radiation therapy, and/or chemotherapy. Even though these procedures are highly invasive and involve deleterious side-effects, they constitute a major part of the standard cancer treatment methodology (Cosentino and Piro, 2018; Pusuluri et al., 2019). These treatments are applied either individually or in combination to combat cancer and its recurrence. However, these conventional methods of treatment are associated with several limitations such as poor solubility due to the hydrophobic nature of the drug, lack of selectivity and specificity, immunosuppression, multidrug resistance, high rate of metastasis and recurrence. These limitations have encouraged the development of alternative therapies based on modulation of the immune system and its responses to better combat cancer either independently or in conjugation with the conventional treatments.
The therapeutic method based on modulating the host’s own immune system to eliminate cancer was first proposed in the early 1890s (Coley, 1991). Unfortunately, the novel technique failed to draw the attention of the oncologists due to the lack of knowledge and understanding of the underlying mechanisms of the immune system. Consequently, over the new few decades, a treatment plan consisting of conventional procedures such as surgery, radiation and/or chemotherapy prevailed as a gold standard for treating cancer.
However, simultaneously the following decades saw a steady rise in the number of studies aimed at unravelling the intrinsic relation between the immune system and cancer (Figures 3, 4). Along the path, a vast knowledge has been gathered by elucidating the role of the host’s immune cells in establishing anti-tumour responses and the evading mechanisms of the cancer cells. The in-depth understanding of the immune mechanisms and the severe limitations of conventional cancer treatments led to the revival of the immunomodulatory approach towards combating cancer (D'Errico et al., 2017; Decker and Safdar 2009).

FIGURE 3. Year-wise number of publications on 'Immune system' and 'Cancer' from the year 1945–2019. As per the data available on PubMed.
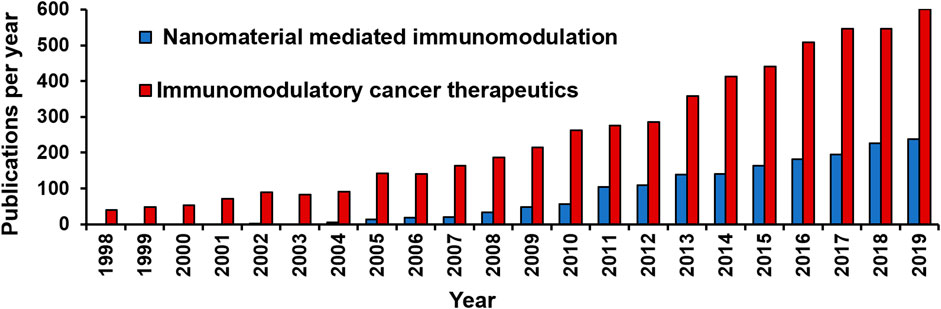
FIGURE 4. Number of publications on “Nanomaterial mediated immunomodulation” and “Immunomodulatory cancer therapeutics” indexed in PubMed from year 1998–2019.
As a result, in the early 2000s, the concept of immunotherapy was revisited as an alternative approach to treat cancer (Zacharski and Sukhatme, 2005). Unlike the traditional treatments, these therapies are based on boosting the host’s immune responses by administering cytokines, antibodies and/or various co-stimulatory signals. Therefore, they have fewer side-effects and are much more effective in eradicating primary tumours and preventing future incidences of recurrence and metastasis (Kroemer and Zitvogel, 2018). Thus, immunotherapy served as a cornerstone for a paradigm shift in the battle against cancer and has become a standard part of the cancer treatment regimen (Abbott and Ustoyev, 2019). Till today, several cancer immunotherapies have been developed based on stimulation of DCs (e.g. DC-based vaccines) (Sabado et al., 2017; Perez and De Palma, 2019), enhancing the T-cell mediated immune responses (e.g. Adoptive T-cell therapy and chimeric antigen receptor (CAR) T-cell therapy) (Met et al., 2019; Miliotou and Papadopoulou, 2018) and by blocking the inhibitory checkpoints that suppress the immune responses towards cancer (e.g. Checkpoint inhibitor therapy) (Ribas and Wolchok, 2018; Xu, W. et al., 2020). In 2018, the Nobel Prize in Physiology or Medicine was awarded to James P. Allison and Tasuku Honjo for their ground-breaking work on cancer immunotherapy via inhibition of negative regulation (Ballas, 2018). Despite the novel approach of cancer immunotherapy techniques, the extent of their clinical applications has been largely restricted due to significant limitations. For example, cancer patients undergoing checkpoint-inhibition immunotherapies are often prone to developing immune-related adverse events and autoimmune diseases (Dhodapkar, 2019; Pauken et al., 2019). Cancer immunotherapies have shown limited effectiveness in the treatment of solid tumours due to the inability of the immune cells to infiltrate the solid tumour and its microenvironment (Melero et al., 2014). Only a fraction of cancer patients responds to administered immunotherapies and the ones who respond often develop acquired resistance to these therapies (Draghi et al., 2019; Hegde and Chen, 2020). Moreover, certain tumours are characterized as “cold” due to the lack of infiltrating T cells indicating the presence of an immunosuppressive TME that discourages immune activation (Bonaventura et al., 2019). Such “cold” tumours act as a major challenge as they show poor response towards traditional cancer immunotherapies. Thus, much of cancer research has been focused on turning these “cold” tumours into “hot” tumours by modulating the TME and boosting the host’s immune system (Duan et al., 2020).
The technological advances in the field of nanotechnology hold a great promise towards the development of better-targeted cancer immunotherapies with higher specificity, stability, and sustained antitumour effects (Shukla and Steinmetz, 2016). Due to their small size, NMs can effectively infiltrate solid tumours, display retention properties, escape clearance, remain in circulation for longer periods and their surfaces can be easily functionalized to bear specific targeting moieties (Liu, Y. et al., 2018). They can also be applied as nano-delivery systems that can encapsulate various small active agents and deliver them in a sustained manner at the target site (Sarkar et al., 2013; Asadi et al., 2017). When compared to their free forms, the NM encapsulated formulations of several drugs have shown better toxicity profiles with lesser side-effects upon administration thereby significantly improving the therapeutic index of these potent drugs (Desai et al., 2006; Fukuda et al., 2017).
In recent times, several cancer immunotherapies have been aimed at targeting the stages of the cancer immunity cycle. The latest advances in this research area include the application of nanomaterials for formulating improved cancer immunotherapies to achieve enhanced tumor-targeting and neutralizing the immuno-suppressive TME (Yang and Zhang, 2020). A recent work involving the fabrication of a three-in-one nanoplatform was successful in amplifying the various phases of the cancer-immunity cycle to boost the overall immune responses against cancer antigens. The immunotherapy was based on NM-mediated delivery of an immune-agent to elicit a robust immune action along with memory responses. Therefore, it acted as a potent nano-weapon for the prevention of tumor metastasis and future relapses (Li Q. et al., 2019). Another interesting study based on liposomal co-delivery of imatinib (IMT) and siRNA-PD-L1 showed promising results towards enhancement of the cancer immunity cycle by knockdown of programmed cell death ligand 1 (PD-L1) which in turn prevented the PDL1-mediated immunosuppression of tumor-specific CTLs (Li and Han, 2020).
Nanoparticles can infiltrate the “cold” tumours allowing co-delivery of a wide variety of payloads specifically to the tumour site thus boosting the immune activation at TME. Additionally, the immunomodulatory properties of the NMs also aid in turning “cold” tumours into “hot” (Rodallec et al., 2018; Buabeid et al., 2020). For example, a recent study based on citrate-coated superparamagnetic iron oxide NPs (SPIONs) display the capacity to act as a magnetic drug delivery system for guided targeting to “cold” tumours by application of an external magnetic field (Mühlberger et al., 2019). The application of NMs in cancer immunotherapies has opened new arenas to integrate therapy and diagnosis into a single nano-therapeutic unit, leading to a paradigm shift in onco-treatments and paving way for novel developments in the field of cancer therapy and diagnosis (Tan et al., 2020). Another recent interesting study was based on the utilization of a zeolitic imidazole framework (ZIF) as a nanoplatform for pH-responsive release of cargo molecules for targeted combinational therapy to treat “cold” tumours. The nanoplatform was loaded with a photothermal agent (indocyanine green) and an immune adjuvant (imiquimod). The multifunctional nanoplatform eradicated the primary tumours in in vivo tumour models and also prevented tumour recurrence when re-challenged (Yu et al., 2020). Since the success of any immunotherapy-based cancer therapy depends largely on enhancing the inherent cancer immunity of the patient, a comprehensive understanding of the underlying mechanisms of immunomodulation is an essential pre-requisite for designing an efficient clinical strategy.
Immunomodulation as a Tool in Cancer Therapeutics
Immunomodulation is the process of altering the immune system and its responses. Such alteration can be of two types: immuno-suppression–where the immune responses are diminished or immuno-stimulation–where the immune responses are amplified (Grabowski et al., 2020; Nishiguchi and Taguchi, 2020). This fine-tuning of the immune system can either occur naturally, as a part of homeostatic regulation or it can be induced by medical interventions to achieve a therapeutic effect. Immunosuppressive therapies are usually applied in the case of auto-immune diseases (Carballido et al., 2020) and immuno-stimulation is a course of action for cancer and infectious diseases (Dittmer and Olbrich, 2003; García-Martínez et al., 2018). The recent trend is to apply nanotechnology to design alternative treatment approaches which can induce therapeutic effects at the target site while causing minimum collateral damage and adverse effects (Li, T. et al., 2020; Tan et al., 2020). Unlike conventional cancer therapies, this approach provides a more precise and personalized therapeutic effect that causes minimum side-effects and allows simultaneous imaging of the target site (monitoring) (Hapuarachchige and Artemov, 2020; Xu, C. M. et al., 2020). As a result, the technique of induced immunomodulation has gained popularity as a tool for cancer therapy (Comparetti et al., 2018; Li, J. et al., 2019; Xu, B. et al., 2020). Since these immunotherapy-based approaches towards eradicating cancer cells rely on employing the body’s own natural defense system to combat cancer they achieve targeted results with fewer side-effects than conventional cancer treatments (Sau et al., 2019).
NMs have gained a lot of attention in the field of biomedical and therapeutics due to several unique set of features offered by this class of smart materials. These properties of NMs are discussed in detail in the following Properties of Nanomaterials (NMs) Section of this article. Over recent years, several types of NMs have been investigated for their ability to react with components of the immune system and modulate its behavior. The modulation of the immune system via nanomaterials is a complex process and can have both beneficial and harmful effects (Alsaleh and Brown, 2018). Also, the nanomaterial-mediated immunomodulation can be achieved either directly- where the NMs itself causes immunomodulatory effects or indirectly- where the NMs acts as a vehicle for targeted delivery of immunomodulatory agents (IMAs) (Bartneck, 2017; Kubackova et al., 2020). Moreover, due to the small size of NMs and the leaky vasculature of tumors, the NMs undergo enhanced permeability and retention (EPR) effects that allow them to access tumors and selectively co-localize within them (Shi et al., 2020). Therefore, nanomaterial-mediated immunomodulation has the ability to execute targeted delivery of cargo molecules to the immune cells, resulting in better clinical outcomes with minimal adverse events. These unique properties have resulted in a fast-growing interest in the field of NM-mediated immunomodulation and application in cancer therapy during the past decade.
Mechanism of Immunomodulation by Nanomaterials
Properties of Nanomaterials
Over the last few decades, advancements in the field of nanotechnology and material sciences have led to a major breakthrough in the fabrication of various NMs composed of DNA (Dai et al., 2017; Jiang et al., 2017), polymers (Chang et al., 2018; Simpson et al., 2019), carbon (Teradal and Jelinek, 2017; Jiang et al., 2019), metals (Dhivya et al., 2015; Singh, 2018), composites (Song et al., 2015; Zhai et al., 2018), liposomes (Zhang et al., 2011; Lorente et al., 2018; Zhang et al., 2018), et cetera for cancer therapy. Synthesis of NMs allows the materials to be fine-tuned to tailor their properties (e.g., size, composition, surface properties, etc.) according to the targeted biological application (Wang et al., 2009). The NMs within the dimension range of 1–100 nm closely resemble the natural hierarchy and architecture of human cells, tissues, and ECM at a nanoscale. This confers NMs with the ability to interact with their surroundings, improving the effectiveness, accuracy, and efficacy of the nanomaterial-mediated therapeutic and diagnostic approaches (Li, B. et al., 2018; Tan and Saltzman, 2004). Additionally, attractive properties of NMs such as a large surface area to volume ratio, tunable dimensions, ease of surface functionalization, and, high stability have also imparted beneficial features to the cancer therapeutic regimens (Sun et al., 2014). Various works have also reported conferring “stealth” properties to nanomaterials by attachment of surface molecules such as polyethylene glycol (PEG) (Suk et al., 2016) and CD47 molecules (Gheibi Hayat et al., 2019) to prolong their systemic circulation time by delaying in vivo clearance (Fam et al., 2020). A recent study on the fabrication of nano-artificial antigen-presenting cells (aAPCs) decorated with both PEG and CD47 moieties have shown evasion of nanoparticles to phagocytic clearance along with their ability to induce the generation of antigen-specific T cells to suppress tumour development (Song et al., 2019). Another promising feature of NMs towards drug delivery applications is the ability to easily functionalize their surfaces with various ligands to improve cellular uptake and achieve a highly specific targeted drug delivery (Cisterna et al., 2016; Sykes et al., 2016).
Due to the enhanced surface area to volume ratio, the porous and hollow nanomaterials display excellent drug loading efficiencies (Yang et al., 2019). Such NMs are capable of efficiently encapsulating the hydrophobic (water-insoluble) cancer drugs as cargo within their framework, thereby improving the stability of drug molecules, protecting them from degradation, improving their bioavailability, and delivering them to the target site in a controlled manner (Kashi et al., 2012). The delivery of therapeutic agents (TAs) conjugated within nanomaterials also increases their circulation time, allows cellular- and/or tissue-specific targeting, and a stimuli-responsive controlled release of TAs at the target site. These factors drastically reduce the adverse effects of the TAs on the nearby cells and tissues (Iglesias et al., 2018). For instance, in the past decade, mesoporous silica nanoparticles (MSNs) have been exploited as stimuli-responsive nano-carriers for targeted delivery of a wide range of small molecules for diagnostic as well as therapeutic approaches. Major advantages of using MSN-type nano-systems for delivery of cytotoxic cancer drugs are improved drug efficacy, stimuli-responsive controlled release at the target site, and reduced toxic effects to nearby healthy tissues (Alyassin et al., 2020; Aquib et al., 2019; Li, T. et al., 2019; Nguyen et al., 2019). Another area of recent breakthroughs in cancer therapeutics has been in the field of NMs [such as gold nanostructures (Cheng et al., 2014; Lee et al., 2017), carbon dots (Li et al., 2016; Liyanage et al., 2020), carbon nanotubes (You et al., 2019), liposomes (Vieira and Gamarra, 2016; Jena et al., 2020), etc.] that possess the ability to cross the blood-brain barrier (BBB). This unique property of such NMs creates an opportunity to deliver therapeutic and imaging agents across the BBB and has stimulated widespread research in exploiting their application in brain-cancer treatment and diagnostics (Tang et al., 2019). Recently, an in vivo study conducted in mice has reported the first successful application of a polymeric nano-immuno-conjugate to deliver immune-therapeutics across BBB and stimulation of local antitumour immune response for the treatment of glioma tumours (Galstyan et al., 2019).
However, the adverse effects associated with the delivery of cytotoxic agents along with the increased chances of drug resistance largely overshadow the therapeutic effects of such an approach (Weber et al., 2015; Babiker et al., 2018; Makovec, 2019). This has led to the emergence of a much efficient treatment regimen for the eradication of tumours involving modifying the body’s own immune responses towards the tumour cells (Irvine et al., 2013). As described in the previous section, such immunomodulatory therapies are aimed at converting immunosuppressive TME into an immunostimulatory one, facilitating the elimination of cancer cells. This also leads to the generation of memory T-cells against cancer antigens thereby discouraging incidences of metastasis and future relapses (Curti et al., 2013; Locy et al., 2018). Consequently, recent works in the area of cancer therapeutics have been majorly focused on utilizing various biomolecules and nanomaterials to increase the specificity and efficacy of direct and/or indirect immunomodulation (Chidambaram et al., 2011). NMs have the potential to be utilized as a delivery system that allows in vivo imaging, sustained cargo release, enhanced circulation time, targeted delivery, and stimuli-responsive release of cargo molecules and multiple agents (Li, L. et al., 2018).
Interaction of NMs With the Immune System
The in-depth knowledge about the components of the immune system and their role in regulating numerous diseases when combined with the recent technological advancement in the field of fabrication of safe and biocompatible NMs have revolutionized the biomedical applications of nanomaterials. Nanomaterial-based therapies have been applied for the treatment of autoimmune diseases (Gao et al., 2017; Kwiatkowski et al., 2020), neurodegenerative diseases (Fornaguera et al., 2015; Turjeman et al., 2015), inflammatory diseases (Li, C. W. et al., 2020; Tran et al., 2015), and cancer (Xin et al., 2017; Samanta et al., 2019). When introduced in vivo, NMs act as foreign entities and consequently lead to various immune responses. Nearly all the NMs exert immunomodulatory effects in vivo which can be either immunostimulatory or immunosuppressive in nature. The unique ability of NMs to interact with components at a nanoscale and bring about change can lead to both therapeutic as well as deleterious events. As a result, in most of the biomedical applications, the modulatory effects of NMs are undesirable as they often lead to adverse effects. For instance, several recent in vitro and in vivo studies have demonstrated the severe immunotoxicity associated with the use of single-walled carbon nanotubes (SWCNTs) for biomedical applications (Sayes et al., 2006; Tian et al., 2006; Wang et al., 2011). The surface chemistry, concentration, density of functionalization, and surface area of SWCNTs critically influence their interaction with macrophages and the immune organs such as the spleen (Sayes et al., 2006; Zhang et al., 2011; Lee et al., 2015).
However, at times, the ability to modulate immune responses by NMs have shown compelling results and serves as a tool for immunomodulatory therapies. Therefore, NMs have found a wide-scale application as a tool to mediated immunomodulation either directly by interacting with the components of the immune system or indirectly by acting as a carrier for the delivery of immunomodulatory agents. Recent studies based on NM-mediated direct immunomodulation and NMs as a delivery system for IM agents are listed in Supplementary Tables S1 and S2 (supplied as Supplementary Material), respectively.
NM Mediated Direct and Indirect Immunomodulation
A variety of immunomodulatory NMs with a unique plethora of physical and chemical attributes have been fabricated for stimulating the immune system to combat cancer. For example, the distinctive ability of the platelets to target cancer cells and their inherent capacity to load drug molecules have been utilized to develop targeted drug delivery nanosystems for anti-cancer therapies. Liposome-based delivery systems are a popular platform for targeted delivery and sustained release of anti-cancer agents (Mukherjee et al., 2019). Various liposomal formulations have been developed for the delivery of immunomodulatory agents (IMAs) that allow modulation of the TME to establish an overall antitumor immune response again tumour antigens (Gilabert-Oriol et al., 2018). Recent studies have also highlighted the inherent tendency of liposomes to interact extensively with the immune system leading to several immunomodulatory effects on the growth of the tumour (La-Beck et al., 2019). Unique core-shell structure allows simultaneous co-delivery of multiple cargo molecules, in vivo protection against degradation and the facile surface chemistry can be applied to decorate the NM surface with active targeting moieties, thus making liposomes an excellent candidate for cancer therapeutic applications.
Additionally, several bio-inspired NMs have also gained popularity due to their enhanced biocompatibility and cyto-friendly nature, eco-friendly mode of synthesis, superior bioactivity when compared to the chemically synthesized NMs (Mukherjee and Patra, 2017). Biosynthesized metallic nanoparticles, especially gold (AuNPs) and silver (AgNPs) have been widely studied for achieving targeted cancer therapy and diagnosis (Balakrishnan et al., 2017; Mukherjee et al., 2015; Mukherjee et al., 2016). For example, a study based on biosynthesized colloidal AgNps prepared from leaf extract showed enhanced antibacterial and anti-cancer activity than the chemically synthesized NPs. The multifunctional AgNPs were biocompatible and exhibited bright red fluorescence upon intracellular localization emphasizing their potential to be utilized for biomedical diagnostic tools (Mukherjee et al., 2014). Thus, immunomodulatory NMs possess unique and tunable properties that hold great potential to be utilized as cancer therapeutic agents.
Clinical Studies and Perspectives
Regardless of the advantages of NMs, very few NM-based immunomodulatory cancer therapies have been actively translated to clinical application majorly due to the limited knowledge about the behavior of NMs when introduced in vivo and their long-term toxicity (Sun et al., 2019). The unique properties of NMs, while being attractive for various biomedical applications, at times also raise quite a few biosafety concerns. For example, due to the surface properties such as hydrophobicity and surface charge, NMs when introduced in vivo tend to agglomerate into larger particles that might lead to accumulation and toxicity (Pattan and Kaul, 2014). Moreover, as a result of their small size, NMs have the ability to penetrate cells and organelles. Several studies have also shown an increase in intracellular oxidative stress and damage when cells are exposed to NMs such as carbon nanotubes (Francis and Devasena, 2018), gold nanoparticles (Chen et al., 2009), copper nanoparticles (Meng et al., 2007), titanium NMs (Demir, 2020), etc. These events often lead to adverse effects such as DNA aberration, genetic instability and inflammation depending upon the cell type, nature of NM and material concentration (Demir, 2021).
Despite the limitations, some of the NM-based immunomodulatory therapies that are undergoing clinical trials hold immense potential towards a safe and successful clinical application in cancer therapeutics. For example, a recent human trial consisting of six patients suffering from glioblastoma (GBM) involved the use of superparamagnetic iron oxide nanoparticles (SPIONs) via the “NanoPaste” technique. The resection cavities were coated with SPIONs after which the patients were subjected to six sessions of hyperthermia using alternating magnetic field lasting for about 1 h each. No major side-effects were observed during the ongoing treatment. After 2–3 months of treatment, the imaging analysis revealed the formation of NP deposits that displayed signs of inflammation and edema. Further analysis indicated that the treatment was effective in eliciting an antitumor response by tumor necrosis, infiltration of macrophages following uptake of NPs, increasing CD3+ T-cells, higher secretion of IFN-γ, upregulated levels of Caspase-3, heat-shock protein 70, HLA-DR, and PD-L1 within the tumor microenvironment (TME). Out of the six patients, two showed prolonged therapeutic responses (>23 months) without further therapies; however, four had to undergo additional surgical procedures for removal of accumulated SPIONs (Grauer et al., 2019).
Gadolinium-chelated polysiloxane based nanoparticles (AGuIX) have shown promising results as a radiosensitizer both in vitro (Mowat et al., 2011) and in vivo (Sancey et al., 2015; Kotb et al., 2016). It has demonstrated great potential as a theranostic agent for enhancing MRI contrast and as a cytotoxic agent when conjugated with radiotherapy (Sancey et al., 2014). These findings have motivated several clinical studies for the safe application of these NPs in human cancer patients (Lux et al., 2019). An ongoing NANO-RAD trial (NCT02820454) utilizing gadolinium-chelated polysiloxane based nanoparticles (AGuIX) as a theranostic as well as a radiosensitizer agent has recently completed the recruitment process. This phase I study will consist of about 15 participants and is aimed at investigating the biosafety, dose tolerance, and toxicology of the AGuIX NPs when applied in combination with standard whole-brain radiotherapy (WBRT). The administration of Nps will be done intravenously (IV) followed by a magnetic resonance imaging (MRI) scan to analyze the distribution of NPs. The patients will be subjected to WBRT starting 4 h after IV administration, 5 days a week till 2 weeks of treatment. The pharmacological studies of AGuIX Nps will be performed at regular intervals by drawing blood from the patients. Phase II of this trial (NANORAD2; NCT03818386) is also currently under the recruitment stage. Another Phase II clinical trial to evaluate the clinical impact of AGuIX in combination with fractionated stereotactic radiotherapy (NANOSTEREO; NCT04094077) is currently recruiting. Additionally, another Phase I clinical trial (NANOCOL; NCT03308604) for patients with locally advanced cervical cancer is currently in the recruitment phase. The trial is based on evaluating the biosafety and dosage tolerance of AGuIX NPs in conjugation with radiation and cisplatin.
Another interesting research study (WDVAX; NCT01753089) which is based on the formulation of a personalized melanoma vaccine is currently under Phase I of clinical trials. The vaccine will contain tumor lysate obtained from the patient’s own melanoma tumor cells combined with other immunostimulatory agents such as CpG and granulocyte-macrophage colony-stimulating factor (GM-CSF). These components will be combined together in a polymeric biomaterial scaffold consisting of PLGA. The trial consists of 23 participants with metastatic melanoma and is aimed at establishing the biosafety and efficacy of the scaffold-based vaccine in modulating the patient’s own immune system to build effective antitumor responses against the melanoma tumor cells (Dolgin, 2013; Singh and Peppas, 2014).
Since, proteins and the immune cells are the first to encounter NMs upon administration into an in vivo biological system, therefore, prior to any clinical application, it is essential to critically assess the nature of interactions of NMs with the cells and the proteins of the living system so as to ensure that the benefits outweigh the limitations of any NM-based clinical therapy. However, majority of the present clinical trials tend to emphasize only on the therapeutic aspect of the NM-based treatments while leaving out the biosafety concerns involved. Also, there is a dire need to develop a unified and standardized procedure for determining the biosafety of NMs during research and the clinical studies in order to minimize adverse effects during human trials and ensuring that more and more NM-based therapies successfully attain clinical translation.
Conclusion
Immunomodulation based cancer therapies rely on altering the body’s own defenses to fight back and eradicate its enemy, cancer. The targeted therapeutic effect in minimal concentrations while causing minimum/no side-effects is the major reason for the success of this approach. However, due to the heterogeneous cellular composition of tumors, individual cancer therapies, especially the ones based on conventional approaches like surgery, radiation, and chemotherapy have shown limited success. When used in isolation, these treatments do not provide a curative measure for cancer. Therefore, to enhance the efficacy of the treatment and achieve better survivability, it is necessary to explore combinatorial cancer therapies. The unintended and unavoidable interactions of nanomaterials with the biological systems including the immune system is a major hurdle for tapping the optimum therapeutic effect. Due to this, only a sparse number of immunotherapies have attained successful clinical application. Thus, the future advancements in applying immunomodulatory nanomaterials for cancer therapeutics should be majorly focused on taking into consideration the biosafety aspects of the use of nanomaterial. Additionally, favorable aspect for the future cancer therapies will be based on delivery of more than one active agent so as to accommodate simultaneous diagnosis, targeting, treatment and monitoring. Such immunomodulatory-based cancer therapeutics will exhibit many promising results and improve survivability when used in conjugation with other techniques to create an effective anti-cancer regimen. Another aspect for potential research in this field can be towards achieving precisely targeted delivery and stimuli-responsive release of immunomodulatory therapeutic agents.
Author Contributions
AJ wrote the paper. AA did the study design, critically reviewed and proofread the manuscript. SS participated in brainstorming session and proofread the manuscript. All authors contributed to the article and approved the submitted version.
Conflict of Interest
The authors declare that the research was conducted in the absence of any commercial or financial relationships that could be construed as a potential conflict of interest.
Supplementary Material
The Supplementary Material for this article can be found online at: https://www.frontiersin.org/articles/10.3389/fchem.2021.629635/full#supplementary-material.
Glossary
Nanomaterials (NMs) NMs are a class of materials that possess at least one dimension within the range of 1–100 nm
Immunity The inherent ability of an organism to avert pathogens and diseases by recognition and subsequent neutralization or elimination of foreign entities by the organism’s immune system
Immunomodulation The process of altering the host’s immune system and its responses
Immuno-stimulation The process of enhancing the ability of the body to evoke a strong and robust immune response against an antigen
Immuno-suppression The diminished ability of the body to induce an immune response due to a lack of immune cells such as T and/or B lymphocyte
References
Abbott, M., and Ustoyev, Y. (2019). Cancer and the immune system: the history and background of immunotherapy. Semin. Oncol. Nurs. 10, 150923. doi:10.1016/j.soncn.2019.08.002
Al-Tameemi, M., Chaplain, M., and d'Onofrio, A. (2012). Evasion of tumours from the control of the immune system: consequences of brief encounters. Biol. Direct 7, 31. doi:10.1186/1745-6150-7-31
Alsaleh, N. B., and Brown, J. M. (2018). Immune responses to engineered nanomaterials: current understanding and challenges. Curr. Opin. Toxicol. 10, 8–14. doi:10.1016/j.cotox.2017.11.011
Alyassin, Y., Sayed, E. G., Mehta, P., Ruparelia, K., Arshad, M. S., Rasekh, M., et al. (2020). Application of mesoporous silica nanoparticles as drug delivery carriers for chemotherapeutic agents. Drug Discov. Today 25, 1513–1520. doi:10.1016/j.drudis.2020.06.006
Aquib, M., Farooq, M. A., Banerjee, P., Akhtar, F., Filli, M. S., Boakye-Yiadom, K. O., et al. (2019). Targeted and stimuli-responsive mesoporous silica nanoparticles for drug delivery and theranostic use. J. Biomed. Mater. Res. 107 (107), 2643–2666. doi:10.1002/jbm.a.36770
Asadi, N., Davaran, S., Panahi, Y., Hasanzadeh, A., Malakootikhah, J., Fallah Moafi, H., et al. (2017). Application of nanostructured drug delivery systems in immunotherapy of cancer: a review. Artif. Cells Nanomed. Biotechnol. 45, 18–23. doi:10.1080/21691401.2016.1178136
Babiker, H. M., McBride, A., Newton, M., Boehmer, L. M., Drucker, A. G., Gowan, M., et al. (2018). Cardiotoxic effects of chemotherapy: a review of both cytotoxic and molecular targeted oncology therapies and their effect on the cardiovascular system. Crit. Rev. Oncol. Hematol. 126, 186–200. doi:10.1016/j.critrevonc.2018.03.014
Baetke, S. C., Lammers, T., and Kiessling, F. (2015). Applications of nanoparticles for diagnosis and therapy of cancer. Br. J. Radiol. 88, 20150207. doi:10.1259/bjr.20150207
Balakrishnan, S., Mukherjee, S., Das, S., Bhat, F. A., Raja Singh, P., Patra, C. R., et al. (2017). Gold nanoparticles-conjugated quercetin induces apoptosis via inhibition of EGFR/PI3K/Akt-mediated pathway in breast cancer cell lines (MCF-7 and MDA-MB-231). Cell Biochem. Funct. 35, 217–231. doi:10.1002/cbf.3266
Ballas, Z. K. (2018). The 2018 Nobel Prize in Physiology or Medicine: an exemplar of bench to bedside in immunology. J. Allergy Clin. Immunol. 12 (142), 1752–1753. doi:10.1016/j.jaci.2018.10.021
Bartneck, M. (2017). Immunomodulatory nanomedicine. Macromol. Biosci. 17, 17. doi:10.1002/mabi.201700021
Belardelli, F., and Ferrantini, M. (2002). Cytokines as a link between innate and adaptive antitumor immunity. Trends Immunol. 23, 201–208. doi:10.1016/s1471-4906(02)02195-6
Bleeker, E. A., de Jong, W. H., Geertsma, R. E., Groenewold, M., Heugens, E. H., Koers-Jacquemijns, M., et al. (2013). Considerations on the EU definition of a nanomaterial: science to support policy making. Regul. Toxicol. Pharmacol. 65, 119–125. doi:10.1016/j.yrtph.2012.11.007
Bonaventura, P., Shekarian, T., Alcazer, V., Valladeau-Guilemond, J., Valsesia-Wittmann, S., Amigorena, S., et al. (2019). Cold tumors: a therapeutic challenge for immunotherapy. Front. Immunol. 10, 168. doi:10.3389/fimmu.2019.00168
Bonilla, F. A., and Oettgen, H. C. (2010). Adaptive immunity. J. Allergy Clin. Immunol. 125, S33–S40. doi:10.1016/j.jaci.2009.09.017
Braff, M. H., and Gallo, R. L. (2006). Antimicrobial peptides: an essential component of the skin defensive barrier. Curr. Top. Microbiol. Immunol. 306, 91–110. doi:10.1007/3-540-29916-5_4
Broide, D. H. (2009). Immunomodulation of allergic disease. Annu. Rev. Med. 60, 279–291. doi:10.1146/annurev.med.60.041807.123524
Buabeid, M. A., Arafa, E. A., and Murtaza, G. (2020). Emerging prospects for nanoparticle-enabled cancer immunotherapy. J. Immunol. Res. 2020, 9624532. doi:10.1155/2020/9624532
Calì, B., Molon, B., and Viola, A. (2017). Tuning cancer fate: the unremitting role of host immunity. Open Biol. 7, 7. doi:10.1098/rsob.170006
Carballido, J. M., Regairaz, C., Rauld, C., Raad, L., Picard, D., and Kammüller, M. (2020). The emerging jamboree of transformative therapies for autoimmune diseases. Front. Immunol. 11, 472. doi:10.3389/fimmu.2020.00472
Chan, C. W., Crafton, E., Fan, H. N., Flook, J., Yoshimura, K., Skarica, M., et al. (2006). Interferon-producing killer dendritic cells provide a link between innate and adaptive immunity. Nat. Med. 12, 207–213. doi:10.1038/nm1352
Chang, R., Wang, P. Y., and Tseng, C. L. (2018). New combination/application of polymer-based nanoparticles for biomedical engineering. Adv. Exp. Med. Biol. 1078, 271–290. doi:10.1007/978-981-13-0950-2_14
Chen, D. S., and Mellman, I. (2017). Elements of cancer immunity and the cancer-immune set point. Nature. 541, 321–330. doi:10.1038/nature21349
Chen, D. S., and Mellman, I. (2013). Oncology meets immunology: the cancer-immunity cycle. Immunity. 39, 1–10. doi:10.1016/j.immuni.2013.07.012
Chen, H., Zhang, W., Zhu, G., Xie, J., and Chen, X. (2017). Rethinking cancer nanotheranostics. Nat Rev Mater. 2, 24. doi:10.1038/natrevmats.2017.24
Chen, Y. S., Hung, Y. C., Liau, I., and Huang, G. S. (2009). Assessment of the in vivo toxicity of gold nanoparticles. Nanoscale Res. Lett. 4, 858–864. doi:10.1007/s11671-009-9334-6
Cheng, Y., Dai, Q., Morshed, R. A., Fan, X., Wegscheid, M. L., Wainwright, D. A., et al. (2014). Blood-brain barrier permeable gold nanoparticles: an efficient delivery platform for enhanced malignant glioma therapy and imaging. Small. 10, 5137–5150. doi:10.1002/smll.201400654
Chidambaram, M., Manavalan, R., and Kathiresan, K. (2011). Nanotherapeutics to overcome conventional cancer chemotherapy limitations. J. Pharm. Pharm. Sci. 14, 67–77. doi:10.18433/j30c7d
Chircop, M., and Speidel, D. (2014). Cellular stress responses in cancer and cancer therapy. Front. Oncol. 4, 304. doi:10.3389/fonc.2014.00304
Cisterna, B. A., Kamaly, N., Choi, W. I., Tavakkoli, A., Farokhzad, O. C., and Vilos, C. (2016). Targeted nanoparticles for colorectal cancer. Nanomedicine. 11, 2443–2456. doi:10.2217/nnm-2016-0194
Coates, M., Blanchard, S., and MacLeod, A. S. (2018). Innate antimicrobial immunity in the skin: a protective barrier against bacteria, viruses, and fungi. PLoS Pathog. 14 (14), e1007353. doi:10.1371/journal.ppat.1007353
Coley, W. B. (1991). The treatment of malignant tumors by repeated inoculations of erysipelas. With a report of ten original cases. Clin. Orthop. Relat. Res. 1893, 3–11.
Comparetti, E. J., Pedrosa, V. A., and Kaneno, R. (2018). Carbon nanotube as a tool for fighting cancer. Bioconjug. Chem. 3, 709–718. doi:10.1021/acs.bioconjchem.7b00563
Cooper, E. L. (2016). Commentary: blurring borders: innate immunity with adaptive features. Front. Microbiol. 7, 358. doi:10.3389/fmicb.2016.00358
Cosentino, D., and Piro, F. (2018). Hyaluronic acid for treatment of the radiation therapy side effects: a systematic review. Eur. Rev. Med. Pharmacol. Sci. 11 (22), 7562–7572. doi:10.26355/eurrev_201811_16298
Curiel, T. J. (2007). Tregs and rethinking cancer immunotherapy. J. Clin. Invest. 117, 1167–1174. doi:10.1172/JCI31202
Curti, B. D., Kovacsovics-Bankowski, M., Morris, N., Walker, E., Chisholm, L., Floyd, K., et al. (2013). OX40 is a potent immune-stimulating target in late-stage cancer patients. Cancer Res. 73, 7189–7198. doi:10.1158/0008-5472.CAN-12-4174
D'Errico, G., Machado, H. L., and Sainz, B. (2017). A current perspective on cancer immune therapy: step-by-step approach to constructing the magic bullet. Clin. Transl. Med. 6, 3. doi:10.1186/s40169-016-0130-5
Dai, Z., Leung, H. M., and Lo, P. K. (2017). Stimuli-responsive self-assembled DNA nanomaterials for biomedical applications. Small. 13, 13. doi:10.1002/smll.201602881
de Visser, K. E., Eichten, A., and Coussens, L. M. (2006). Paradoxical roles of the immune system during cancer development. Nat. Rev. Cancer. 6, 24–37. doi:10.1038/nrc1782
Decker, W. K., and Safdar, A. (2009). Bioimmunoadjuvants for the treatment of neoplastic and infectious disease: Coley's legacy revisited. Cytokine Growth Factor Rev. 20, 271–281. doi:10.1016/j.cytogfr.2009.07.004
Demir, E. (2021). A review on nanotoxicity and nanogenotoxicity of different shapes of nanomaterials. J. Appl. Toxicol. 41, 118–147. doi:10.1002/jat.4061
Demir, E. (2020). An in vivo study of nanorod, nanosphere, and nanowire forms of titanium dioxide using Drosophila melanogaster: toxicity, cellular uptake, oxidative stress, and DNA damage. J. Toxicol. Environ. Health 83, 456–469. doi:10.1080/15287394.2020.1777236
Desai, N., Trieu, V., Yao, Z., Louie, L., Ci, S., Yang, A., et al. (2006). Increased antitumor activity, intratumor paclitaxel concentrations, and endothelial cell transport of cremophor-free, albumin-bound paclitaxel, ABI-007, compared with cremophor-based paclitaxel. Clin. Cancer Res. 12, 1317–1324. doi:10.1158/1078-0432.CCR-05-1634
Dhivya, S., Ajita, J., and Selvamurugan, N. (2015). Metallic nanomaterials for bone tissue engineering. J. Biomed. Nanotechnol. 11, 1675–1700. doi:10.1166/jbn.2015.2115
Dhodapkar, K. M. (2019). Autoimmune complications of cancer immunotherapy. Curr. Opin. Immunol. 12 (61), 54–59. doi:10.1016/j.coi.2019.08.004
Dickneite, G., Kaspereit, F., and Sedlacek, H. H. (1984). Stimulation of cell-mediated immunity by bestatin correlates with reduction of bacterial persistence in experimental chronic Salmonella typhimurium infection. Infect. Immun. 44, 168–174. doi:10.1128/IAI.44.1.168-174.1984
Diou, O., Tsapis, N., and Fattal, E. (2012). Targeted nanotheranostics for personalized cancer therapy. Expert Opin. Drug Deliv. 9, 1475–1487. doi:10.1517/17425247.2012.736486
Dittmer, U., and Olbrich, A. R. (2003). Treatment of infectious diseases with immunostimulatory oligodeoxynucleotides containing CpG motifs. Curr. Opin. Microbiol. 6, 472–477. doi:10.1016/j.mib.2003.09.007
Draghi, A., Chamberlain, C. A., Furness, A., and Donia, M. (2019). Acquired resistance to cancer immunotherapy. Semin. Immunopathol. 41, 31–40. doi:10.1007/s00281-018-0692-y
Drews, J. (1980). A role for immune stimulation in the treatment of microbial infections?. Infection 8, 2–4. doi:10.1007/BF01677390
Duan, Q., Zhang, H., Zheng, J., and Zhang, L. (2020). Turning cold into hot: firing up the tumor microenvironment. Trends Cancer 7 (6), 605–618. doi:10.1016/j.trecan.2020.02.022
Dunn, G. P., Bruce, A. T., Ikeda, H., Old, L. J., and Schreiber, R. D. (2002). Cancer immunoediting: from immunosurveillance to tumor escape. Nat. Immunol. 3, 991–998. doi:10.1038/ni1102-991
Dunn, G. P., Koebel, C. M., and Schreiber, R. D. (2006). Interferons, immunity and cancer immunoediting. Nat. Rev. Immunol. 6, 836–848. doi:10.1038/nri1961
Dunn, G. P., Old, L. J., and Schreiber, R. D. (2004). The three Es of cancer immunoediting. Annu. Rev. Immunol. 22, 329–360. doi:10.1146/annurev.immunol.22.012703.104803
Elzey, B. D., Sprague, D. L., and Ratliff, T. L. (2005). The emerging role of platelets in adaptive immunity. Cell. Immunol. 238, 1–9. doi:10.1016/j.cellimm.2005.12.005
Elzey, B. D., Tian, J., Jensen, R. J., Swanson, A. K., Lees, J. R., Lentz, S. R., et al. (2003). Platelet-mediated modulation of adaptive immunity. A communication link between innate and adaptive immune compartments. Immunity 19, 9–19. doi:10.1016/s1074-7613(03)00177-8
Ephrem, A., Misra, N., Hassan, G., Dasgupta, S., Delignat, S., Duong Van Huyen, J. P., et al. (2005). Immunomodulation of autoimmune and inflammatory diseases with intravenous immunoglobulin. Clin. Exp. Med. 5, 135–140. doi:10.1007/s10238-005-0079-y
Fam, S. Y., Chee, C. F., Yong, C. Y., Ho, K. L., Mariatulqabtiah, A. R., and Tan, W. S. (2020). Stealth coating of nanoparticles in drug-delivery systems. Nanomaterials 10, 787. doi:10.3390/nano10040787
Fehniger, T. A., Cooper, M. A., Nuovo, G. J., Cella, M., Facchetti, F., Colonna, M., et al. (2003). CD56bright natural killer cells are present in human lymph nodes and are activated by T cell-derived IL-2: a potential new link between adaptive and innate immunity. Blood 101, 3052–3057. doi:10.1182/blood-2002-09-2876
Feng, X., Xu, W., Li, Z., Song, W., Ding, J., and Chen, X. (2019). Immunomodulatory nanosystems. Adv. Sci. 6, 1900101. doi:10.1002/advs.201900101
Ferrari, S., Rovati, B., Porta, C., Alessandrino, P. E., Bertolini, A., Collovà, E., et al. (2003). Lack of dendritic cell mobilization into the peripheral blood of cancer patients following standard- or high-dose chemotherapy plus granulocyte-colony stimulating factor. Cancer Immunol. Immunother. 52, 359–366. doi:10.1007/s00262-002-0365-4
Finlay, B. B., and McFadden, G. (2006). Anti-immunology: evasion of the host immune system by bacterial and viral pathogens. Cell 124, 767–782. doi:10.1016/j.cell.2006.01.034
Fornaguera, C., Feiner-Gracia, N., Calderó, G., García-Celma, M. J., and Solans, C. (2015). Galantamine-loaded PLGA nanoparticles, from nano-emulsion templating, as novel advanced drug delivery systems to treat neurodegenerative diseases. Nanoscale 7, 12076–12084. doi:10.1039/c5nr03474d
Francis, A. P., and Devasena, T. (2018). Toxicity of carbon nanotubes: a review. Toxicol. Ind. Health 34, 200–210. doi:10.1177/0748233717747472
Fukuda, A., Tahara, K., Hane, Y., Matsui, T., Sasaoka, S., Hatahira, H., et al. (2017). Comparison of the adverse event profiles of conventional and liposomal formulations of doxorubicin using the FDA adverse event reporting system. PLoS One 12, e0185654. doi:10.1371/journal.pone.0185654
Galstyan, A., Markman, J. L., Shatalova, E. S., Chiechi, A., Korman, A. J., Patil, R., et al. (2019). Blood-brain barrier permeable nano immunoconjugates induce local immune responses for glioma therapy. Nat. Commun. 08, 3850.
Gao, W., Xiong, Y., Li, Q., and Yang, H. (2017). Inhibition of toll-like receptor signaling as a promising therapy for inflammatory diseases: a journey from molecular to nano therapeutics. Front. Physiol. 8, 508. doi:10.3389/fphys.2017.00508
García-Martínez, E., Smith, M., Buqué, A., Aranda, F., de la Peña, F. A., Ivars, A., et al. (2018). Trial Watch: Immunostimulation with recombinant cytokines for cancer therapy. Oncoimmunology 7, e1433982.
Gheibi Hayat, S. M., Bianconi, V., Pirro, M., and Sahebkar, A. (2019). Stealth functionalization of biomaterials and nanoparticles by CD47 mimicry. Int. J. Pharm. 569, 118628. doi:10.1016/j.ijpharm.2019.118628
Gilabert-Oriol, R., Ryan, G. M., Leung, A. W. Y., Firmino, N. S., Bennewith, K. L., and Bally, M. B. (2018). Liposomal formulations to modulate the tumour microenvironment and antitumour immune response. Int. J. Mol. Sci. 19 (10), 2922. doi:10.3390/ijms19102922
Grabowski, M. M., Sankey, E. W., Ryan, K. J., Chongsathidkiet, P., Lorrey, S. J., Wilkinson, D. S., et al. (2020). Immune suppression in gliomas. J. Neuro Oncol. 151 (1), 3–12. doi:10.1007/s11060-020-03483-y
Grauer, O., Jaber, M., Hess, K., Weckesser, M., Schwindt, W., Maring, S., et al. (2019). Combined intracavitary thermotherapy with iron oxide nanoparticles and radiotherapy as local treatment modality in recurrent glioblastoma patients. J. Neuro Oncol. 141, 83–94. doi:10.1007/s11060-018-03005-x
Gregersen, P. K., and Behrens, T. W. (2006). Genetics of autoimmune diseases--disorders of immune homeostasis. Nat. Rev. Genet. 7, 917–928. doi:10.1038/nrg1944
Hall, M. W., Joshi, I., Leal, L., and Ooi, E. E. (2020). Immune modulation in COVID-19: strategic considerations for personalized therapeutic intervention. Clin. Infect. Dis. ciaa904. doi:10.1093/cid/ciaa904
Hapuarachchige, S., and Artemov, D. (2020). Theranostic pretargeting drug delivery and imaging platforms in cancer precision medicine. Front. Oncol. 10, 1131. doi:10.3389/fonc.2020.01131
Hegde, P. S., and Chen, D. S. (2020). Top 10 challenges in cancer immunotherapy. Immunity 52, 17–35. doi:10.1016/j.immuni.2019.12.011
Houghton, A. N. (1994). Cancer antigens: immune recognition of self and altered self. J. Exp. Med. 180, 1–4. doi:10.1084/jem.180.1.1
Iglesias, N., Galbis, E., Díaz-Blanco, M. J., de-Paz, M. V., and Galbis, J. A. (2018). Loading studies of the anticancer drug camptothecin into dual stimuli-sensitive nanoparticles. Stability scrutiny. Int. J. Pharm. 550, 429–438. doi:10.1016/j.ijpharm.2018.08.026
Immunotherapies for Autoimmune Diseases (2019). Cancer immunotherapies repurposed for use in autoimmunity. Nat. Biomed. Eng. 3 (4), 247. doi:10.1038/s41551-019-0394-3
Ingraham, N. E., Lotfi-Emran, S., Thielen, B. K., Techar, K., Morris, R. S., Holtan, S. G., et al. (2020). Immunomodulation in COVID-19. Lancet Respir. Med. 6, 544–546. doi:10.1016/S2213-2600(20)30226-5
Irvine, D. J., Swartz, M. A., and Szeto, G. L. (2013). Engineering synthetic vaccines using cues from natural immunity. Nat. Mater. 12, 978–990. doi:10.1038/nmat3775
Iwasaki, A., and Medzhitov, R. (2015). Control of adaptive immunity by the innate immune system. Nat. Immunol. 16, 343–353. doi:10.1038/ni.3123
Jena, L., McErlean, E., and McCarthy, H. (2020). Delivery across the blood-brain barrier: nanomedicine for glioblastoma multiforme. Drug Deliv. Transl. Res. 10, 304–318. doi:10.1007/s13346-019-00679-2
Jiang, B. P., Zhou, B., Lin, Z., Liang, H., and Shen, X. C. (2019). Recent advances in carbon nanomaterials for cancer phototherapy. Chemistry 25, 3993–4004. doi:10.1002/chem.201804383
Jiang, Q., Luo, Z., Men, Y., Yang, P., Peng, H., Guo, R., et al. (2017). Red blood cell membrane-camouflaged melanin nanoparticles for enhanced photothermal therapy. Biomaterials 143, 29–45. doi:10.1016/j.biomaterials.2017.07.027
Jo, S. D., Ku, S. H., Won, Y. Y., Kim, S. H., and Kwon, I. C. (2016). Targeted nanotheranostics for future personalized medicine: recent progress in cancer therapy. Theranostics 6, 1362–1377. doi:10.7150/thno.15335
Kashi, T. S., Eskandarion, S., Esfandyari-Manesh, M., Marashi, S. M., Samadi, N., Fatemi, S. M., et al. (2012). Improved drug loading and antibacterial activity of minocycline-loaded PLGA nanoparticles prepared by solid/oil/water ion pairing method. Int. J. Nanomed. 7, 221–234. doi:10.2147/IJN.S27709
Kazemi, T., Younesi, V., Jadidi-Niaragh, F., and Yousefi, M. (2016). Immunotherapeutic approaches for cancer therapy: an updated review. Artif Cells Nanomed. Biotechnol. 44, 769–779. doi:10.3109/21691401.2015.1019669
Kelly, J., and Leonard, W. J. (2003). Immune deficiencies due to defects in cytokine signaling. Curr. Allergy Asthma Rep. 3, 396–401. doi:10.1007/s11882-003-0073-y
Kitamura, T., Qian, B. Z., and Pollard, J. W. (2015). Immune cell promotion of metastasis. Nat. Rev. Immunol. 15, 73–86. doi:10.1038/nri3789
Knochelmann, H. M., Dwyer, C. J., Bailey, S. R., Amaya, S. M., Elston, D. M., Mazza-McCrann, J. M., et al. (2018). When worlds collide: Th17 and Treg cells in cancer and autoimmunity. Cell. Mol. Immunol. 15, 458–469. doi:10.1038/s41423-018-0004-4
Kotb, S., Detappe, A., Lux, F., Appaix, F., Barbier, E. L., Tran, V. L., et al. (2016). Gadolinium-based nanoparticles and radiation therapy for multiple brain melanoma metastases: proof of concept before phase I trial. Theranostics 6, 418–427. doi:10.7150/thno.14018
Kroemer, G., and Zitvogel, L. (2018). Cancer immunotherapy in 2017: the breakthrough of the microbiota. Nat. Rev. Immunol. 18, 87–88. doi:10.1038/nri.2018.4
Kubackova, J., Zbytovska, J., and Holas, O. (2020). Nanomaterials for direct and indirect immunomodulation: a review of applications. Eur. J. Pharm. Sci. 142, 105139. doi:10.1016/j.ejps.2019.105139
Kwiatkowski, A. J., Stewart, J. M., Cho, J. J., Avram, D., and Keselowsky, B. G. (2020). Nano and microparticle emerging strategies for treatment of autoimmune diseases: multiple sclerosis and type 1 diabetes. Adv. Healthc. Mater. 6 (9), e2000164.
La-Beck, N. M., Liu, X., and Wood, L. M. (2019). Harnessing liposome interactions with the immune system for the next breakthrough in cancer drug delivery. Front. Pharmacol. 10, 220. doi:10.3389/fphar.2019.00220
Lakshmi Narendra, B., Eshvendar Reddy, K., Shantikumar, S., and Ramakrishna, S. (2013). Immune system: a double-edged sword in cancer. Inflamm. Res. 62, 823–834. doi:10.1007/s00011-013-0645-9
Lee, C., Hwang, H. S., Lee, S., Kim, B., Kim, J. O., Oh, K. T., et al. (2017). Rabies virus-inspired silica-coated gold nanorods as a photothermal therapeutic platform for treating brain tumors. Adv. Mater. Weinheim 29, 5563. doi:10.1002/adma.201605563
Lee, S., Khang, D., and Kim, S. H. (2015). High dispersity of carbon nanotubes diminishes immunotoxicity in spleen. Int. J. Nanomed. 10, 2697–2710. doi:10.2147/IJN.S80836
Lei, X., Lei, Y., Li, J. K., Du, W. X., Li, R. G., Yang, J., et al. (2020). Immune cells within the tumor microenvironment: biological functions and roles in cancer immunotherapy. Cancer Lett. 2, 126–133. doi:10.1016/j.canlet.2019.11.009
Li, B., Wang, F., Gui, L., He, Q., Yao, Y., and Chen, H. (2018). The potential of biomimetic nanoparticles for tumor-targeted drug delivery. Nanomedicine 13, 2099–2118. doi:10.2217/nnm-2018-0017
Li, C., and Han, X. (2020). Melanoma cancer immunotherapy using PD-L1 siRNA and imatinib promotes cancer-immunity cycle. Pharm. Res. 37, 109. doi:10.1007/s11095-020-02838-4
Li, C. W., Li, L. L., Chen, S., Zhang, J. X., and Lu, W. L. (2020). Antioxidant nanotherapies for the treatment of inflammatory diseases. Front. Bioeng. Biotechnol. 8, 200. doi:10.3389/fbioe.2020.00200
Li, J., Van Valkenburgh, J., Hong, X., Conti, P. S., Zhang, X., and Chen, K. (2019). Small molecules as theranostic agents in cancer immunology. Theranostics 9, 7849–7871. doi:10.7150/thno.37218
Li, L., and Neaves, W. B. (2006). Normal stem cells and cancer stem cells: the niche matters. Cancer Res. 66, 4553–4557. doi:10.1158/0008-5472.CAN-05-3986
Li, L., Wang, J., Kong, H., Zeng, Y., and Liu, G. (2018). Functional biomimetic nanoparticles for drug delivery and theranostic applications in cancer treatment. Sci. Technol. Adv. Mater. 19, 771–790. doi:10.1080/14686996.2018.1528850
Li, Q., Zhang, D., Zhang, J., Jiang, Y., Song, A., Li, Z., et al. (2019). A three-in-one immunotherapy nanoweapon via Cascade-amplifying cancer-immunity cycle against tumor metastasis, relapse, and postsurgical regrowth. Nano Lett. 19, 6647–6657. doi:10.1021/acs.nanolett.9b02923
Li, S., Amat, D., Peng, Z., Vanni, S., Raskin, S., De Angulo, G., et al. (2016). Transferrin conjugated nontoxic carbon dots for doxorubicin delivery to target pediatric brain tumor cells. Nanoscale 8, 16662–16669. doi:10.1039/c6nr05055g
Li, T., Qin, X., Li, Y., Shen, X., Li, S., Yang, H., et al. (2020). Cell membrane coated-biomimetic nanoplatforms toward cancer theranostics. Front. Bioeng. Biotechnol. 8, 371. doi:10.3389/fbioe.2020.00371
Li, T., Shi, S., Goel, S., Shen, X., Xie, X., Chen, Z., et al. (2019). Recent advancements in mesoporous silica nanoparticles towards therapeutic applications for cancer. Acta Biomater. 4, 1–13. doi:10.1016/j.actbio.2019.02.031
Liu, Y., and Cao, X. (2016). Immunosuppressive cells in tumor immune escape and metastasis. J Mol Med. 94, 509–522. doi:10.1007/s00109-015-1376-x
Liu, Y., Wang, X., Hussain, M., Lv, M., Dong, X., Wang, T., et al. (2018). Theranostics applications of nanoparticles in cancer immunotherapy. Med. Sci. 6. doi:10.3390/medsci6040100
Liu, Z., Jiang, W., Nam, J., Moon, J. J., and Kim, B. Y. S. (2018). Immunomodulating nanomedicine for cancer therapy. Nano Lett. 18 (18), 6655–6659. doi:10.1021/acs.nanolett.8b02340
Liyanage, P. Y., Zhou, Y., Al-Youbi, A. O., Bashammakh, A. S., El-Shahawi, M. S., Vanni, S., et al. (2020). Pediatric glioblastoma target-specific efficient delivery of gemcitabine across the blood-brain barrier via carbon nitride dots. Nanoscale. 12, 7927–7938. doi:10.1039/d0nr01647k
Locy, H., de Mey, S., de Mey, W., De Ridder, M., Thielemans, K., and Maenhout, S. K. (2018). Immunomodulation of the tumor microenvironment: turn foe into friend. Front. Immunol. 9, 2909. doi:10.3389/fimmu.2018.02909
Lorente, C., Arias, J. L., Cabeza, L., Ortiz, R., Prados, J. C., Melguizo, C., et al. (2018). Nano-engineering of biomedical prednisolone liposomes: evaluation of the cytotoxic effect on human colon carcinoma cell lines. J. Pharm. Pharmacol. 70, 488–497. doi:10.1111/jphp.12882
Lux, F., Tran, V. L., Thomas, E., Dufort, S., Rossetti, F., Martini, M., et al. (2019). AGuIX® from bench to bedside-Transfer of an ultrasmall theranostic gadolinium-based nanoparticle to clinical medicine. Br. J. Radiol. 92, 20180365. doi:10.1259/bjr.20180365
Makovec, T. (2019). Cisplatin and beyond: molecular mechanisms of action and drug resistance development in cancer chemotherapy. Radiol. Oncol. 03, 148–158.
Martinon, F., and Tschopp, J. (2004). Inflammatory caspases: linking an intracellular innate immune system to autoinflammatory diseases. Cell. 117, 561–574. doi:10.1016/j.cell.2004.05.004
Melero, I., Rouzaut, A., Motz, G. T., and Coukos, G. (2014). T-cell and NK-cell infiltration into solid tumors: a key limiting factor for efficacious cancer immunotherapy. Cancer Discov. 4, 522–526. doi:10.1158/2159-8290.CD-13-0985
Meng, H., Chen, Z., Xing, G., Yuan, H., Chen, C., Zhao, F., et al. (2007). Ultrahigh reactivity provokes nanotoxicity: explanation of oral toxicity of nano-copper particles. Toxicol. Lett. 175, 102–110. doi:10.1016/j.toxlet.2007.09.015
Met, Ö., Jensen, K. M., Chamberlain, C. A., Donia, M., and Svane, I. M. (2019). Principles of adoptive T cell therapy in cancer. Semin. Immunopathol. 41, 49–58. doi:10.1007/s00281-018-0703-z
Miliotou, A. N., and Papadopoulou, L. C. (2018). CAR T-cell therapy: a new era in cancer immunotherapy. Curr. Pharm. Biotechnol. 19, 5–18. doi:10.2174/1389201019666180418095526
Mittal, D., Gubin, M. M., Schreiber, R. D., and Smyth, M. J. (2014). New insights into cancer immunoediting and its three component phases--elimination, equilibrium and escape. Curr. Opin. Immunol. 27, 16–25. doi:10.1016/j.coi.2014.01.004
Mohme, M., Riethdorf, S., and Pantel, K. (2017). Circulating and disseminated tumour cells - mechanisms of immune surveillance and escape. Nat. Rev. Clin. Oncol. 14, 155–167. doi:10.1038/nrclinonc.2016.144
Moser, M., and Leo, O. (2010). Key concepts in immunology. Vaccine. 28 (Suppl. 3), C2–C13. doi:10.1016/j.vaccine.2010.07.022
Mowat, P., Mignot, A., Rima, W., Lux, F., Tillement, O., Roulin, C., et al. (2011). In vitro radiosensitizing effects of ultrasmall gadolinium based particles on tumour cells. J. Nanosci. Nanotechnol. 11, 7833–7839. doi:10.1166/jnn.2011.4725
Muenst, S., Läubli, H., Soysal, S. D., Zippelius, A., Tzankov, A., and Hoeller, S. (2016). The immune system and cancer evasion strategies: therapeutic concepts. J. Intern. Med. 279, 541–562. doi:10.1111/joim.12470
Mühlberger, M., Janko, C., Unterweger, H., Friedrich, R. P., Friedrich, B., Band, J., et al. (2019). Functionalization of T lymphocytes with citrate-coated superparamagnetic iron oxide nanoparticles for magnetically controlled immune therapy. Int. J. Nanomed. 14, 8421–8432. doi:10.2147/IJN.S218488
Mukherjee, A., Waters, A. K., Kalyan, P., Achrol, A. S., Kesari, S., and Yenugonda, V. M. (2019). Lipid-polymer hybrid nanoparticles as a next-generation drug delivery platform: state of the art, emerging technologies, and perspectives. Int. J. Nanomed. 14, 1937–1952. doi:10.2147/IJN.S198353
Mukherjee, S., Chowdhury, D., Kotcherlakota, R., Patra, S., Bhadra, M. P., Bojja, S., et al. (2014). Potential theranostics application of bio-synthesized silver nanoparticles (4-in-1 system). Theranostics 4, 316–335. doi:10.7150/thno.7819
Mukherjee, S., Dasari, M., Priyamvada, S., Kotcherlakota, R., Bollu, V. S., and Patra, C. R. (2015). A green chemistry approach for the synthesis of gold nanoconjugates that induce the inhibition of cancer cell proliferation through induction of oxidative stress and their in vivo toxicity study. J. Mater. Chem. B. 3, 3820–3830. doi:10.1039/c5tb00244c
Mukherjee, S., and Patra, C. R. (2017). Biologically synthesized metal nanoparticles: recent advancement and future perspectives in cancer theranostics. Future Sci. OA. 3, FSO203. doi:10.4155/fsoa-2017-0035
Mukherjee, S., Sau, S., Madhuri, D., Bollu, V. S., Madhusudana, K., Sreedhar, B., et al. (2016). Green synthesis and Characterization of monodispersed gold nanoparticles: toxicity study, delivery of doxorubicin and its bio-distribution in mouse model. J. Biomed. Nanotechnol. 12, 165–181. doi:10.1166/jbn.2016.2141
Musette, P., and Bouaziz, J. D. (2018). B cell modulation strategies in autoimmune diseases: new concepts. Front. Immunol. 9, 622. doi:10.3389/fimmu.2018.00622
Nguyen, T. L., Choi, Y., and Kim, J. (2019). Mesoporous silica as a versatile platform for cancer immunotherapy. Adv. Mater. 31, e1803953. doi:10.1002/adma.201803953
Nishiguchi, A., and Taguchi, T. (2020). Sustained-immunostimulatory nanocellulose scaffold to enhance vaccine efficacy. J. Biomed. Mater. Res. 108, 1159–1170. doi:10.1002/jbm.a.36890
Palucka, K., and Banchereau, J. (1999). Dendritic cells: a link between innate and adaptive immunity. J. Clin. Immunol. 19, 12–25.
Pancer, Z., and Cooper, M. D. (2006). The evolution of adaptive immunity. Annu. Rev. Immunol. 24, 497–518. doi:10.1146/annurev.immunol.24.021605.090542
Parkin, J., and Cohen, B. (2001). An overview of the immune system. Lancet 357, 1777–1789. doi:10.1016/S0140-6736(00)04904-7
Pasare, C., and Medzhitov, R. (2004). Toll-like receptors: linking innate and adaptive immunity. Microb. Infect. 6, 1382–1387. doi:10.1016/j.micinf.2004.08.018
Pattan, G., and Kaul, G. (2014). Health hazards associated with nanomaterials. Toxicol. Ind. Health 30, 499–519. doi:10.1177/0748233712459900
Pauken, K. E., Dougan, M., Rose, N. R., Lichtman, A. H., and Sharpe, A. H. (2019). Adverse events following cancer immunotherapy: obstacles and opportunities. Trends Immunol. 06, 511–523.
Pearce, A., Haas, M., Viney, R., Pearson, S. A., Haywood, P., Brown, C., et al. (2017). Incidence and severity of self-reported chemotherapy side effects in routine care: a prospective cohort study. PLoS One 12, e0184360. doi:10.1371/journal.pone.0184360
Peng, J., Hamanishi, J., Matsumura, N., Abiko, K., Murat, K., Baba, T., et al. (2015). Chemotherapy induces programmed cell death-ligand 1 overexpression via the nuclear factor-κb to Foster an immunosuppressive tumor microenvironment in ovarian cancer. Cancer Res. 75, 5034–5045. doi:10.1158/0008-5472.CAN-14-3098
Perez, C. R., and De Palma, M. (2019). Engineering dendritic cell vaccines to improve cancer immunotherapy. Nat. Commun. 11 (10), 5408.
Pusuluri, A., Wu, D., and Mitragotri, S. (2019). Immunological consequences of chemotherapy: single drugs, combination therapies and nanoparticle-based treatments. J. Control Release. 7, 130–154. doi:10.1016/j.jconrel.2019.04.020
Qin, W., Huang, G., Chen, Z., and Zhang, Y. (2017). Nanomaterials in targeting cancer stem cells for cancer therapy. Front. Pharmacol. 8, 1. doi:10.3389/fphar.2017.00001
Restifo, N. P., Dudley, M. E., and Rosenberg, S. A. (2012). Adoptive immunotherapy for cancer: harnessing the T cell response. Nat. Rev. Immunol. 12, 269–281. doi:10.1038/nri3191
Ribas, A., and Wolchok, J. D. (2018). Cancer immunotherapy using checkpoint blockade. Science. 3, 1350–1355. doi:10.1126/science.aar4060
Rodallec, A., Sicard, G., Fanciullino, R., Benzekry, S., Lacarelle, B., Milano, G., et al. (2018). Turning cold tumors into hot tumors: harnessing the potential of tumor immunity using nanoparticles. Expet Opin. Drug Metabol. Toxicol. 14, 1139–1147. doi:10.1080/17425255.2018.1540588
Roy Chowdhury, M., Schumann, C., Bhakta-Guha, D., and Guha, G. (2016). Cancer nanotheranostics: strategies, promises and impediments. Biomed. Pharmacother. 84, 291–304. doi:10.1016/j.biopha.2016.09.035
Ruter, J., Barnett, B. G., Kryczek, I., Brumlik, M. J., Daniel, B. J., Coukos, G., et al. (2009). Altering regulatory T cell function in cancer immunotherapy: a novel means to boost the efficacy of cancer vaccines. Front. Biosci. 14, 1761–1770. doi:10.2741/3338
Sabado, R. L., Balan, S., and Bhardwaj, N. (2017). Dendritic cell-based immunotherapy. Cell Res. 27, 74–95. doi:10.1038/cr.2016.157
Samanta, K., Setua, S., Kumari, S., Jaggi, M., Yallapu, M. M., and Chauhan, S. C. (2019). Gemcitabine combination nano therapies for pancreatic cancer. Pharmaceutics. 11. doi:10.3390/pharmaceutics11110574
Sancey, L., Kotb, S., Truillet, C., Appaix, F., Marais, A., Thomas, E., et al. (2015). Long-term in vivo clearance of gadolinium-based AGuIX nanoparticles and their biocompatibility after systemic injection. ACS Nano. 9, 2477–2488. doi:10.1021/acsnano.5b00552
Sancey, L., Lux, F., Kotb, S., Roux, S., Dufort, S., Bianchi, A., et al. (2014). The use of theranostic gadolinium-based nanoprobes to improve radiotherapy efficacy. Br. J. Radiol. 87, 20140134. doi:10.1259/bjr.20140134
Sarkar, S., Alam, M. A., Shaw, J., and Dasgupta, A. K. (2013). Drug delivery using platelet cancer cell interaction. Pharm Res. 30, 2785–2794. doi:10.1007/s11095-013-1097-1
Sau, S., Alzhrani, R., Bhise, K., Alsaab, H. O., Kashaw, S. K., and Iyer, A. K. (2019). Nanomaterials for tumor immunomodulation and overcoming current clinical challenges. Nanomedicine. 06 (14), 1515–1519
Sayes, C. M., Liang, F., Hudson, J. L., Mendez, J., Guo, W., Beach, J. M., et al. (2006). Functionalization density dependence of single-walled carbon nanotubes cytotoxicity in vitro. Toxicol. Lett. 161, 135–142. doi:10.1016/j.toxlet.2005.08.011
Schön, M. P., and Erpenbeck, L. (2018). The Interleukin-23/Interleukin-17 Axis links adaptive and innate immunity in psoriasis. Front. Immunol. 9, 1323
Schreiber, R. D., Old, L. J., and Smyth, M. J. (2011). Cancer immunoediting: integrating immunity's roles in cancer suppression and promotion. Science 331, 1565–1570. doi:10.1126/science.1203486
Shankaran, V., Ikeda, H., Bruce, A. T., White, J. M., Swanson, P. E., Old, L. J., et al. (2001). IFNgamma and lymphocytes prevent primary tumour development and shape tumour immunogenicity. Nature 410, 1107–1111. doi:10.1038/35074122
Shi, Y., van der Meel, R., Chen, X., and Lammers, T. (2020). The EPR effect and beyond: strategies to improve tumor targeting and cancer nanomedicine treatment efficacy. Theranostics 10, 7921–7924. doi:10.7150/thno.49577
Shukla, S., and Steinmetz, N. F. (2016). Emerging nanotechnologies for cancer immunotherapy. Exp. Biol. Med. 241, 1116–1126. doi:10.1177/1535370216647123
Simpson, J. D., Smith, S. A., Thurecht, K. J., and Such, G. (2019). Engineered polymeric materials for biological applications: overcoming challenges of the bio-nano Interface Polymers 11 (9), 1441. doi:10.3390/polym11091441
Singh, A., and Peppas, N. A. (2014). Hydrogels and scaffolds for immunomodulation. Adv Mater Weinheim 26, 6530–6541. doi:10.1002/adma.201402105
Singh, M. R. (2018). Application of metallic nanomaterials in nanomedicine. Adv. Exp. Med. Biol. 1052, 83–102. doi:10.1007/978-981-10-7572-8_8
Smalley Rumfield, C., Pellom, S. T., Morillon, Y. M., Schlom, J., and Jochems, C. (2020). Immunomodulation to enhance the efficacy of an HPV therapeutic vaccine. J. Immunother. Cancer 6, 8. doi:10.1136/jitc-2020-000612
Smyth, M. J., Dunn, G. P., and Schreiber, R. D. (2006). Cancer immunosurveillance and immunoediting: the roles of immunity in suppressing tumor development and shaping tumor immunogenicity. Adv. Immunol. 90, 1–50. doi:10.1016/S0065-2776(06)90001-7
Song, F., Li, X., Wang, Q., Liao, L., and Zhang, C. (2015). Nanocomposite hydrogels and their applications in drug delivery and tissue engineering. J. Biomed. Nanotechnol. 11, 40–52. doi:10.1166/jbn.2015.1962
Song, S., Jin, X., Zhang, L., Zhao, C., Ding, Y., Ang, Q., et al. (2019). PEGylated and CD47-conjugated nanoellipsoidal artificial antigen-presenting cells minimize phagocytosis and augment anti-tumor T-cell responses. Int. J. Nanomed. 14, 2465–2483. doi:10.2147/IJN.S195828
Suk, J. S., Xu, Q., Kim, N., Hanes, J., and Ensign, L. M. (2016). PEGylation as a strategy for improving nanoparticle-based drug and gene delivery. Adv. Drug Deliv. Rev. 99, 28–51. doi:10.1016/j.addr.2015.09.012
Sun, J. C., and Lanier, L. L. (2009). Natural killer cells remember: an evolutionary bridge between innate and adaptive immunity?. Eur. J. Immunol. 39, 2059–2064. doi:10.1002/eji.200939435
Sun, T., Zhang, Y. S., Pang, B., Hyun, D. C., Yang, M., and Xia, Y. (2014). Engineered nanoparticles for drug delivery in cancer therapy. Angew Chem. Int. Ed. Engl. 53, 12320–12364. doi:10.1002/anie.201403036
Sun, W., Li, S., Tang, G., Luo, Y., Ma, S., Sun, S., et al. (2019). Recent progress of nanoscale metal-organic frameworks in cancer theranostics and the challenges of their clinical application. Int. J. Nanomed. 14, 10195–10207. doi:10.2147/IJN.S230524
Swann, J. B., and Smyth, M. J. (2007). Immune surveillance of tumors. J. Clin. Invest. 117, 1137–1146. doi:10.1172/JCI31405
Sykes, E. A., Dai, Q., Sarsons, C. D., Chen, J., Rocheleau, J. V., Hwang, D. M., et al. (2016). Tailoring nanoparticle designs to target cancer based on tumor pathophysiology. Proc. Natl. Acad. Sci. USA 113, E1142–E1151. doi:10.1073/pnas.1521265113
Symowski, C., and Voehringer, D. (2017). Interactions between innate lymphoid cells and cells of the innate and adaptive immune system. Front. Immunol. 8, 1422. doi:10.3389/fimmu.2017.01422
Takeuchi, O., and Akira, S. (2010). Pattern recognition receptors and inflammation. Cell 140, 805–820. doi:10.1016/j.cell.2010.01.022
Tan, J., and Saltzman, W. M. (2004). Biomaterials with hierarchically defined micro- and nanoscale structure. Biomaterials. 25, 3593–3601. doi:10.1016/j.biomaterials.2003.10.034
Tan, P., He, L., Han, G., and Zhou, Y. (2017). Optogenetic immunomodulation: shedding light on antitumor immunity. Trends Biotechnol. 35, 215–226. doi:10.1016/j.tibtech.2016.09.002
Tan, Y. Y., Yap, P. K., Xin Lim, G. L., Mehta, M., Chan, Y., Ng, S. W., et al. (2020). Perspectives and advancements in the design of nanomaterials for targeted cancer theranostics. Chem. Biol. Interact. 329, 109221. doi:10.1016/j.cbi.2020.109221
Tang, W., Fan, W., Lau, J., Deng, L., Shen, Z., and Chen, X. (2019). Emerging blood-brain-barrier-crossing nanotechnology for brain cancer theranostics. Chem. Soc. Rev. 48, 2967–3014. doi:10.1039/c8cs00805a
Tay, M. Z., Poh, C. M., Rénia, L., MacAry, P. A., and Ng, L. F. P. (2020). The trinity of COVID-19: immunity, inflammation and intervention. Nat. Rev. Immunol. 06, 363–374.
Teradal, N. L., and Jelinek, R. (2017). Carbon nanomaterials in biological studies and biomedicine. Adv. Healthc. Mater. 6, 6. doi:10.1002/adhm.201700574
Tian, F., Cui, D., Schwarz, H., Estrada, G. G., and Kobayashi, H. (2006). Cytotoxicity of single-wall carbon nanotubes on human fibroblasts. Toxicol. In Vitro. 20, 1202–1212. doi:10.1016/j.tiv.2006.03.008
Tomar, N., and De, R. K. (2014). A brief outline of the immune system. Methods Mol. Biol. 1184, 3–12. doi:10.1007/978-1-4939-1115-8_1
Tran, T. H., Mattheolabakis, G., Aldawsari, H., and Amiji, M. (2015). Exosomes as nanocarriers for immunotherapy of cancer and inflammatory diseases. Clin. Immunol. 160, 46–58. doi:10.1016/j.clim.2015.03.021
Turjeman, K., Bavli, Y., Kizelsztein, P., Schilt, Y., Allon, N., Katzir, T. B., et al. (2015). Nano-drugs based on nano sterically stabilized liposomes for the treatment of inflammatory neurodegenerative diseases. PLoS One. 10, e0130442. doi:10.1371/journal.pone.0130442
Turvey, S. E., and Broide, D. H. (2010). Innate immunity. J. Allergy Clin. Immunol. 125, S24–S32. doi:10.1016/j.jaci.2009.07.016
Valenta, R., Mittermann, I., Werfel, T., Garn, H., and Renz, H. (2009). Linking allergy to autoimmune disease. Trends Immunol. 30, 109–116. doi:10.1016/j.it.2008.12.004
Vieira, D. B., and Gamarra, L. F. (2016). Getting into the brain: liposome-based strategies for effective drug delivery across the blood-brain barrier. Int. J. Nanomed. 11, 5381–5414. doi:10.2147/IJN.S117210
Waldhauer, I., and Steinle, A. (2008). NK cells and cancer immunosurveillance. Oncogene. 27, 5932–5943. doi:10.1038/onc.2008.267
Wang, H., Franco, F., and Ho, P. C. (2017). Metabolic regulation of Tregs in cancer: opportunities for immunotherapy. Trends Cancer 3, 583–592. doi:10.1016/j.trecan.2017.06.005
Wang, J., Sun, P., Bao, Y., Liu, J., and An, L. (2011). Cytotoxicity of single-walled carbon nanotubes on PC12 cells. Toxicol. In Vitro. 25, 242–250. doi:10.1016/j.tiv.2010.11.010
Wang, X., Wang, Y., Chen, Z. G., and Shin, D. M. (2009). Advances of cancer therapy by nanotechnology. Cancer Res. Treat. 41, 1–11. doi:10.4143/crt.2009.41.1.1
Weber, J. S., Yang, J. C., Atkins, M. B., and Disis, M. L. (2015). Toxicities of immunotherapy for the practitioner. J. Clin. Oncol. 33, 2092–2099. doi:10.1200/JCO.2014.60.0379
Wertel, I., Polak, G., Barczyński, B., and Kotarski, J. (2007). Subpopulations of peripheral blood dendritic cells during chemotherapy of ovarian cancer. Ginekol. Pol. 78, 768–771.
Whiteside, T. L. (2008). The tumor microenvironment and its role in promoting tumor growth. Oncogene 27, 5904–5912. doi:10.1038/onc.2008.271
Wing, E. J., and Remington, J. S. (1977). Cell-mediated immunity and its role in resistance to infection. West. J. Med. 126, 14–31.
Xin, Y., Huang, M., Guo, W. W., Huang, Q., Zhang, L. Z., and Jiang, G. (2017). Nano-based delivery of RNAi in cancer therapy. Mol Cancer. 16, 134. doi:10.1186/s12943-017-0683-y
Xu, B., Cui, Y., Wang, W., Li, S., Lyu, C., Wang, S., et al. (2020). Immunomodulation-enhanced nanozyme-based tumor Catalytic therapy. Adv Mater. 32, e2003563. doi:10.1002/adma.202003563
Xu, C. M., Tang, M., Feng, J., Xia, H. F., Wu, L. L., Pang, D. W., et al. (2020). A liquid biopsy-guided drug release system for cancer theranostics: integrating rapid circulating tumor cell detection and precision tumor therapy. Lab Chip. 04, 1418–1425. doi:10.1039/D0LC00149J
Xu, W., Atkins, M. B., and McDermott, D. F. (2020). Checkpoint inhibitor immunotherapy in kidney cancer. Nat. Rev. Urol. 3, 137–150. doi:10.1038/s41585-020-0282-3
Yang, G., Liu, Y., Wang, H., Wilson, R., Hui, Y., Yu, L., et al. (2019). Bioinspired core-shell nanoparticles for hydrophobic drug delivery. Angew Chem. Int. Ed. Engl. 58, 14357–14364. doi:10.1002/anie.201908357
Yang, J., and Zhang, C. (2020). Regulation of cancer-immunity cycle and tumor microenvironment by nanobiomaterials to enhance tumor immunotherapy. Wiley Interdiscip. Rev. Nanomed. Nanobiotechnol. 12, e1612.
Yoshioka, Y., Kuroda, E., Hirai, T., Tsutsumi, Y., and Ishii, K. J. (2017). Allergic responses induced by the immunomodulatory effects of nanomaterials upon skin exposure. Front. Immunol. 8, 169. doi:10.3389/fimmu.2017.00169
You, Y., Wang, N., He, L., Shi, C., Zhang, D., Liu, Y., et al. (2019). Designing dual-functionalized carbon nanotubes with high blood-brain-barrier permeability for precise orthotopic glioma therapy. Dalton Trans. 48, 1569–1573. doi:10.1039/c8dt03948h
Yu, W., Sun, J., Liu, F., Yu, S., Hu, J., Zhao, Y., et al. (2020). Treating immunologically cold tumors by precise cancer photoimmunotherapy with an extendable nanoplatform. ACS Appl. Mater. Interfaces 12, 40002–40012. doi:10.1021/acsami.0c09469
Zacharski, L. R., and Sukhatme, V. P. (2005). Coley's toxin revisited: immunotherapy or plasminogen activator therapy of cancer?. J. Thromb. Haemostasis 3, 424–427. doi:10.1111/j.1538-7836.2005.01110.x
Zhai, M., Xu, Y., Zhou, B., and Jing, W. (2018). Keratin-chitosan/n-ZnO nanocomposite hydrogel for antimicrobial treatment of burn wound healing: Characterization and biomedical application. J. Photochem. Photobiol. B. 180, 253–258. doi:10.1016/j.jphotobiol.2018.02.018
Zhang, Y., Cui, Z., Kong, H., Xia, K., Pan, L., Li, J., et al. (2016). One-shot immunomodulatory nanodiamond agents for cancer immunotherapy. Adv. Mater. Weinheim 28, 2699–2708. doi:10.1002/adma.201506232
Zhang, Y., Xu, Y., Li, Z., Chen, T., Lantz, S. M., Howard, P. C., et al. (2011). Mechanistic toxicity evaluation of uncoated and PEGylated single-walled carbon nanotubes in neuronal PC12 cells. ACS Nano 5, 7020–7033. doi:10.1021/nn2016259
Keywords: cancer, nanomaterials, immunomodulation, therapeutics, immunotherapy
Citation: Jindal A, Sarkar S and Alam A (2021) Nanomaterials-Mediated Immunomodulation for Cancer Therapeutics. Front. Chem. 9:629635. doi: 10.3389/fchem.2021.629635
Received: 15 November 2020; Accepted: 18 January 2021;
Published: 23 February 2021.
Edited by:
Subrata Kumar Pore, Ministry of Science and Technology (India), IndiaReviewed by:
Yevgeny Brudno, University of North Carolina at Chapel Hill, United StatesAmit Ranjan Maity, Amity University Kolkata, India
Copyright © 2021 Jindal, Sarkar and Alam. This is an open-access article distributed under the terms of the Creative Commons Attribution License (CC BY). The use, distribution or reproduction in other forums is permitted, provided the original author(s) and the copyright owner(s) are credited and that the original publication in this journal is cited, in accordance with accepted academic practice. No use, distribution or reproduction is permitted which does not comply with these terms.
*Correspondence: Aftab Alam, maa96@cam.ac.uk, alam.camb@gmail.com