Enovoltaics: Symbiotic integration of photovoltaics in vineyards
- Department of Applied Physics and Naval Technology, Technical University of Cartagena (UPCT), Cartagena, Spain
In this study, we propose the symbiotic integration of photovoltaic (PV) systems into previously built vineyards structures, so as to reduce land intervention, visual impact and costs while suppressing impact over crop production and quality. For this purpose, we have identified grape trellises as an ideal crop type to implement this concept based on a simulation study that analyzes the shading patterns and the PV energy generation of different PV design configurations using Photovoltaic Geographical Information System’s solar radiation data and system performance tool. Our proposal consists in the vertical integration of photovoltaic surfaces over the vines, using the same trellis structure, therefore minimizing cost and land building. We found that a ratio between row distance and trellis height equal or greater than 1.5 allows for the PV integration without generating significant shadowing between consecutive lines, while vertical orientation of the panels allows complete irradiation to the plants below. Different module configurations have been proposed and evaluated, resulting in a range of 40–60% installable capacity (compared to a ground mounted installation) with negligible shadowing over the leaves and grapes. Land equivalent ratio for the proposed architectures ranges between 1.27–1.50, therefore confirming the viability of this proposed agrivoltaic solution.
1 Introduction
Worldwide Photovoltaic (PV) installed capacity has grown exponentially during the last decade (IRENA, 2020) driven by the need for tackling the current challenges of increasing world energy demand, climate change and environmental sustainability. The transition towards a climate neutrality, crystallized in political initiatives such as the European Green Deal (European Commission, 2019), which promotes a reduction of 55% in greenhouse emissions by 2030 and climate neutrality by 2050, include a significative promotion of renewable energies deployment.
In this context of urgent development, extensive ground-mounted photovoltaic power plants (installed capacity >1MWp) where the maximization of energy production per land surface (kWh/m2/year) is sought out represent an economically viable option with attractive profits (Vartiainen et al., 2020). Consequently, this type of installations is spreading towards a wide variety of locations and ecosystems, creating a competence for the land use and increasing the pressure on agricultural activities (Hernandez et al., 2014). Moreover, decreasing profits in agriculture sector motivate farmers to abandon their activity in favor of a more profitable land renting or selling for photovoltaic use (Gazheli and Di Corato, 2013). A photovoltaic development model based on an extensive use of land concept, where food production is substituted by energy generation may probably be the cause of several problems instead of any solution (social rejection, food price increase, food scarcity, etc.) (Späth, 2018; Sargentis et al., 2021).
As a response to this use of land conflict, new approaches considering integrated perspectives have arisen: agrivoltaics, agrophotovoltaics, solar sharing, agri-PV or agrisolar are some of the terms used to refer to a same concept: shared location for photovoltaic technology and agricultural production (Toledo and Scognamiglio, 2021). This concept was firstly introduced by Goetzberger and Zastrow in 1981 (Goetzberger and Zastrow, 1981). These researchers proposed an elevated structure (2 m) and enough inter-panel distance (at least three times the module height) so as to obtain a uniform irradiance on the floor and at the same time allow free machinery movement. In 2005, Japanese engineer Akira Nagashima developed the first industrial system under this concept using a pergola-like structure (Nagashima, 2005), and from 2011 several groups have developed proof-of-concept systems exploring their potential under different perspectives: from land productivity increase, estimated between 35–70% (Dupraz et al., 2011), to economic land value over 30% (Dinesh and Pearce, 2016), water savings by 14–29% (Marrou et al., 2013), or social acceptance (Ketzer et al., 2020).
When trying to describe the challenges associated to agrivoltaics, probably the term solar sharing is the most descriptive way. Sharing the solar resource to simultaneously produce food and energy implies that the design of the photovoltaic system cannot always follow a standard approach where panels orientation is intended to optimize energy production, and the system design could come into conflict with an optimized food production (Toledo and Scognamiglio, 2021; Trommsdorff et al., 2021). Therefore, system adaptations to local climate, crop type or land shape must be implemented. Different solutions have been carried out attending to specific crops. Design solutions are commonly classified based on their application (crop production or livestock farming (Maia et al., 2020)), kind of system (open-field PV or PV greenhouse (Yano and Cossu, 2019)), type of structure (interspace PV (Imran and Riaz, 2021) or elevated PV (Dupraz et al., 2011; Amaducci et al., 2018; Trommsdorff et al., 2021)) or PV technology (fixed, dynamic (Valle et al., 2017), semitransparent (Gorjian et al., 2022), selective-wavelength devices (Loik et al., 2017), etc.). All proposed solutions seek to find a balance between tolerable shade and energy production, which depends mainly on plant species and climate. A balance between both productions must generally be achieved, where not the maximum energy per area is generated and the agricultural production may be lowered to some extent whether in quality or quantity, although in some cases panel shading can be beneficial for crop growth (Hassanpour Adeh et al., 2018; Sekiyama and Nagashima, 2019; El Kolaly et al., 2020). Most studies found inhibitory effects on plant growth with more than 50% PV cover rate, while no significant effects on plant growth and quality with a PV coverage rate of equal to or less than 25% (average yield reduction of less than 25%) with the exception of specific crops like strawberry and spinach, which performed better at 50% and 60% PV cover rate (Touil et al., 2021).
In most of the cases (except those in which land is previously divided into differentiated areas for panels and crop) an additional structure for PV panels is needed. Although necessary, this implies additional costs that in some cases may reduce or even compromise the economic viability of the system (Fraunhofer ISE, 2020). Not only it has an economic impact, but even more importantly, it may have a strong visual and environment impact (in terms of materials use, soil degradation due to construction stage, etc.). For instance, an integrated solution as a 222 kWp PV parking lot requires 72 t of steel, compared to 36 t of galvanized steel used in a conventional PV mounted system, which produces eight times more CO2 emissions (Serrano-Luján et al., 2015).
Among the different crops studied, grapes appear as an infrequent one. Few academic studies can be found about agrivoltaics implementation on vineyards. The effects on grape growth and energy production in a rain-hit-protection facility PV integrated installation, where panels coverture was restricted to 30% were analyzed (Cho et al., 2020). A slight decrease in grapes quality was found (sugar content and grape weight), although normal quality levels could be restored by a 10-days harvesting delay. In a modelled techno-economical study (Malu et al., 2017), found substantial economic benefits in a trellis-based vineyard, where PV panels were proposed to be horizontally mounted alternatively in the interspace between lines. The study did not take into consideration any biological aspect. Both studies make use of the interspace between vine lines, that appears in a trellis-based vineyard, to implement PV structures or manage the produced shadow. This type of trained vine growth structures is spread worldwide, due to the benefits regarding maintenance, harvesting facility or maturation homogeneity, among others.
On the commercial side, several pilot plants are being developed and several companies are focusing on vineyard agrivoltaics (Sun Agri, 2021). In these cases, the implemented solution consists in elevated (4–5 m) dynamic structures where the shadowing over the vineyard is controlled, promoting protection against climate conditions (excessive irradiation as well as hailstones). However, the cost associated to these structures is high. Similar structures have been reported to have estimated costs that may exceed six times those of a conventional floor mounted installation (405 €/kWp compared to 65 €/kWp (Schindele et al., 2020)) which may represent a limitation to their commercial deployment, preventing farmers to adopt this solution due to the high initial investment.
In this study, we propose a symbiotic integration of the PV system into previously built agricultural structures, so as to reduce land intervention, visual impact and costs while minimizing impact over crop production and quality. Grape trellises is as an ideal crop type to implement this concept. In these vineyards, vines are evenly distributed along a supporting structure consisting in metallic wires, and at the same time organized in lines with the adequate separation to allow for maintenance and in some cases mechanical harvesting. Our proposal consists in the vertical integration of photovoltaic surfaces over the vines, using the same trellis structure, therefore minimizing cost and land building. Consequently, the trellis structure plays a dual role of vine training and PV supporting structure. Distance between lines allows for the PV integration without generating significant shadowing between consecutive lines, while vertical orientation of the panels allows complete irradiation to the plants below. With this concept, competition for the solar resource is minimized and both uses, farming and energy production, are allowed.
2 Proposed systems
The proposed agrivoltaic solution considers the solar modules mounted above the grape trellises. Posts and wires provide support for vines as well as PV array support and cabling. A shadow analysis is performed to determine which PV useful area is available on the upper side of the structure without no conflict between grape production and solar modules projected shadow. Silicon-based PV technology is taken as the reference because it is consolidated at market level thanks to its efficiency and stability. At present, majority of silicon PV wafers are produced with side lengths of 156 mm (Saga, 2010), hence simulations were carried out considering multiples of 200 mm (considering minimum encapsulation for 1-cell unit size). The layout trellis dimensions are based on a trellised vineyard located in the experimental farm of the Technical University of Cartagena (Spain), Tomas Ferro (37.6889, -0.9513); these dimensions are representative for vertical trained vineyards management in the region (see Figures 1–A). The geometric characteristics for the simulation are described by the inter-row distance (d), the trellis height (L), and the PV module height (l). To perform the analysis, PVGIS v5.2 (European Commission, 2020) hourly data is used considering the different components of radiation, that is, beam (B), diffuse (D) and reflected (R) as well as the sun elevation angle. There are numerous specific software tools for PV system modeling and performance estimation; the characteristics of each program are commonly based on what type of solar radiation databases can be used, PV product libraries, inputs required, shadow modeling, electrical losses, and other features (González-Peña et al., 2021). PVGIS is well-known for its ease of use and accessibility. It offers a highly complete solar radiation database in Europe, Asia, Africa and South America that was obtained by satellite and includes data from 2005 to 2020, with an intuitive interface and few input parameters required. Furthermore, its reliability has been validated in numerous studies. However, it lacks a database of PV-related products, restricting detailed modeling of the installation (e.g., defining distribution of PV panels or connection with the inverter), which is primarily considered in other subscription PV tools. Nonetheless, PVGIS provides free an open access to full time series of hourly values of both energy yield and solar radiation, including Sun elevation angle, allowing for system performance analysis and shadow projection based on geometrical calculations. Although border effects cannot be considered under this approach, the results obtained using this database enable the development of a comparative analysis of different PV configurations. The incident irradiance (G) on the trellis height L is evaluated thought the shadow projection from the solar module to the next row which determines the module height limit. As sampling points, different heights along the trellis are considered; if the reference point is shaded, the incident irradiance is the sum of diffuse and reflected components; otherwise, it is global irradiance at the plane of the structure (See Figures 1–B).
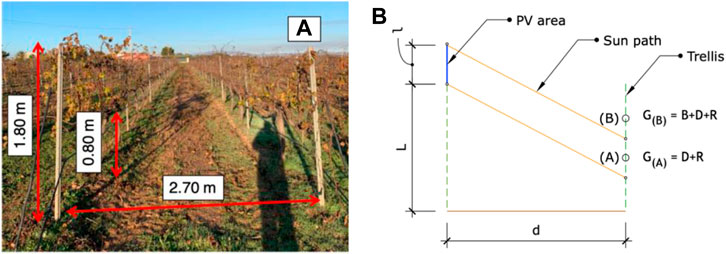
FIGURE 1. (A) Layout trellis dimensions from trellised vineyard located in the experimental farm of the Technical University of Cartagena (Spain). (B) Schematic arrangement of the agrivoltaic solution. Incident irradiance on the trellis is calculated based on the Sun path and irradiation components.
3 Analysis
3.1 PV available space
As previously mentioned, the geometric characteristics of the experimental farm Tomas Ferro were chosen for simulation. Grape trellis are spaced 2.7 m (d), and the vineyard height structure reaches 1.8 m from the ground (L). Therefore, the ratio between row distance and trellis height takes the value of 1.5. As long as this ratio is maintained or enlarged, all the results presented in this section with regards to PV available space and shadowing can be extended to other vineyard configurations. The height limit of the solar module is tested at 0.2, 0.4, 0.6 and 0.8 m (l). The shadow projection is calculated for trellis rows-oriented east-west; therefore, the solar elevation angle will determine the length shadow. Figure 2 shows the schematic arrangement of the PV modules over the trellis and the Sun path at 10 a.m. (time with low elevation angle) in solstice and equinox dates for heights considered. It is noted that during the winter solstice, shadows will reach the trunk for a few days without shading the crop in most cases (0.2, 0.4 and 0.6 m).
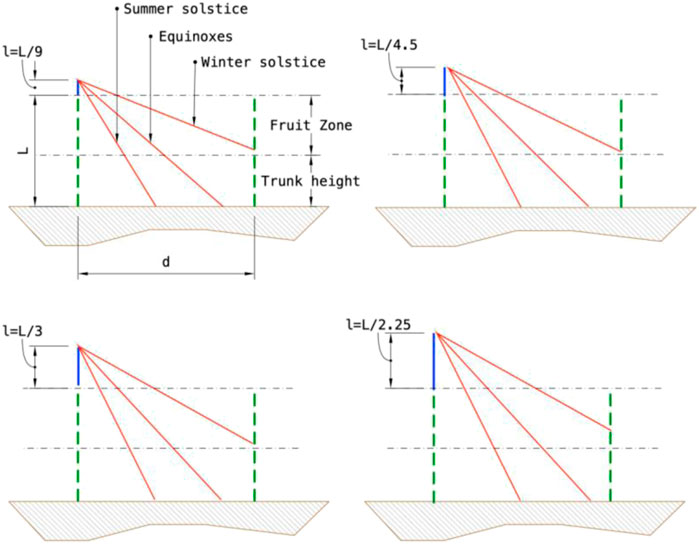
FIGURE 2. Shadow length projection in solstice and equinox dates at 10 a.m. and different PV heights. Top left l = L/9 (0.2 m), top right l = L/4.5 (0.4 m), bottom left l = L/3 (0.6 m) and bottom right l = L/2.25 (0.8 m).
Further analysis is performed, taking into account losses by shadows on annual basis. Python 3 was used for the analysis; the code is accessible in the Supplementary Material. Figure 3 shows annual irradiation on trellised vineyards at different heights. As can be seen, most of losses occur at heights less than 120 cm, which corresponds to the range of trunk heights in vineyard training systems (from 45 to 120 cm (Gutiérrez-Gamboa et al., 2021)). The height of the trunk at the reference experimental farm is 0.8 m, an average value that will serve as a reference for the analyses.
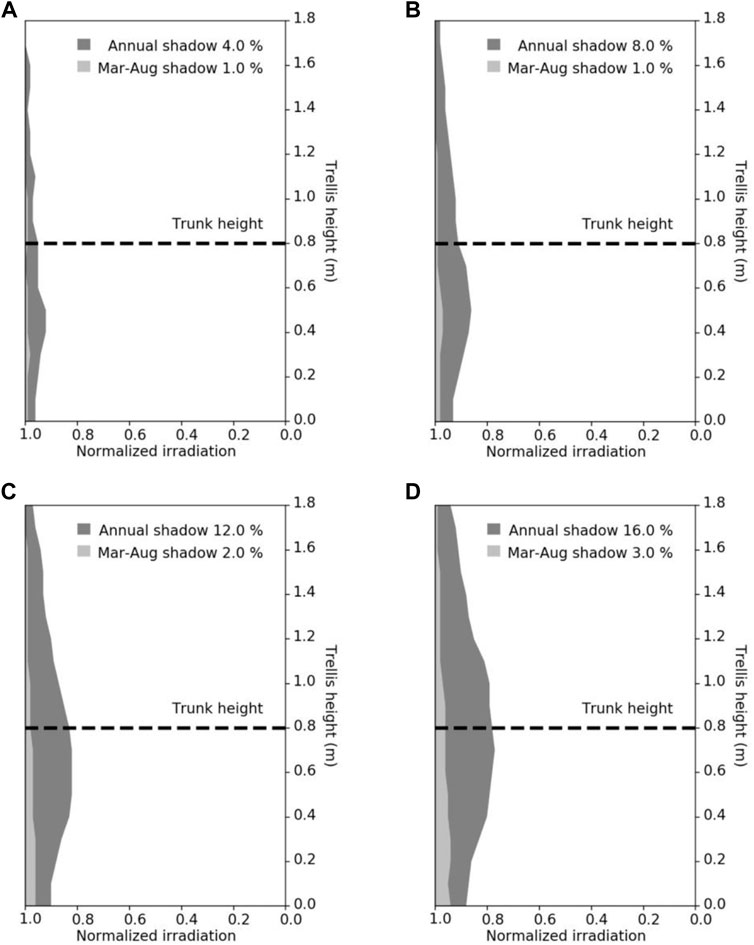
FIGURE 3. Normalized irradiation at different heights of the trellis (L) considering different PV area above the vineyard trellis (A) 0.2 m, (B) 0.4 m, (C) 0.6 m and (D) 0.8 m.
Figure 3 show the shadow losses over the trellis structure due to the PV installation for different heights, ranging from 4% for 0.2 m PV height to 16% for 0.8 m height. However, these values increase considerably due to the influence of the months with low solar elevation angles, which coincide with period of dormancy in Winter. Therefore, months from March to August are used as criteria, as they include the periods of leaf growth, flowering, berry ripening, and harvest. Under this criterion, for 0.6 and 0.8 m of installed PV height, shadows in the grape zone account for less than 2% and 3% respectively. If just the vine height above trunk (0.8 m), where the leaf area and fruit zone are located and the effect of shadows can have an impact on the crop is considered, annual irradiation losses decrease to less than 1% and 2% respectively.
3.2 PV design integration and energy generation
Different PV configurations are proposed in order to identify the optimal agrivoltaic design configuration by adjusting PV arrays in the available space above the vineyard trellis. As seen in the previous section, 0.6 m and 0.8 m PV structure heights on the upper side of the vineyard trellis structure guarantee less than 2% of annual losses irradiation in the crop zone for the critical period of the grape growth. To meet a symbiotic integration criterion, PV useful area of 0.6 m, i.e., one-third of the trellis height (which guarantees less than 1% of shadows) is selected for the analysis in this section. Two additional criteria were considered in the PV systems design: firstly, ensure easy working duties in the crop, specially harvesting. Mechanical harvesting as well as manual harvesting were considered. In this regard, as the PV systems are mounted above 1.80 m height, manual duties are allowed. Besides, a total height of 2.4 m (1.8 m trellis +0.6 m PV) guarantees a safe working area for most of the available harvesting machinery. In order to also allow a safe lateral working area, PV structures are limited to 40 cm (20 cm each side of the trellis). Secondly, when using multi-row PV configurations, row spacing and panel tilting is calculated in the basis of no shadowing between rows. Considering these criteria, five main topologies are proposed and analyzed: vertical mono-facial (V-MONO), vertical bi-facial (V-BI), venetian blind with optimized slat angle for energy generation (BLINDx2), venetian blind with modified slat angle to maximize installed capacity (BLINDx3) and roof type (ROOF). Each configuration is represented schematically in Figure 4.
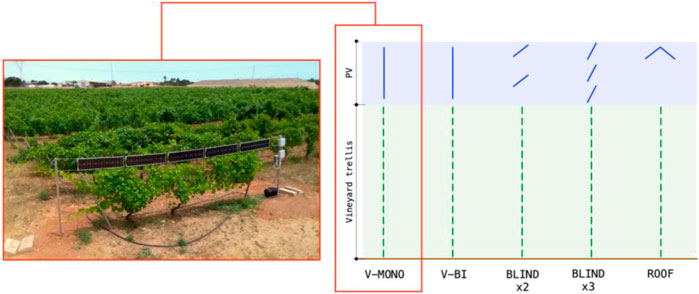
FIGURE 4. Schematic PV configurations proposed. Picture shows a proof-of-concept installation for one of the proposed configurations (V-MONO).
PV modules with 156 mm silicon PV wafers are used to set up each PV array. The efficiency of each PV module is considered 20% (NREL, 2022) at standard test condition (STC) which is representative of the average performance of this technology in the last decade. For vertical configurations, considering module dimensions of 1.4 m length and 0.6 m width, the PV module can accommodate 24 cells (3 rows and 8 columns, row width 0.6 m with room for 3 cells and extra space for encapsulation) with a nominal peak power of 117 W for mono-facial technology. Passivated emitter rear contact (PERC) technology is considered for bifacial PV. The bifaciality factor in this technology ranges from 0.7 to 0.8 (Gu et al., 2020). As input for the analysis, a bifaciality factor of 0.75 is taken. Venetian blind and roof configurations are based on units of 1 row and 8 cells. Each unit measures 1.4 m length and 0.3 m width (156 mm of cell plus an additional space for glass encapsulation) and a nominal peak power of 39 W. Blind systems have the advantage of reducing the impact of wind loads on the structure and allowing tilting so as to improve energy generation per installed power when compared to PV solutions mounted vertically. Therefore, PV blind configuration with two different slat angles is investigated: optimized slat angle that prioritizes energy generation, and modified slat angle calculated to ensure that the maximum installed capacity in the available space. In the first case, with a corresponding optimized tilt angle of 35°, and taking into account the maximum solar height corresponding to this latitude (75.7° of solar elevation angle, corresponding to the summer solstice at solar noon), just two modules can be placed avoiding shadows between adjacent units. In the second case, imposing three modules to maximize installed capacity, and the same maximum solar height, a maximum tilt angle of 81°, avoiding shadows between units, is obtained. Roof type configuration (double tilt mounted system) is set up with an optimized tilt angle, but due to the height constraints, only one unit by side can be placed without casting shadows on the grape beneath. Table 1 shows a summary of the technical specifications regarding module dimensions and tilt for each configuration. It also includes the installed capacity per hectare and a comparison to a conventional ground-mounted PV system (PV-GM), which have a land use that ranges from 1.3 to 2.5 ha/MW (NREL, 2013) depending on the size of the system. For comparison purposes, 2 ha/MW is set as reference.
The annual energy generated in one ha for each configuration is calculated using PVGIS energy production method. Since the potential of each design solution depends on the row vineyard orientation, values are obtained for nine different PV module orientations. Results are presented in Figure 5.
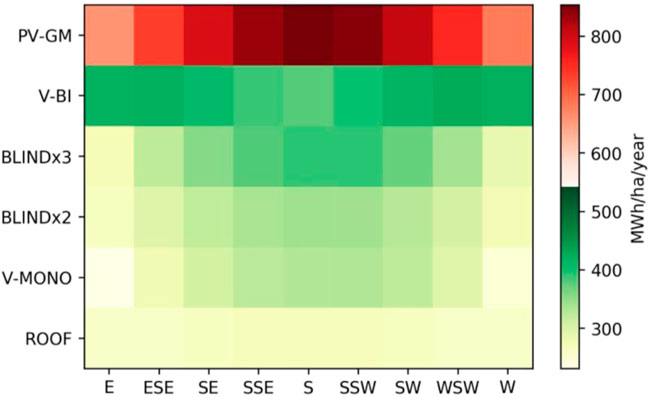
FIGURE 5. Annual energy generation per hectare for each configuration in different orientations (referring to the orientation of the PV module’s face).
When compared to ground mounted PV, expected lower energy generation is found for all trellis-integrated double-use-of-land configurations. A maximum of 500 MWh/ha/year is found for the best case (E-W oriented bifacial modules), as compared to 800 MWh/ha/year for single-use-of–land ground mounted PV (which corresponds to 63%). In terms of energy generation, bifacial technology is the most favorable solution with a range from 44% (azimuth 0° - south oriented) to the mentioned 63% (E-W orientation) when compared to the PV single use of land. Although the installed capacity is similar for V-MONO, V-BI and BLINDx3 configurations, the energy produced for every unit of capacity, the yield (kWh/kWp), differs mainly due to orientation and slope of each solution. Figure 6 shows the variation of specific yield as function of PV configuration and orientation. The maximum yield is found to be 1707 kWh/kWp for ground mounted PV facing equator, which corresponds to the BLINDx2 configuration with the same tilt and azimuth. For V-MONO configuration, the yield varies from 765 kWh/kWp to 1099 kWh/kWp.
Despite the obvious fact that the energy production of an integrated system cannot compete with a conventional design that prioritizes energy generation due to less installed capacity per area and not optimized tilt angle, a dual use of land approach may offer additional benefits when analyzed from a global perspective include both crop and energy productions. To quantify these benefits, the Land Equivalent Ratio (LER) (Dupraz et al., 2011; Trommsdorff et al., 2021) indicator is commonly used in agrivoltaic systems. It is defined as:
where Yagri denotes agricultural yield in a single use of land and Yagri-APV refers to the yield in an agrivoltaic system for the same area unit. YPV indicates electricity production under a standard PV system assumption, while YAPV represents the energy production of the agrivoltaic system. In the proposed solution, the agricultural yield is assumed to be not affected, therefore the agricultural ratio will be one, and for the second term, using the electricity production of ground mounted PV with optimal orientation and inclination as reference, LER intervals for all the proposed configurations and the different orientations analyzed, range from 1.27 (V-MONO facing east) to 1.50 (V-BI E-W) (see Figure 7). That means that in the worst-case scenario, a 100-ha agrivoltaic farm would produce the same amount of electricity and grape crop as a 100-ha conventional farm plus a 27-ha ground mounted optimized PV installation.
4 Prospects
The proposed integration of photovoltaic modules into grape trellis structures appears as an interesting approach for agrivoltaic solutions, when compared to commonly used elevated PV structures. On one side, installed capacity per ha for the trellis-integrated structures ranges between 40–60%, where similar or lower PV coverages are imposed for elevated structures due to shadowing restrictions. On the other side, integration of the PV modules into the trellis structure (directly or with simple reinforcements) seems to be an economically competitive approach. Ongoing efforts in pilot PV installations may shed some light in these regards in the near future. In any case, preliminary prospects on the potential of this solution seems promising. We considered the Region of Murcia (1131300 ha surface situated in the southeast of Spain) where the experimental farm used for this proposal is located, as a study case. This region counts with a total of three wine production areas with certificate of origin protection, among other areas which together account for a total of 29.000 ha of vineyards. Additionally, accumulated photovoltaic installed power in the region by 2019 was 1100 MW (IDAE, 2019).
Therefore, according to our results, available installed power for vineyards integrated PV would range from 5845 to 8768 MW, that is, between 5 and 8 times the total installed power in the Region of Murcia. This case illustrates the huge potential of this proposed agrivoltaic solution. Murcia is the Spanish region with the most installed PV capacity per km2, that is 98.5 kWp/km2, due to its high annual irradiation, and a clear example where recent massive PV plants installation is increasing pressure on agricultural activities (agriculture accounts for 41% of the region’s land area (Spanish Ministry of Agriculture, Fisheries and Food, 2019)). The proposed solution would help farmers relieve pressure by allowing them to stabilize their income without jeopardizing their main activity or creating land-use competition.
While results in terms of available energy production and possible installed capacity seem promising, a number of additional technical, economic and social aspects are opened for further study:
Demonstration projects to experimentally validate the results of this modeling study are desirable, so that the potential effects of partial shadowing from PV modules on yield and wine quality can be quantified. In addition, these projects will also serve to investigate other technical aspects and economic viability of the proposed system. This includes the assessment of the compatibility of the balance of system (BOS) with the use of conventional machinery and farming practices, e.g., PV arrays cabling interconnection among the trellises so that it can be compatible with machinery pass.
Detailed structural studies will shed some light on the needs for supporting additional weight created by the PV modules and wind loads. Reinforcement of the structure could be needed, for instance, thus leading to an increase of the cost for the implementation (e.g., The weight of glass-glass PV modules with 4 mm glass on each side is around 17 kg/m2 (ONYX solar, 2022) so, although bifacial technology presents better performance in this configuration, additional reinforcements could be needed in that case). Initial vineyard structures (metal or wood based, maintenance state, etc.) will play a significative role in the initial inversion and/or reinforcement needs.
Additionally, exploring interconnections among the various dimensions of social acceptance is another key factor for the future implementation of this solution. Installation of huge solar plants in land, where local communities are excluded from economic and labor benefits, is generating an increasing social rejection. This vineyard integrated solution, where energy production is distributed among different locations and owners, would allow the constitution of energy communities from existing farming cooperatives in locations with a high density of vineyards, which could encourage farmers to adopt this technology by improving local economic development. In this sense, it will be interesting to see how involving farmers in maintenance tasks (e.g., in the pruning process that will be necessary both for the vineyard and so that the shadows of the leaves do not cover the photovoltaic panel), which reinforces the symbiotic nature of this solution, could promote the broad adoption of this technology by aligning it with rural identity and interests.
5 Conclusion
Vertical integration of photovoltaic modules into vineyards trellis structures has been proposed as an effective agrivoltaic solution, reducing land intervention, visual impact and costs while suppressing impact over crop production and quality.
Distance between vineyards lines and vine height have been considered to propose a number of module configurations (including vertical, venetian blind and roof type) without generating significant shadowing between consecutive lines. One-third of the trellis height in a system with a ratio of 1.5 between inter-row distance and height of the trellis has been stablished as the maximum height to minimize shadowing.
Installable capacity per hectare and energy yield have been evaluated for five proposed module configurations and multiple orientations, resulting in a range between 44–63% energy generation compared to a ground mounted installation. Land equivalent ratio (LER) was calculated, and ranged between 1.27–1.50, confirming the viability of the proposed agrivoltaic solution. Moreover, comparing the limitations of photovoltaic coverage for elevated structures (the most frequently used configuration in vineyards), the proposed integrated solution appears as a viable alternative.
In summary, the proposed symbiotic integration of photovoltaic modules into a vineyard trellis structure (termed Enovoltaics) appears as a promising approach among agrovoltaics for dual land use where crop production is prioritized. Ongoing pilot installations will bring additional information about the benefits of this solution, while application to other crop training systems (olive or almond bushes, among others) could be envisioned.
Data availability statement
The raw data supporting the conclusion of this article will be made available by the authors, without undue reservation.
Author contributions
JP: Conceptualization, Investigation, Data Curation, Writing—Original Draft, CT: Methodology, Investigation, Formal analysis, Writing—Original Draft. JA: Investigation, Writing—Review and Editing.
Funding
This work was funded by Ministerio de Ciencia e Innovación-Agencia Estatal de Investigación (AEI-MICINN,Spain) Grant number PID 2019-104272RB-C55 and by Fundación Séneca (Región de Murcia) Grant 20985/PI/18. C. T. is grateful for his postdoctoral fellowship “Margarita Salas” as part of the Spanish University System requalification program funded by the Ministry of Universities with EU Next Generation funds.
Acknowledgments
Authors would like to thank to PVGIS for making their data freely available.
Conflict of interest
The authors declare that the research was conducted in the absence of any commercial or financial relationships that could be construed as a potential conflict of interest.
Publisher’s note
All claims expressed in this article are solely those of the authors and do not necessarily represent those of their affiliated organizations, or those of the publisher, the editors and the reviewers. Any product that may be evaluated in this article, or claim that may be made by its manufacturer, is not guaranteed or endorsed by the publisher.
Supplementary material
The Supplementary Material for this article can be found online at: https://www.frontiersin.org/articles/10.3389/fenrg.2022.1007383/full#supplementary-material
References
Amaducci, S., Yin, X., and Colauzzi, M. (2018). Agrivoltaic systems to optimise land use for electric energy production. Appl. Energy 220, 545–561. doi:10.1016/j.apenergy.2018.03.081
Cho, J., Park, S. M., Park, A. R., Lee, O. C., Nam, G., and Ra, I.-H. (2020). Application of photovoltaic systems for agriculture: A study on the relationship between power generation and farming for the improvement of photovoltaic applications in agriculture. Energies 13, 4815. doi:10.3390/en13184815
Dinesh, H., and Pearce, J. M. (2016). The potential of agrivoltaic systems. Renew. Sustain. Energy Rev. 54, 299–308. doi:10.1016/j.rser.2015.10.024
Dupraz, C., Marrou, H., Talbot, G., Dufour, L., Nogier, A., and Ferard, Y. (2011). Combining solar photovoltaic panels and food crops for optimising land use: Towards new agrivoltaic schemes. Renew. Energy 36, 2725–2732. doi:10.1016/j.renene.2011.03.005
El Kolaly, W., Ma, W., Li, M., and Darwesh, M. (2020). The investigation of energy production and mushroom yield in greenhouse production based on mono photovoltaic cells effect. Renew. Energy 159, 506–518. doi:10.1016/j.renene.2020.05.144
European Commission (2020). Photovoltaic geographical information system (PVGIS v5.2). Available at: http://re.jrc.ec.europa.eu/pvg_tools/en/tools.html (Accessed November 29, 2021).
European Commission (2019). The European green deal. Available at: https://ec.europa.eu/info/strategy/priorities-2019-2024/european-green-deal_en (Accessed May 13, 2022).
Fraunhofer, I. S. E. (2020). Agrivoltaics: Opportunities for agriculture and the energy transition. A guideline for Germany. Available at: https://www.ise.fraunhofer.de/en/publications/studies/agrivoltaics-opportunities-for-agriculture-and-the-energy-transition.html (Accessed August 30, 2022).
Gazheli, A., and Di Corato, L. (2013). Land-use change and solar energy production: A real option approach. Agric. Finance Rev. 73, 507–525. doi:10.1108/AFR-05-2012-0024
Goetzberger, A., and Zastrow, A. (1981). On the coexistence of solar-energy conversion and plant cultivation. Int. J. Sol. Energy 1, 55–69. doi:10.1080/01425918208909875
González-Peña, D., García-Ruiz, I., Díez-Mediavilla, M., Dieste-Velasco, M. I., and Alonso-Tristán, C. (2021). Photovoltaic prediction software: Evaluation with real data from northern Spain. Appl. Sci. 11, 5025. doi:10.3390/app11115025
Gorjian, S., Bousi, E., Özdemir, Ö. E., Trommsdorff, M., Kumar, N. M., Anand, A., et al. (2022). Progress and challenges of crop production and electricity generation in agrivoltaic systems using semi-transparent photovoltaic technology. Renew. Sustain. Energy Rev. 158, 112126. doi:10.1016/j.rser.2022.112126
Gu, W., Ma, T., Ahmed, S., Zhang, Y., and Peng, J. (2020). A comprehensive review and outlook of bifacial photovoltaic (bPV) technology. Energy Convers. Manag. 223, 113283. doi:10.1016/j.enconman.2020.113283
Gutiérrez-Gamboa, G., Zheng, W., and Martínez de Toda, F. (2021). Strategies in vineyard establishment to face global warming in viticulture: A mini review. J. Sci. Food Agric. 101, 1261–1269. doi:10.1002/jsfa.10813
Hassanpour Adeh, E., Selker, J. S., and Higgins, C. W. (2018). Remarkable agrivoltaic influence on soil moisture, micrometeorology and water-use efficiency. PLOS ONE 13, e0203256. doi:10.1371/journal.pone.0203256
Hernandez, R. R., Easter, S. B., Murphy-Mariscal, M. L., Maestre, F. T., Tavassoli, M., Allen, E. B., et al. (2014). Environmental impacts of utility-scale solar energy. Renew. Sustain. Energy Rev. 29, 766–779. doi:10.1016/j.rser.2013.08.041
IDAE (2019). Spanish statistical report on renewable energies. Available at: https://informesweb.idae.es/informe-estadistico/informe.php.
Imran, H., and Riaz, M. H. (2021). Investigating the potential of east/west vertical bifacial photovoltaic farm for agrivoltaic systems. J. Renew. Sustain. Energy 13, 033502. doi:10.1063/5.0054085
IRENA (2020). Solar energy data. Available at: https://www.irena.org/solar (Accessed May 13, 2022).
Ketzer, D., Weinberger, N., Rösch, C., and Seitz, S. B. (2020). Land use conflicts between biomass and power production – citizens’ participation in the technology development of Agrophotovoltaics. J. Responsible Innov. 7, 193–216. doi:10.1080/23299460.2019.1647085
Loik, M. E., Carter, S. A., Alers, G., Wade, C. E., Shugar, D., Corrado, C., et al. (2017). Wavelength-selective solar photovoltaic systems: Powering greenhouses for plant growth at the food-energy-water nexus. Earth's. Future 5, 1044–1053. doi:10.1002/2016EF000531
Maia, A. S. C., Culhari, E. de A., Fonsêca, V. de F. C., Milan, H. F. M., and Gebremedhin, K. G. (2020). Photovoltaic panels as shading resources for livestock. J. Clean. Prod. 258, 120551. doi:10.1016/j.jclepro.2020.120551
Malu, P. R., Sharma, U. S., and Pearce, J. M. (2017). Agrivoltaic potential on grape farms in India. Sustain. Energy Technol. Assessments 23, 104–110. doi:10.1016/j.seta.2017.08.004
Marrou, H., Dufour, L., and Wery, J. (2013). How does a shelter of solar panels influence water flows in a soil–crop system? Eur. J. Agron. 50, 38–51. doi:10.1016/j.eja.2013.05.004
Nagashima, A. (2005). Sunlight power generation system. Japan Patent No. 2005-277038 (Accessed October 6, 2005).
NREL (2022). Champion photovoltaic module efficiency chart. Photovoltaic research Available at: https://www.nrel.gov/pv/module-efficiency.html (Accessed May 13, 2022).
NREL (2013). Land use by system technology. Available at: https://www.nrel.gov/analysis/tech-size.html (Accessed July 20, 2022).
ONYX solar (2022). Technical guide, photovoltaic glass for buildings. Available at: https://www.onyxsolar.com/all-you-need (Accessed September 1, 2022).
Saga, T. (2010). Advances in crystalline silicon solar cell technology for industrial mass production. NPG Asia Mat. 2, 96–102. doi:10.1038/asiamat.2010.82
Sargentis, G.-F., Siamparina, P., Sakki, G.-K., Efstratiadis, A., Chiotinis, M., and Koutsoyiannis, D. (2021). Agricultural land or photovoltaic parks? The water–energy–food nexus and land development perspectives in the thessaly plain, Greece. Sustainability 13, 8935. doi:10.3390/su13168935
Schindele, S., Trommsdorff, M., Schlaak, A., Obergfell, T., Bopp, G., Reise, C., et al. (2020). Implementation of agrophotovoltaics: Techno-economic analysis of the price-performance ratio and its policy implications. Appl. Energy 265, 114737. doi:10.1016/j.apenergy.2020.114737
Sekiyama, T., and Nagashima, A. (2019). Solar sharing for both food and clean energy production: Performance of agrivoltaic systems for corn, A typical shade-intolerant crop. Environments 6, 65. doi:10.3390/environments6060065
Serrano-Luján, L., García-Valverde, R., Espinosa, N., García-Cascales, M. S., Sánchez-Lozano, J. M., and Urbina, A. (2015). Environmental benefits of parking-integrated photovoltaics: A 222 kWp experience. Prog. Photovolt. Res. Appl. 23, 253–264. doi:10.1002/pip.2415
Spanish Ministry of Agriculture, Fisheries and Food (2019). Encuesta sobre Superficies y Rendimientos de Cultivos (ESYRCE). Available at: https://www.mapa.gob.es/es/estadistica/temas/estadisticas-agrarias/agricultura/esyrce/.
Späth, L. (2018). Large-scale photovoltaics? Yes please, but not like this! Insights on different perspectives underlying the trade-off between land use and renewable electricity development. Energy Policy 122, 429–437. doi:10.1016/j.enpol.2018.07.029
Sun Agri (2021). Dynamic agrivoltaics. Key findings: Vine growing. Available at: https://sunagri.fr/en/key-findings-vine-growing/(Accessed December 23, 2021).
Toledo, C., and Scognamiglio, A. (2021). Agrivoltaic systems design and assessment: A critical review, and a descriptive model towards a sustainable landscape vision (Three-Dimensional agrivoltaic patterns). Sustainability 13, 6871. doi:10.3390/su13126871
Touil, S., Richa, A., Fizir, M., and Bingwa, B. (2021). Shading effect of photovoltaic panels on horticulture crops production: A mini review. Rev. Environ. Sci. Biotechnol. 20, 281–296. doi:10.1007/s11157-021-09572-2
Trommsdorff, M., Kang, J., Reise, C., Schindele, S., Bopp, G., Ehmann, A., et al. (2021). Combining food and energy production: Design of an agrivoltaic system applied in arable and vegetable farming in Germany. Renew. Sustain. Energy Rev. 140, 110694. doi:10.1016/j.rser.2020.110694
Valle, B., Simonneau, T., Sourd, F., Pechier, P., Hamard, P., Frisson, T., et al. (2017). Increasing the total productivity of a land by combining mobile photovoltaic panels and food crops. Appl. Energy 206, 1495–1507. doi:10.1016/j.apenergy.2017.09.113
Vartiainen, E., Masson, G., Breyer, C., Moser, D., and Román Medina, E. (2020). Impact of weighted average cost of capital, capital expenditure, and other parameters on future utility-scale PV levelised cost of electricity. Prog. Photovolt. Res. Appl. 28, 439–453. doi:10.1002/pip.3189
Keywords: agrivoltaics, solar PV, vineyard, energy, PV integration
Citation: Padilla J, Toledo C and Abad J (2022) Enovoltaics: Symbiotic integration of photovoltaics in vineyards. Front. Energy Res. 10:1007383. doi: 10.3389/fenrg.2022.1007383
Received: 30 July 2022; Accepted: 14 September 2022;
Published: 29 September 2022.
Edited by:
Isabel M. Moreno-Garcia, Universidad de Córdoba, SpainReviewed by:
Jesús Rodrigo-Comino, University of Granada, SpainDimitrios D. Piromalis, University of West Attica, Greece
Copyright © 2022 Padilla, Toledo and Abad. This is an open-access article distributed under the terms of the Creative Commons Attribution License (CC BY). The use, distribution or reproduction in other forums is permitted, provided the original author(s) and the copyright owner(s) are credited and that the original publication in this journal is cited, in accordance with accepted academic practice. No use, distribution or reproduction is permitted which does not comply with these terms.
*Correspondence: Javier Padilla, Javier.padilla@upct.es; Carlos Toledo, Carlos.toledo@upct.es
†These authors contributed equally to this work and share first authorship