- Department of Microbiology and Immunology, David H. Smith Center for Vaccine Biology and Immunology, University of Rochester, Rochester, NY, United States
Maintenance of homeostatic immune surveillance and development of effective adaptive immune responses require precise regulation of spatial and temporal lymphocyte trafficking throughout the body to ensure pathogen clearance and memory generation. Dysregulation of lymphocyte activation and migration can lead to impaired adaptive immunity, recurrent infections, and an array of autoimmune diseases and chronic inflammation. Central to the recruitment of T cells, integrins are cell surface receptors that regulate adhesion, signal transduction, and migration. With 24 integrin pairs having been discovered to date, integrins are defined not only by the composition of the heterodimeric pair but by cell-type specific expression and their ligands. Furthermore, integrins not only facilitate adhesion but also induce intracellular signaling and have recently been uncovered as mechanosensors providing additional complexity to the signaling pathways. Among several leukocyte-specific integrins, lymphocyte function-associated antigen-1 (LFA-1 or αLβ2; CD11a/CD18) is a key T cell integrin, which plays a major role in regulating T cell activation and migration. Adhesion to LFA-1’s ligand, intracellular adhesion receptor 1 (ICAM-1) facilitates firm endothelium adhesion, prolonged contact with antigen-presenting cells, and binding to target cells for killing. While the downstream signaling pathways utilized by LFA-1 are vastly conserved they allow for highly disparate responses. Here, we summarize the roles of LFA-1 and ongoing studies to better understand its functions and regulation.
Introduction
Precise spatial and temporal regulation of adhesion and de-adhesion is critical for immune cell development, localization, and pathogen clearance. LFA-1 is a key T cell integrin that plays a critical role in the regulation of these functions. With this highly diverse set of roles, it is unsurprising that LFA-1 has been implicated in numerous autoimmune and inflammatory conditions including inflammatory bowel disease, psoriasis, diabetes, and arthritis (1, 2). Intriguingly, intracellular signals dictating LFA-1 activation are highly conserved between migration, T cell activation, and cytolytic activity suggesting that any alterations in the signaling may cause substantial biological consequences during the host immune responses. This review will discuss our current understanding of the role of LFA-1 during T cell activation, effector functions, and memory formation.
LFA-1 Structure
LFA-1 is composed of α- and β- subunits that together form a heterodimer expressed at the cell surface. These subunits include long extracellular domains, a single transmembrane domain, and short cytoplasmic tails (Figure 1). The extension of LFA-1, which resembles a switchblade-like motion, requires substantial changes to the conformation of both subunits (3). LFA-1 has at least three separate conformational states that are conferred by movement of the extracellular and cytosolic domains: (1) closed/bent, where the integrin has low affinity for ligand and is conformationally unavailable to bind ligand; (2) closed/extended, where the integrin is extended allowing for interaction with ligand, but the cytosolic tails remain closed; and (3) open/extended, where the integrin has high affinity for its ligand and the cytosolic tails have separated (Figure 1i) (3–5).
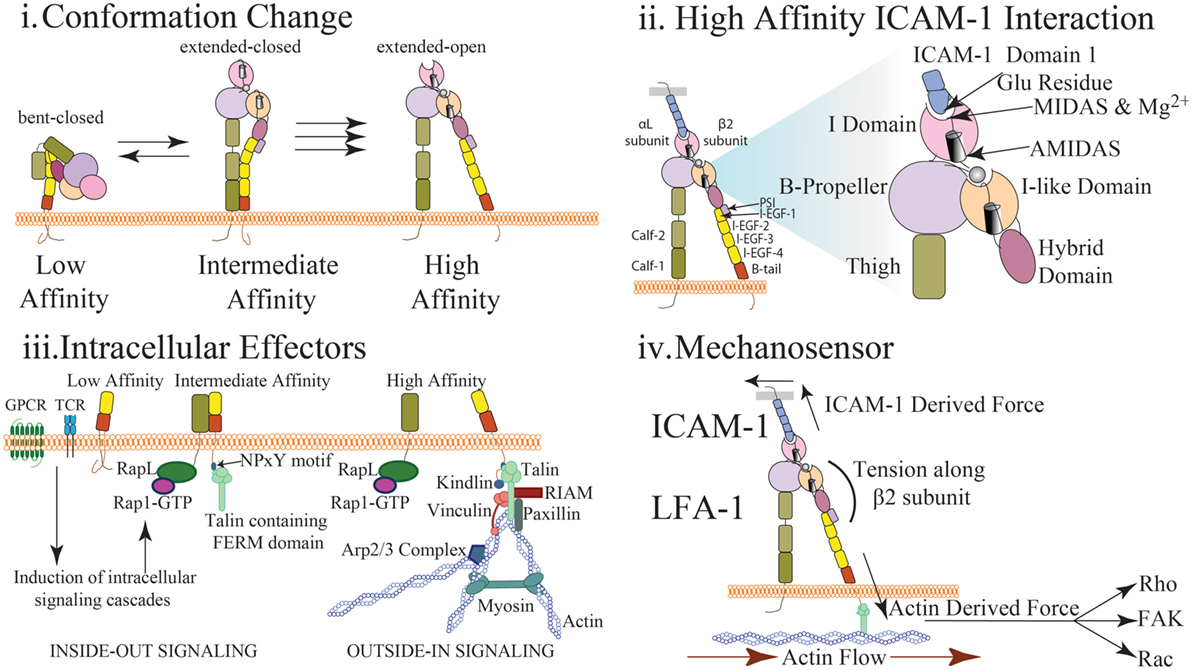
Figure 1. Multidimensional regulation of LFA-1 affinity (i) LFA-1 affinity regulation is mediated via conformational changes to LFA-1 structure. In the low affinity state, the bent conformation causes the ligand binding αI domain to be inaccessible to interact with ICAM-1. In the intermediate affinity state, the extracellular leg domains are straightened allowing for low affinity interactions between LFA-1 and ICAM-1. Importantly, the intracellular domains of LFA-1 are not separated and the metal ion-dependent adhesion site (MIDAS) binding site closed. In the high affinity state, disruption of the salt bridge between the α and β cytosolic tails results in conformational shift along the β subunit and αI domain resulting in high affinity LFA-1 via the opening of the ligand-binding site. (ii) The αI domain contains the MIDAS within which resides Mg2+ coordinating the binding pocket. This site interacts with the glutamic acid-34 in Domain 1 of ICAM-1 to facilitate binding. This induces a shift in the α7 helix to cause the hybrid domain to swing out further stabilizing LFA-1 structure. Additional sites surrounding the MIDAS such as AMIDAS and ligand-induced metal-binding site assist with coordination of the binding pocket and stabilization of high affinity LFA-1. (iii) Upon T cell receptor or chemokine activation, RAP1-GTP recruits a number of factors including RAPL that interact with the α subunit of LFA-1 to induce integrin activation (inside-out signaling). Similarly, talin cleavage allows the FERM domain to interact with the NPxY motif of the cytosolic tail on the β subunit. This interaction causes a dissociation of the salt bridge inducing cytosolic tail separation. Kindlin also contains a FERM domain and interacts with the β subunit to further stabilize high affinity LFA-1. Molecules such as RIAM, talin, paxillin, and vinculin may interact with the cytosolic tails to recruit additional effector molecules and promote a scaffold to interact with actin and reinforce LFA-1 activity (outside-in signaling). Arp2/3 will promote continued actin filament growth while MyH9 functions to provide stress on actin fibers to induce LFA-1 dissociation from ligand. (iv) Interaction of LFA-1 with ICAM-1 and β-actin allows for force driven responses along the β subunit. Transmission of force (arrows) along the β-subunit has been measured in pN scale with actin flow functioning to direct the orientation and location of LFA-1 both at the immunological synapse and during cell migration. Stabilization of the integrin in the high affinity conformation via force generation requires adhesion to both the cytoskeleton and ICAM-1. The stiffness of the substrate may also alter the level of force generated thus altering the signaling response. Downstream signal is induced via outside in signaling generated through the stabilization of high affinity LFA-1. Phosphorylation of focal adhesion kinase through force generation may play a role in mediating cell adhesion and proliferation. Rho signaling, and thus actin polymerization, may also be altered through changes in force generation resulting in changes in actin dynamics and cell migration. Induction of Rac and CDC42 may also be altered through force generation resulting in changes to cell proliferation and survival.
Roughly half of all integrins, including LFA-1, express an αI domain, which is critical for ligand binding and contains a metal ion-dependent adhesion site (MIDAS) that binds Mg2+ to coordinate the binding pocket (Figure 1ii) (3). ICAM-1 will directly bind with the LFA-1 MIDAS and Mg2+ by interacting with a glutamic acid residue found in Domain 1 of ICAM-1 (Figure 1ii) (6). LFA-1 is also capable of binding ICAM-2 and ICAM-3 albeit with much lower affinity. Two additional sites, ligand-induced metal-binding site (LIMBS) and adjacent to MIDAS (ADMIDAS), have been shown to regulate cytosolic tail separation and reduce cell spreading, respectively (7–9). Two domains on the α subunit leg, calf-1 and calf-2, have a Ca2+ binding loop that is critical to the subunit bending. The β subunit consists of the I-like domain, which is homologous to the αI domain and plays a key role in determining specificity. The hybrid domain, which connects the upper and lower portions of the β subunit, is critical for conformation change. The β subunit leg consists of a plexin/semaphorin/integrin domain that is connected to the βI domain and four integrin epidermal growth factor-like (I-EGF) domains, which facilitate β leg bending (Figure 1i–ii) (3, 5, 8–10).
To facilitate conformation changes, a number of structural modifications occur utilizing the abovementioned integrin components. As the α7-helix is displaced downward during integrin activation, the hybrid domain swings outward leading to separation of the extracellular legs (6). However, due to the flexibility of the extracellular β subunit leg, this separation facilitates only extension of the leg and does not separate the cytosolic tails. Complete cytosolic tail separation requires intracellular effectors, such as talin (Figure 1iii) and stabilization through interaction with actin and ICAM-1 (Figure 1iv). Fluorescent resonance energy transfer (FRET) studies have demonstrated cytosolic tails closely interact under resting conditions, and upon activation, the tails separate and induce an integrin conformational change to high affinity (11, 12). This process through which intracellular signals induce integrin activation is termed “inside-out” signaling. Similarly, integrin adhesion to ligand induces intracellular responses in a process termed “outside-in” signaling. High affinity integrin conformation can be achieved via either inside-out or outside-in signaling.
LFA-1 and T Cell Migration
Extravasation of immune cells from the vasculature is a highly organized process composed of five steps: (1) weak tethering/rolling, (2) firm adhesion, (3) crawling, (4) paracellular or transcellular migration through the endothelium, and (5) migration through the basement membrane (13, 14). Initial adhesion of cells to the endothelium is mediated by the expression of both selectins and addressins (15–19). Upon tethering, cells begin to decrease velocity and roll along the endothelium forming transient low-affinity interactions. Subsequently, immune cells may firmly adhere to the endothelium or be released back into circulation. Firm adhesion to the endothelium is induced by chemokine stimulations and high-affinity integrin activation via inside out signaling (20–23). The integrin conformation change can lead to as much as a 10,000-fold affinity increase of LFA-1 to its ligand ICAM-1 (3, 21). ICAM-1 is expressed at low levels and is highly upregulated upon damage or inflammation (24, 25). After secretion, chemokines bind glycosaminoglycan proteins, such as CD44 and syndecans, expressed on the endothelium (26, 27) to subsequently be presented to immune cells. Chemokine and LFA-1 engagement initiate a series of intracellular cascades that induce T cell polarization inducing subsequent migration (28, 29).
LFA-1-mediated adhesion plays essential roles in both naïve and activated T cells extravasation into the lymph node and tissue, respectively (30). Shulman et al. demonstrated that this process can be mediated via intra-endothelial chemokine stores at the immune/endothelial cell synapse and surprisingly, that T cell adhesion to the endothelium appeared independent of chemokine stimulation (31, 32). With single-dye tracking and conformation specific antibodies, Bakker et al. demonstrate that in resting monocytes that roughly 5% of LFA-1 is in nanoclusters that are in a fully active state and bound to the cytoskeleton, suggesting that low levels of LFA-1 activation may occur independently of chemokine stimuli (33). It is tempting to speculate from these data that integrin engagement may occur independent of chemokine stimulation suggesting that chemokine may simply act to reinforce integrin engagement and facilitate transmigration. While high affinity interactions are necessary for adhesion, constitutive expression of LFA-1 with the intermediate affinity I-domain led to impaired crawling and diapadesis through limiting detachment at the rear of the cell (34). This demonstrates a need for dynamic regulation of LFA-1 affinity. Indeed, studies have demonstrated that defects in LFA-1 adhesion and activation (changes in conformation, clustering, or cell signaling) through therapeutic treatments and genetic abnormalities can cause deficient immune response and autoimmunity (2, 35–37).
While LFA-1 plays a crucial role in adhesion to the vasculature, very late antigen-4 (VLA-4; α4β1) has also been implicated in T cell extravasation (14, 38–41). In tissues such as the CNS, LFA-1 inhibition is not sufficient to inhibit cell extravasation as cells also utilize VLA-4 (42, 43). However, in other tissues such as the retina, T cell infiltration was LFA-1 dependent and vastly VLA-4 independent suggesting that tissue-specificity plays a critical role in determining integrin-mediated extravasation (44). Indeed, in a bronchial epithelial model, inhibition of LFA-1 lead to a 75% decrease in infiltration whereas inhibition of ICAM-1 or ICAM-2 alone lead to a 50% reduction. However, when both ICAM-1 and ICAM-2 were blocked a 70% reduction in infiltration was observed (45). Additionally, immune cells may alter their integrin dependency. Glatigny et al. demonstrated in T regulatory (Treg) cells that when VLA-4 expression was blocked, cells were still capable of migration utilizing LFA-1 (46). These data demonstrates a highly diverse set of mechanisms, which dictate immune cell infiltration (43).
After firm adhesion, T cells crawl along the endothelium searching for a site to migrate across the endothelial monolayer into the tissue (47). Migration along the endothelium is primarily dictated by chemokine signals that direct cell chemotaxis via chemotactic gradients. However, LFA-1-ICAM-1 interaction also plays a critical role in regulating the direction of T cell migration in the blood vessel. T cells and hematopoietic stem/progenitor cells can migrate against shear flow on ICAM-1, while T cells mainly migrate with the flow on VCAM-1 (42, 48, 49). Diapadesis can occur through either paracellular (in between the junction of two cells) or transcellular (through a single endothelial cell) mechanisms. While most cells (~90%) are thought to utilize paracellular migration, the processes dictating para- vs. trans- cellular migration are still being investigated (50, 51). Evidence suggests that ICAM-1 density, monolayer organization (e.g., tricellular junctions), and previous cell diapadesis at the same location (“hot spots”) are all implicated in dictating this phenomenon (50, 52–55). Additionally, cells have been found to survey the tissue with LFA-1/Wiskott–Aldrich Syndrome Protein-dependent protrusions, which have been observed to penetrate as deep as 600 nm into the endothelial cell to promote transcellular migration (56).
Additionally, endothelial cells may facilitate diapadesis via the lateral border recycling compartment (LBRC) (54, 57). Mediating changes in endothelial cell junctions to facilitate extravasation, the LBRC has been shown to be essential for transcellular migration. Additionally, numerous diapadesis regulators, including cadherins, CD99, junction adhesion molecules, and platelet endothelial cell adhesion molecules are thought to determine and mediate cell extravasation and are thus a topic of continued research (58–60). We demonstrated that uropod elongation acts as the final step in leukocyte transendothelial migration. During this elongation, CD18+ microparticles are left behind which may play a role in either prevention or promotion of leukocyte transmigration at the site (55).
Upon successful migration across the monolayer, T cells utilize a number of β4 and β1 integrins to migrate along the basement membrane composed primarily of collagen and laminin. Intriguingly, the basement membrane appears to be lost directly at the transmigration site (61, 62). While the exact reason for this loss remains under investigation, it is believed to help control cell migration, mediate cell death at the infiltration site, and maintain tissue structure. Following this last step of migration across the endothelial barrier, immune cells continue to migrate through the tissue interstitium to exert their effector function (63).
During T cell migration, LFA-1 engagement is primarily utilized in two-dimensional spaces. One study found that neither LFA-1 nor α4 integrins support stable adhesions of naive T cells to neighboring T cells, DCs or stroma in the lymph node T cell zones (64). Indeed, studies have shown that in dense, 3D tissues dendritic cells are capable of migrating without integrin adhesion though actin-polymerization (“flowing”) and myosin II-based contractions (“squeezing”) (65). However, T cells appear to require integrin-mediated adhesion in the tissue microenvironments under inflammation (66). Therefore, it is likely that integrin-mediated T cell migration is determined by integrin/ligand expression and tissue density in which the cell is found. It is also important to note that, while LFA-1-independent migration occurs under depleting conditions within the lymph node, the outcome of the immune response may be altered. Additional studies demonstrated that LFA-1 blockade abolished directed, high velocity migration of naïve T cells (67), suggesting that LFA-1-mediated migration is important for the speed, and the pattern of T cell migration in the lymph node.
In addition to the conformational changes in LFA-1 (see Chapter 1), precise and dynamic regulation of LFA-1 recycling is a key to ensure efficient T cell migration and adapt to the constantly changing microenvironment (68, 69). While LFA-1 recycling occurs constitutively, as much as 75% of all integrins are internalized and redistributed within 15 min of cell migration onset (69). While integrins can utilize both clathrin-dependent and -independent pathways (69), LFA-1-dependent endocytosis is primarily mediated through clathrin-independent, cholesterol-sensitive mechanisms (68) and play an important role both in mediating cell migration and cell polarization through the partitioning of molecules near LFA-1 (68–70). Upon internalization, a series of steps determine the fate of intracellular LFA-1 (degradation vs. recycling). Integrins are generally thought to return to the cell surface through via either a direct exocytosis route (via Rab4 or Rab5) or the perinuclear recycling compartment route (via Rab11) (69, 71, 72). LFA-1 containing vesicles during T-antigen-presenting cell (APC) interactions have also been found to require Arf6 + Rab22 (72). LFA-1 can specifically utilize a Rab13-dependent pathway through which Rab13 associates with Mst1 to facilitate increased integrin activation, as evidenced by increased LFA-1 clustering and cell migration (69, 73). Additionally, LFA-1 recycling requires Rap2-expressing vesicles which work synergistically with Rab13 to mediate new adhesion, while Rap2 facilitates continuous adhesion (74). T cell activation may also be impeded through defects in LFA-1 recycling as demonstrated with Rab13 or Rab27 inhibition (73, 75, 76). However, determining the precise roles of each recycling mechanism during LFA-1-mediated cell migration and activation requires more investigation.
LFA-1 and T Cell Activation
T cell activation is a highly organized process that can be divided into distinct events described by both T cell motility in the lymph node and T-APC interactions. The first phase is highly dynamic, as immune cells migrate along fibroblastic reticular cells (FRCs) while scanning for antigens. The second phase is defined by low motility and high interaction between the T cells and APCs. The final stage is characterized by regaining a high level of motility, effector differentiation, and proliferation (77).
T cells migrate along FRCs to sample antigens via random interactions with antigen-bearing dendritic cells. These short, transient interactions, termed kinapses, are characterized by reduced T cell migration (78). Interaction with APC is determined via affinity between the peptide-MHC (pMHC) complex and the T cell receptor (TCR). As these interactions are low affinity (1–100 nM Kd), they are highly specific for only 1 × 105–1 × 106 TCRs (79, 80). Upon recognition of cognate antigen, a T cell ceases migration and induces surface and cytosolic changes in both the APC and the T cell. This phenomenon is defined as the second phase of T cell activation and is characterized by a loss of motility, extended T cell/APC interaction and the formation of an immunological synapse (IS) (77, 78). These changes include a loss of polarity in the T cell and surface molecule reorganization referred to as supramolecular activation clusters (SMACs). The IS can be segregated into three distinct portions similar to a bullseye pattern. The center of the bullseye, aptly named center-SMAC, contains the TCR/CD3 complex and the co-stimulatory molecules CD28 and PKCθ. The outermost ring, or the distal ring (d-SMAC), is composed of the phosphatase CD45, and the center ring, or peripheral SMAC (p-SMAC) is composed of LFA-1 and talin (81). Surprisingly, it has recently been observed that under basal conditions, LFA-1 can be found in clusters prebound to the cytoskeleton suggesting this may help to induce initial cell adhesion and formation of nanoclusters upon TCR engagement (33, 82). Interactions at the IS can be defined by the duration of the interaction. Synapse formation (>5 min) and kinapse formation (<5 min) are determined by the affinity of the TCR/pMHC complex and the activation phase of the T cell. Evidence suggests that both types of interaction are required for complete T cell activation to balance activation signals imparted from the APC with differentiation and proliferation. Additionally, dysregulation of this process may lead to tolerance or autoimmunity through altering the balance of activation signals the T cell receives or through altering the affinity threshold required to engage TCR/MHC complexes (78).
Interaction between TCR and pMHC induces a phosphorylation-mediated signaling cascade. This process activates LFA-1 at the IS leading to firm adhesion required for effective T cell activation. LFA-1 may be activated through a number of pathways that convert the small GTPase RAP1 to its active GTP bound form (83–85). Importantly, RAP1 activation is dependent on the context of activation (TCR vs. chemokine) with data demonstrating that RhoH functions as a rheostat with differential localization within the cell leading to alterations in LFA-1 activation (86). Many of these pathways require the LAT signalosome, including the PLCγ1 activation of DAG regulated-guanine exchange factor (GEF)1 (CalDAG-GEF), which directly acts on RAP1. Additionally the adaptor protein CRKII can interact with C3G, a GEF, to activate RAP1. CRKII-C3G can also be activated via the WASP family member 2 (WAVE2) actin related protein 2/3 complex (ARP2/3)-ABL complex. Upon conversion of RAP1 from the inactive GDP-bound form to the active GTP-bound form, it interacts with ADAP and the adaptor SKAP55 to recruit RAP1 to the plasma membrane (87). This allows for recruitment of the RAP1 effectors RAPL, Mst1, PDK, and RIAM to induce integrin activation. The RAP1/Mst1/Kindlin3 complex can be formed through inside-out integrin activation signals, but may also play a role in stabilizing outside in signaling (88–91). This process is essential for LFA-1 recycling, as RAP1 complexes play key roles in delivering LFA-1 vesicles to the cell surface (78, 81, 92, 93).
Antigen-presenting cell expression of ICAM-1 is also required for effective T cell activation. ICAM-1 expression on DC’s plays a crucial role in mediating T cell migration and localization throughout the lymph node (94–96). Additionally, ICAM-1 clustering on APC is essential for effective LFA-1 engagement and T cell activation (97, 98). Interestingly, LFA-1 has also been directly implicated by CD8+ DCs to facilitate T cell activation via acting as a scavenger receptor to collect antigen from antigen-bearing DCs (99).
Disregulation of LFA-1 expression can lead to changes in T cell activation and differentiation (28, 100–102). Stable engagement of LFA-1/ICAM-1 is required to re-enforce many pathways for complete T cell differentiation. LFA-1 crosstalk with Notch signaling has been shown to induce IFNγ production and re-enforce Th1 cell functions suggesting that LFA-1 engagement with tissue resident APCs will further strengthen T cell differentiation (103). Similarly, Tregs have been shown to require talin and LFA-1 activation to induce IL-2Rα upregulation, which is required for Treg function (104, 105). Additionally, we recently demonstrated that an intracellular pool of LFA-1 is relocalized to the cell surface upon initial T/APC interactions and plays a key role in T cell memory development (76).
LFA-1 and T Cell Cytotoxic Response
Fully differentiated effector CD8+ T cells kill infected/transformed target cells via caspase-dependent apoptosis (106, 107). Upon recognition of a target antigen via TCR/pMHC complex formation, CD8+ T cell activates LFA-1 and binds to ICAM-1 expressed on the target cell. Important functions of LFA-1 during the cytotoxic response was demonstrated with LFA-1 blockers that inhibited target cell killing (107–109). Cytotoxic T cells form short, LFA-1 driven, kinapse-like interactions with infected cells to facilitate killing with interactions for as little as 10 min inducing apoptosis in target cells (110–112). Similar to the IS formed during T cell activation, TCR-derived (113–115), inside-out signals induce translocation of the microtubule organizing center (MTOC) toward the contact between IS and the target cell. The MTOC and microtubules interact with LFA-1 within the p-SMAC to define the ring shape structure observed during perforin/granzyme release (116–118). Organization of LFA-1 at the p-SMAC is thought to act as a “gasket” to prevent cytolytic granules from escaping (119). Furthermore, studies have indicated that the stability and strength of the LFA-1-mediated contact is critical for effective cytolytic activity (118). Additionally, CTLA-4 signaling has been shown to lead to RAP1-mediated increase in LFA-1 binding (120, 121). While the purpose is unclear, it is possible that this mediates low affinity TCR interactions or in cases of high stimulation, induces greater cell polarization and migration. Finally, galectin coating of the tumor infiltrating leukocytes (TILs) surfaces has led to decreased LFA-1 recruitment and activation at the IS and reduced cytokine secretion, further supporting a key role for LFA-1 in mediating TIL cytotoxic function (109).
LFA-1 and T Cell Memory Development
As described above, LFA-1 plays a critical role in facilitating naïve T cell activation and differentiation through T cell-APC contacts. Indeed, defects in LFA-1/ICAM-1 interactions have been shown to lead to impairment of memory formation (122, 123). Interestingly, ICAM-1 expression on T cells is important for T cell clustering during transient T–T interactions that provide additional cues for proliferation (124). ICAM-1 deficient cells resulted in higher levels of IFN-γ and granzyme B as well as, increased KLRG-1 expression suggesting increased differentiation toward short-lived effector cells (97). LFA-1 expression is required for the retention of tissue resident memory cells in the hepatic sinusoids and facilitate their migratory patterns unlike skin resident memory cells that are largely sessile (125). Additionally, numerous allograft rejection model studies have demonstrated both in mice and non-human primates that LFA-1 blockades reduce or delay memory cell mediated rejection (126–129). While the precise mechanism of this appears to be a combination of infiltration, proliferation, and cytokine secretion, this demonstrates LFA-1’s multifaceted role in memory T cell function. Finally, it is important to note that this is not exclusive to LFA-1 as studies have suggested the integrin VLA-1 is required for memory T cell development in the airway against influenza infection (130, 131).
Intriguingly, several studies have shown that LFA-1, along with CD8, CD3, and CD43, are asymmetrically inherited into the two daughter (proximal vs. distal) cells upon initial T cell division (132, 133). This has been further studied with fate-associated factors such as IL-2Rα, IFNγR, and T-bet all being asymmetrically distributed (133). Importantly, we demonstrated that unequal inheritance of LFA-1 in daughter cells caused differences in migration, T-APC contacts, tissue retention, and effector functions (76). This study further demonstrated that the unequal inheritance of LFA-1 plays an important role in memory generation and differentiation of T cells into both effector and memory subsets.
LFA-1 as a Mechanosensor
Recent evidence suggests a role for LFA-1 as a mechanosensor affecting cell signaling and integrin activation through the force generated by ligand binding. For example, it has been proposed that integrin adhesion occurs through a “catch bond” in which as the tension at the ligand binding site increases, the affinity also increases (134, 135). Recent work with a FRET-based LFA-1 tension sensor demonstrated significant tension across the β subunit of LFA-1 upon ICAM-1 binding resulting in the stabilization of active LFA-1 (136). Importantly, force generation requires adhesion to both ICAM-1 and actin to result in increased integrin constraint, cell tension, and cell signaling (33, 98, 136, 137). Actin remodeling via WASP-dependent mechanisms is essential for the assembly and distribution of high affinity LFA-1 clusters at the IS. The control of LFA-1 topology at the IS by WASP is related to both the control of the CD4+ T cell stop signal (138) and the CD8+ T cell cytotoxic activity (139). Further work has demonstrated that retrograde actin flow dictates LFA-1 orientation when bound to ICAM-1 on migrating Jurkat T (137). Similarly, TAGLN2 dependent inhibition of actin depolymerization is required for stable IS (140). Unsurprisingly, this force generation has been shown to be an important part of IS formation, cell cytotoxicity, and may modulate cell migration (42, 136, 141–143). As described in reviews by Sun et al. and Gauthier et al., the role of integrin tensile force requires continued study to fully elucidate its functions on in T cell activation, migration, and cytotoxicity (144, 145).
Conclusion
As this review has shown, LFA-1 functions are extremely varied but play a critical role in facilitating effective immune responses. Our understanding of the mechanisms through which LFA-1 mediates immune cell function have grown exponentially, yet many questions still remain. As our understanding grows, our capability to modulate this highly adaptive molecule to better treat autoimmunity, cancer, and allograft rejection will continue to improve.
Author Contributions
BW and MK both researched the topic, wrote and edited the manuscript, and made the figure for this manuscript.
Conflict of Interest Statement
The authors declare that the research was conducted in the absence of any commercial or financial relationships that could be construed as a potential conflict of interest.
The handling Editor declared a shared affiliation, though no other collaboration, with the authors.
Acknowledgments
We would like to thank the Kim lab for helpful discussions.
Funding
This work was financially supported through grants from the National Institute of Health (AI02851 to MK). The authors have no conflicting financial interests.
References
1. Mitroulis I, Alexaki VI, Kourtzelis I, Ziogas A, Hajishengallis G, Chavakis T. Leukocyte integrins: role in leukocyte recruitment and as therapeutic targets in inflammatory disease. Pharmacol Ther (2015) 147:123–35. doi:10.1016/j.pharmthera.2014.11.008
2. Ley K, Rivera-Nieves J, Sandborn WJ, Shattil S. Integrin-based therapeutics: biological basis, clinical use and new drugs. Nat Rev Drug Discov (2016) 15:173–83. doi:10.1038/nrd.2015.10
3. Shimaoka M, Takagi J, Springer TA. Conformational regulation of integrin structure and function. Annu Rev Biophys Biomol Struct (2002) 31:485–516. doi:10.1146/annurev.biophys.31.101101.140922
4. Shimaoka M, Lu C, Palframan RT, Von Andrian UH, Mccormack A, Takagi J, et al. Reversibly locking a protein fold in an active conformation with a disulfide bond: integrin alphaL I domains with high affinity and antagonist activity in vivo. Proc Natl Acad Sci U S A (2001) 98:6009–14. doi:10.1073/pnas.101130498
5. Shimaoka M, Xiao T, Liu JH, Yang Y, Dong Y, Jun CD, et al. Structures of the alpha L I domain and its complex with ICAM-1 reveal a shape-shifting pathway for integrin regulation. Cell (2003) 112:99–111. doi:10.1016/S0092-8674(02)01257-6
6. Lu C, Shimaoka M, Ferzly M, Oxvig C, Takagi J, Springer TA. An isolated, surface-expressed I domain of the integrin alphaLbeta2 is sufficient for strong adhesive function when locked in the open conformation with a disulfide bond. Proc Natl Acad Sci U S A (2001) 98:2387–92. doi:10.1073/pnas.041606398
7. Luo BH, Springer TA. Integrin structures and conformational signaling. Curr Opin Cell Biol (2006) 18:579–86. doi:10.1016/j.ceb.2006.08.005
8. Luo B-H, Carman CV, Springer TA. Structural basis of integrin regulation and signaling. Annu Rev Immunol (2007) 25:619–47. doi:10.1146/annurev.immunol.25.022106.141618
9. Xie C, Zhu J, Chen X, Mi L, Nishida N, Springer TA. Structure of an integrin with an alphaI domain, complement receptor type 4. EMBO J (2010) 29:666–79. doi:10.1038/emboj.2009.367
10. Shimonaka M, Katagiri K, Nakayama T, Fujita N, Tsuruo T, Yoshie O, et al. Rap1 translates chemokine signals to integrin activation, cell polarization, and motility across vascular endothelium under flow. J Cell Biol (2003) 161:417–27. doi:10.1083/jcb.200301133
11. Dustin ML, Springer TA. T-cell receptor cross-linking transiently stimulates adhesiveness through LFA-1. Nature (1989) 341:619–24. doi:10.1038/341619a0
12. Kim M, Carman CV, Springer TA. Bidirectional transmembrane signaling by cytoplasmic domain separation in integrins. Science (2003) 301:1720–5. doi:10.1126/science.1084174
13. Spangrude GJ, Braaten BA, Daynes RA. Molecular mechanisms of lymphocyte extravasation. I. Studies of two selective inhibitors of lymphocyte recirculation. J Immunol (1984) 132:354–62.
14. Sumagin R, Prizant H, Lomakina E, Waugh RE, Sarelius IH. LFA-1 and Mac-1 define characteristically different intralumenal crawling and emigration patterns for monocytes and neutrophils in situ. J Immunol (2010) 185:7057–66. doi:10.4049/jimmunol.1001638
15. Chen S, Springer TA. An automatic braking system that stabilizes leukocyte rolling by an increase in selectin bond number with shear. J Cell Biol (1999) 144:185–200. doi:10.1083/jcb.144.1.185
16. Weninger W, Crowley MA, Manjunath N, Andrian UHV. Migratory properties of naive, effector, and memory Cd8+ T cells. J Exp Med (2001) 194:953–66. doi:10.1084/jem.194.7.953
17. M’Rini C, Cheng G, Schweitzer C, Cavanagh LL, Palframan RT, Mempel TR, et al. A novel endothelial L-selectin ligand activity in lymph node medulla that is regulated by alpha(1,3)-fucosyltransferase-IV. J Exp Med (2003) 198:1301–12. doi:10.1084/jem.20030182
18. Baaten BJ, Cooper AM, Swain SLPD, Bradley LM. Location, location, location: the impact of migratory heterogeneity on T cell function. Immunol Memory (2013) 4:311. doi:10.3389/fimmu.2013.00311
19. McEver RP. Selectins: initiators of leucocyte adhesion and signalling at the vascular wall. Cardiovasc Res (2015) 107:331–9. doi:10.1093/cvr/cvv154
20. Bleul CC, Fuhlbrigge RC, Casasnovas JM, Aiuti A, Springer TA. A highly efficacious lymphocyte chemoattractant, stromal cell-derived factor 1 (SDF-1). J Exp Med (1996) 184:1101–9. doi:10.1084/jem.184.3.1101
21. Constantin G, Majeed M, Giagulli C, Piccio L, Kim JY, Butcher EC, et al. Chemokines trigger immediate β2 integrin affinity and mobility changes: differential regulation and roles in lymphocyte arrest under flow. Immunity (2000) 13:759–69. doi:10.1016/S1074-7613(00)00074-1
22. Murdoch C, Finn A. Chemokine receptors and their role in inflammation and infectious diseases. Blood (2000) 95(10):3032–43.
23. Comerford I, Harata-Lee Y, Bunting MD, Gregor C, Kara EE, Mccoll SR. A myriad of functions and complex regulation of the CCR7/CCL19/CCL21 chemokine axis in the adaptive immune system. Cytokine Growth Factor Rev (2013) 24:269–83. doi:10.1016/j.cytogfr.2013.03.001
24. Pober JS. Effects of tumour necrosis factor and related cytokines on vascular endothelial cells. Ciba Found Symp (1987) 131:170–84.
25. Patarroyo M, Makgoba MW. Leucocyte adhesion to cells in immune and inflammatory responses. Lancet (1989) 2:1139–42. doi:10.1016/S0140-6736(89)91498-0
26. Haynes BF, Telen MJ, Hale LP, Denning SM. CD44 – a molecule involved in leukocyte adherence and T-cell activation. Immunol Today (1989) 10:423–8. doi:10.1016/0167-5699(89)90040-6
27. Middleton J, Patterson AM, Gardner L, Schmutz C, Ashton BA. Leukocyte extravasation: chemokine transport and presentation by the endothelium. Blood (2002) 100:3853–60. doi:10.1182/blood.V100.12.3853
28. Raab M, Wang H, Lu Y, Smith X, Wu Z, Strebhardt K, et al. T cell receptor “inside-out” pathway via signaling module SKAP1-RapL regulates T cell motility and interactions in lymph nodes. Immunity (2010) 32:541–56. doi:10.1016/j.immuni.2010.03.007
29. Springer TA, Dustin ML. Integrin inside-out signaling and the immunological synapse. Curr Opin Cell Biol (2012) 24:107–15. doi:10.1016/j.ceb.2011.10.004
30. Krummel MF, Bartumeus F, Gerard A. T cell migration, search strategies and mechanisms. Nat Rev Immunol (2016) 16:193–201. doi:10.1038/nri.2015.16
31. Shulman Z, Shinder V, Klein E, Grabovsky V, Yeger O, Geron E, et al. Lymphocyte crawling and transendothelial migration require chemokine triggering of high-affinity LFA-1 integrin. Immunity (2009) 30:384–96. doi:10.1016/j.immuni.2008.12.020
32. Shulman Z, Cohen SJ, Roediger B, Kalchenko V, Jain R, Grabovsky V, et al. Transendothelial migration of lymphocytes mediated by intraendothelial vesicle stores rather than by extracellular chemokine depots. Nat Immunol (2012) 13:67–76. doi:10.1038/ni.2173
33. Bakker GJ, Eich C, Torreno-Pina JA, Diez-Ahedo R, Perez-Samper G, Van Zanten TS, et al. Lateral mobility of individual integrin nanoclusters orchestrates the onset for leukocyte adhesion. Proc Natl Acad Sci U S A (2012) 109:4869–74. doi:10.1073/pnas.1116425109
34. Park EJ, Peixoto A, Imai Y, Goodarzi A, Cheng G, Carman CV, et al. Distinct roles for LFA-1 affinity regulation during T-cell adhesion, diapedesis, and interstitial migration in lymph nodes. Blood (2010) 115:1572–81. doi:10.1182/blood-2009-08-237917
35. Shattil SJ, Kim C, Ginsberg MH. The final steps of integrin activation: the end game. Nat Rev Mol Cell Biol (2010) 11:288–300. doi:10.1038/nrm2871
36. Lowin T, Straub RH. Integrins and their ligands in rheumatoid arthritis. Arthritis Res Ther (2011) 13:244. doi:10.1186/ar3464
37. Schwab N, Ulzheimer JC, Fox RJ, Schneider-Hohendorf T, Kieseier BC, Monoranu CM, et al. Fatal PML associated with efalizumab therapy. Neurology (2012) 78:458–67. doi:10.1212/WNL.0b013e3182478d4b
38. Springer TA, Rothlein R, Anderson DC, Burakoff SJ, Krensky AM. The function of LFA-1 in cell-mediated killing and adhesion: studies on heritable LFA-1, Mac-1 deficiency and on lymphoid cell self-aggregation. Adv Exp Med Biol (1985) 184:311–22. doi:10.1007/978-1-4684-8326-0_21
39. Elices MJ, Osborn L, Takada Y, Crouse C, Luhowskyj S, Hemler ME, et al. VCAM-1 on activated endothelium interacts with the leukocyte integrin VLA-4 at a site distinct from the VLA-4/fibronectin binding site. Cell (1990) 60:577–84. doi:10.1016/0092-8674(90)90661-W
40. Shimizu K, Libby P, Shubiki R, Sakuma M, Wang Y, Asano K, et al. Leukocyte integrin Mac-1 promotes acute cardiac allograft rejection. Circulation (2008) 117:1997–2008. doi:10.1161/CIRCULATIONAHA.107.724310
41. Zarbock A, Ley K, Mcever RP, Hidalgo A. Leukocyte ligands for endothelial selectins: specialized glycoconjugates that mediate rolling and signaling under flow. Blood (2011) 118:6743–51. doi:10.1182/blood-2011-07-343566
42. Dominguez GA, Anderson NR, Hammer DA. The direction of migration of T-lymphocytes under flow depends upon which adhesion receptors are engaged. Integr Biol (Camb) (2015) 7:345–55. doi:10.1039/c4ib00201f
43. Schlager C, Korner H, Krueger M, Vidoli S, Haberl M, Mielke D, et al. Effector T-cell trafficking between the leptomeninges and the cerebrospinal fluid. Nature (2016) 530:349–53. doi:10.1038/nature16939
44. Dewispelaere R, Lipski D, Foucart V, Bruyns C, Frere A, Caspers L, et al. ICAM-1 and VCAM-1 are differentially expressed on blood-retinal barrier cells during experimental autoimmune uveitis. Exp Eye Res (2015) 137:94–102. doi:10.1016/j.exer.2015.06.017
45. Porter JC, Hall A. Epithelial ICAM-1 and ICAM-2 regulate the egression of human T cells across the bronchial epithelium. FASEB J (2009) 23:492–502. doi:10.1096/fj.08-115899
46. Glatigny S, Duhen R, Arbelaez C, Kumari S, Bettelli E. Integrin alpha L controls the homing of regulatory T cells during CNS autoimmunity in the absence of integrin alpha 4. Sci Rep (2015) 5:7834. doi:10.1038/srep07834
47. Thelen M, Stein JV. How chemokines invite leukocytes to dance. Nat Immunol (2008) 9:953–9. doi:10.1038/ni.f.207
48. Steiner O, Coisne C, Cecchelli R, Boscacci R, Deutsch U, Engelhardt B, et al. Differential roles for endothelial ICAM-1, ICAM-2, and VCAM-1 in shear-resistant T cell arrest, polarization, and directed crawling on blood-brain barrier endothelium. J Immunol (2010) 185:4846–55. doi:10.4049/jimmunol.0903732
49. Buffone A Jr, Anderson NR, Hammer DA. Migration against the direction of flow is LFA-1 dependent in human hematopoietic stem and progenitor cells. J Cell Sci (2017) 131:jcs205575. doi:10.1242/jcs.205575
50. Sumagin R, Sarelius IH. Intercellular adhesion molecule-1 enrichment near tricellular endothelial junctions is preferentially associated with leukocyte transmigration and signals for reorganization of these junctions to accommodate leukocyte passage. J Immunol (2010) 184:5242–52. doi:10.4049/jimmunol.0903319
51. Woodfin A, Voisin MB, Beyrau M, Colom B, Caille D, Diapouli FM, et al. The junctional adhesion molecule JAM-C regulates polarized transendothelial migration of neutrophils in vivo. Nat Immunol (2011) 12:761–9. doi:10.1038/ni.2062
52. Allingham MJ, Buul JDV, Burridge K. ICAM-1-mediated, Src- and Pyk2-dependent vascular endothelial cadherin tyrosine phosphorylation is required for leukocyte transendothelial migration. J Immunol (2007) 179:4053–64. doi:10.4049/jimmunol.179.6.4053
53. Wallez Y, Huber P. Endothelial adherens and tight junctions in vascular homeostasis, inflammation and angiogenesis. Biochim Biophys Acta (2008) 1778:794–809. doi:10.1016/j.bbamem.2007.09.003
54. Mamdouh Z, Mikhailov A, Muller WA. Transcellular migration of leukocytes is mediated by the endothelial lateral border recycling compartment. J Exp Med (2009) 206:2795–808. doi:10.1084/jem.20082745
55. Hyun YM, Sumagin R, Sarangi PP, Lomakina E, Overstreet MG, Baker CM, et al. Uropod elongation is a common final step in leukocyte extravasation through inflamed vessels. J Exp Med (2012) 209:1349–62. doi:10.1084/jem.20111426
56. Carman CV, Sage PT, Sciuto TE, De La Fuente MA, Geha RS, Ochs HD, et al. Transcellular diapedesis is initiated by invasive podosomes. Immunity (2007) 26:784–97. doi:10.1016/j.immuni.2007.04.015
57. Sullivan DP, Ruffer C, Muller WA. Isolation of the lateral border recycling compartment using a diaminobenzidine-induced density shift. Traffic (2014) 15:1016–29. doi:10.1111/tra.12184
58. Broermann A, Winderlich M, Block H, Frye M, Rossaint J, Zarbock A, et al. Dissociation of VE-PTP from VE-cadherin is required for leukocyte extravasation and for VEGF-induced vascular permeability in vivo. J Exp Med (2011) 208:2393–401. doi:10.1084/jem.20110525
59. Vestweber D. Relevance of endothelial junctions in leukocyte extravasation and vascular permeability. Ann N Y Acad Sci (2012) 1257:184–92. doi:10.1111/j.1749-6632.2012.06558.x
60. Vockel M, Vestweber D. How T cells trigger the dissociation of the endothelial receptor phosphatase VE-PTP from VE-cadherin. Blood (2013) 122:2512–22. doi:10.1182/blood-2013-04-499228
61. Ratzinger G, Stoitzner P, Ebner S, Lutz MB, Layton GT, Rainer C, et al. Matrix metalloproteinases 9 and 2 are necessary for the migration of Langerhans cells and dermal dendritic cells from human and murine skin. J Immunol (2002) 168:4361–71. doi:10.4049/jimmunol.168.9.4361
62. Kelley LC, Lohmer LL, Hagedorn EJ, Sherwood DR. Traversing the basement membrane in vivo: a diversity of strategies. J Cell Biol (2014) 204:291–302. doi:10.1083/jcb.201311112
63. Huber AR, Weiss SJ. Disruption of the subendothelial basement membrane during neutrophil diapedesis in an in vitro construct of a blood vessel wall. J Clin Invest (1989) 83:1122–36. doi:10.1172/JCI113992
64. Woolf E, Grigorova I, Sagiv A, Grabovsky V, Feigelson SW, Shulman Z, et al. Lymph node chemokines promote sustained T lymphocyte motility without triggering stable integrin adhesiveness in the absence of shear forces. Nat Immunol (2007) 8:1076–85. doi:10.1038/ni1499
65. Lammermann T, Bader BL, Monkley SJ, Worbs T, Wedlich-Soldner R, Hirsch K, et al. Rapid leukocyte migration by integrin-independent flowing and squeezing. Nature (2008) 453:51–5. doi:10.1038/nature06887
66. Overstreet MG, Gaylo A, Angermann BR, Hughson A, Hyun Y-M, Lambert K, et al. Inflammation-induced interstitial migration of effector CD4+ T cells is dependent on integrin [alpha]V. Nat Immunol (2013) 14:949–58. doi:10.1038/ni.2682
67. Katakai T, Habiro K, Kinashi T. Dendritic cells regulate high-speed interstitial T cell migration in the lymph node via LFA-1/ICAM-1. J Immunol (2013) 191:1188–99. doi:10.4049/jimmunol.1300739
68. Fabbri M, Di Meglio S, Gagliani MC, Consonni E, Molteni R, Bender JR, et al. Dynamic partitioning into lipid rafts controls the endo-exocytic cycle of the alphaL/beta2 integrin, LFA-1, during leukocyte chemotaxis. Mol Biol Cell (2005) 16:5793–803. doi:10.1091/mbc.E05-05-0413
69. Caswell PT, Vadrevu S, Norman JC. Integrins: masters and slaves of endocytic transport. Nat Rev Mol Cell Biol (2009) 10:843–53. doi:10.1038/nrm2799
70. Fabbri M, Fumagalli L, Bossi G, Bianchi E, Bender JR, Pardi R. A tyrosine-based sorting signal in the beta2 integrin cytoplasmic domain mediates its recycling to the plasma membrane and is required for ligand-supported migration. EMBO J (1999) 18:4915–25. doi:10.1093/emboj/18.18.4915
71. Pellinen T, Arjonen A, Vuoriluoto K, Kallio K, Fransen JA, Ivaska J. Small GTPase Rab21 regulates cell adhesion and controls endosomal traffic of beta1-integrins. J Cell Biol (2006) 173:767–80. doi:10.1083/jcb.200509019
72. Johnson DL, Wayt J, Wilson JM, Donaldson JG. Arf6 and Rab22 mediate T cell conjugate formation by regulating clathrin-independent endosomal membrane trafficking. J Cell Sci (2017) 130:2405–15. doi:10.1242/jcs.200477
73. Nishikimi A, Ishihara S, Ozawa M, Etoh K, Fukuda M, Kinashi T, et al. Rab13 acts downstream of the kinase Mst1 to deliver the integrin LFA-1 to the cell surface for lymphocyte trafficking. Sci Signal (2014) 7:ra72. doi:10.1126/scisignal.2005199
74. Stanley P, Tooze S, Hogg N. A role for Rap2 in recycling the extended conformation of LFA-1 during T cell migration. Biol Open (2012) 1:1161–8. doi:10.1242/bio.20122824
75. Ioannou MS, Bell ES, Girard M, Chaineau M, Hamlin JN, Daubaras M, et al. DENND2B activates Rab13 at the leading edge of migrating cells and promotes metastatic behavior. J Cell Biol (2015) 208:629–48. doi:10.1083/jcb.201407068
76. Capece T, Walling BL, Lim K, Kim KD, Bae S, Chung HL, et al. A novel intracellular pool of LFA-1 is critical for asymmetric CD8+ T cell activation and differentiation. J Cell Biol (2017) 216:3817–29. doi:10.1083/jcb.201609072
77. Mempel TR, Henrickson SE, Von Andrian UH. T-cell priming by dendritic cells in lymph nodes occurs in three distinct phases. Nature (2004) 427:154–9. doi:10.1038/nature02238
78. Dustin ML. T-cell activation through immunological synapses and kinapses. Immunol Rev (2008) 221:77–89. doi:10.1111/j.1600-065X.2008.00589.x
79. Rudolph MG, Stanfield RL, Wilson IA. How TCRs bind MHCs, peptides, and coreceptors. Annu Rev Immunol (2006) 24:419–66. doi:10.1146/annurev.immunol.23.021704.115658
80. Stone JD, Chervin AS, Kranz DM. T-cell receptor binding affinities and kinetics: impact on T-cell activity and specificity. Immunology (2009) 126:165–76. doi:10.1111/j.1365-2567.2008.03015.x
81. Alarcon B, Mestre D, Martinez-Martin N. The immunological synapse: a cause or consequence of T-cell receptor triggering? Immunology (2011) 133:420–5. doi:10.1111/j.1365-2567.2011.03458.x
82. Cambi A, Joosten B, Koopman M, De Lange F, Beeren I, Torensma R, et al. Organization of the integrin LFA-1 in nanoclusters regulates its activity. Mol Biol Cell (2006) 17:4270–81. doi:10.1091/mbc.E05-12-1098
83. Stadtmann A, Brinkhaus L, Mueller H, Rossaint J, Bolomini-Vittori M, Bergmeier W, et al. Rap1a activation by CalDAG-GEFI and p38 MAPK is involved in E-selectin-dependent slow leukocyte rolling. Eur J Immunol (2011) 41:2074–85. doi:10.1002/eji.201041196
84. Deng Q, Huttenlocher A. Leukocyte migration from a fish eye’s view. J Cell Sci (2012) 125:3949–56. doi:10.1242/jcs.093633
85. Garcon F, Okkenhaug K. PI3Kdelta promotes CD4(+) T-cell interactions with antigen-presenting cells by increasing LFA-1 binding to ICAM-1. Immunol Cell Biol (2016) 94:486–95. doi:10.1038/icb.2016.1
86. Baker CM, Comrie WA, Hyun YM, Chung HL, Fedorchuk CA, Lim K, et al. Opposing roles for RhoH GTPase during T-cell migration and activation. Proc Natl Acad Sci U S A (2012) 109:10474–9. doi:10.1073/pnas.1114214109
87. Lettau M, Kliche S, Kabelitz D, Janssen O. The adapter proteins ADAP and Nck cooperate in T cell adhesion. Mol Immunol (2014) 60:72–9. doi:10.1016/j.molimm.2014.03.017
88. Calderwood DA, Campbell ID, Critchley DR. Talins and kindlins: partners in integrin-mediated adhesion. Nat Rev Mol Cell Biol (2013) 14:503–17. doi:10.1038/nrm3624
89. Moretti FA, Moser M, Lyck R, Abadier M, Ruppert R, Engelhardt B, et al. Kindlin-3 regulates integrin activation and adhesion reinforcement of effector T cells. Proc Natl Acad Sci U S A (2013) 110:17005–10. doi:10.1073/pnas.1316032110
90. Rognoni E, Ruppert R, Fassler R. The kindlin family: functions, signaling properties and implications for human disease. J Cell Sci (2016) 129:17–27. doi:10.1242/jcs.161190
91. Kondo N, Ueda Y, Kita T, Ozawa M, Tomiyama T, Yasuda K, et al. NDR1-dependent regulation of kindlin-3 controls high-affinity LFA-1 binding and immune synapse organization. Mol Cell Biol (2017) 37:e424–416. doi:10.1128/MCB.00424-16
92. Yokosuka T, Saito T. The immunological synapse, TCR microclusters, and T cell activation. In: Saito T, Batista F, editors. Immunological Synapse. Current Topics in Microbiology and Immunology. Vol 340. Berlin, Heidelberg: Springer (2010).
93. Brownlie RJ, Zamoyska R. T cell receptor signalling networks: branched, diversified and bounded. Nat Rev Immunol (2013) 13:257–69. doi:10.1038/nri3403
94. Boyd AW, Wawryk SO, Burns GF, Fecondo JV. Intercellular adhesion molecule 1 (ICAM-1) has a central role in cell-cell contact-mediated immune mechanisms. Proc Natl Acad Sci U S A (1988) 85:3095–9. doi:10.1073/pnas.85.9.3095
95. Dubey C, Croft M, Swain SL. Costimulatory requirements of naive CD4+ T cells. ICAM-1 or B7-1 can costimulate naive CD4 T cell activation but both are required for optimum response. J Immunol (1995) 155:45–57.
96. Petruzzelli L, Maduzia L, Springer TA. Differential requirements for LFA-1 binding to ICAM-1 and LFA-1-mediated cell aggregation. J Immunol (1998) 160:4208–16.
97. Zumwalde NA, Domae E, Mescher MF, Shimizu Y. ICAM-1-dependent homotypic aggregates regulate CD8 T cell effector function and differentiation during T cell activation. J Immunol (2013) 191:3681–93. doi:10.4049/jimmunol.1201954
98. Comrie WA, Li S, Boyle S, Burkhardt JK. The dendritic cell cytoskeleton promotes T cell adhesion and activation by constraining ICAM-1 mobility. J Cell Biol (2015) 208:457–73. doi:10.1083/jcb.201406120
99. Segura E, Guerin C, Hogg N, Amigorena S, Thery C. CD8+ dendritic cells use LFA-1 to capture MHC-peptide complexes from exosomes in vivo. J Immunol (2007) 179:1489–96. doi:10.4049/jimmunol.179.3.1489
100. Tohyama Y, Katagiri K, Pardi R, Lu C, Springer TA, Kinashi T. The critical cytoplasmic regions of the alphaL/beta2 integrin in Rap1-induced adhesion and migration. Mol Biol Cell (2003) 14:2570–82. doi:10.1091/mbc.E02-09-0615
101. Wang Y, Li D, Nurieva R, Yang J, Sen M, Carreño R, et al. LFA-1 affinity regulation is necessary for the activation and Proliferation of naive T cells. J Biol Chem (2009) 284:12645–53. doi:10.1074/jbc.M807207200
102. Wabnitz GH, Lohneis P, Kirchgessner H, Jahraus B, Gottwald S, Konstandin M, et al. Sustained LFA-1 cluster formation in the immune synapse requires the combined activities of L-plastin and calmodulin. Eur J Immunol (2010) 40:2437–49. doi:10.1002/eji.201040345
103. Verma NK, Fazil MH, Ong ST, Chalasani ML, Low JH, Kottaiswamy A, et al. LFA-1/ICAM-1 ligation in human T cells promotes Th1 polarization through a GSK3beta signaling-dependent notch pathway. J Immunol (2016) 197:108–18. doi:10.4049/jimmunol.1601160
104. Chinen T, Kannan AK, Levine AG, Fan X, Klein U, Zheng Y, et al. An essential role for the IL-2 receptor in Treg cell function. Nat Immunol (2016) 17:1322–33. doi:10.1038/ni.3540
105. Klann JE, Remedios KA, Kim SH, Metz PJ, Lopez J, Mack LA, et al. Talin plays a critical role in the maintenance of the regulatory T cell pool. J Immunol (2017) 198:4639–51. doi:10.4049/jimmunol.1601165
106. Andersen MH, Schrama D, Thor Straten P, Becker JC. Cytotoxic T cells. J Invest Dermatol (2006) 126:32–41. doi:10.1038/sj.jid.5700001
107. De La Roche M, Asano Y, Griffiths GM. Origins of the cytolytic synapse. Nat Rev Immunol (2016) 16:421–32. doi:10.1038/nri.2016.54
108. Davignon D, Martz E, Reynolds T, Kurzinger K, Springer TA. Lymphocyte function-associated antigen 1 (LFA-1): a surface antigen distinct from Lyt-2,3 that participates in T lymphocyte-mediated killing. Proc Natl Acad Sci U S A (1981) 78:4535–9. doi:10.1073/pnas.78.7.4535
109. Petit AE, Demotte N, Scheid B, Wildmann C, Bigirimana R, Gordon-Alonso M, et al. A major secretory defect of tumour-infiltrating T lymphocytes due to galectin impairing LFA-1-mediated synapse completion. Nat Commun (2016) 7:12242. doi:10.1038/ncomms12242
110. Sanderson CJ. The mechanism of T-cell mediated cytotoxicity. VIII. Zeiosis corresponds to irreversible phase (programming for lysis) in steps leading to lysis. Immunology (1981) 42:201–6.
111. Halle S, Keyser KA, Stahl FR, Busche A, Marquardt A, Zheng X, et al. In vivo killing capacity of cytotoxic T cells is limited and involves dynamic interactions and T cell cooperativity. Immunity (2016) 44:233–45. doi:10.1016/j.immuni.2016.01.010
112. Halle S, Halle O, Forster R. Mechanisms and dynamics of T cell-mediated cytotoxicity in vivo. Trends Immunol (2017) 38:432–43. doi:10.1016/j.it.2017.04.002
113. Kohl S, Springer TA, Schmalstieg FC, Loo LS, Anderson DC. Defective natural killer cytotoxicity and polymorphonuclear leukocyte antibody-dependent cellular cytotoxicity in patients with LFA-1/OKM-1 deficiency. J Immunol (1984) 133:2972–8.
114. Krensky AM, Mentzer SJ, Clayberger C, Anderson DC, Schmalstieg FC, Burakoff SJ, et al. Heritable lymphocyte function-associated antigen-1 deficiency: abnormalities of cytotoxicity and proliferation associated with abnormal expression of LFA-1. J Immunol (1985) 135:3102–8.
115. Schmits R, Kundig TM, Baker DM, Shumaker G, Simard JJ, Duncan G, et al. LFA-1-deficient mice show normal CTL responses to virus but fail to reject immunogenic tumor. J Exp Med (1996) 183:1415–26. doi:10.1084/jem.183.4.1415
116. Kuhn JR, Poenie M. Dynamic polarization of the microtubule cytoskeleton during CTL-mediated killing. Immunity (2002) 16:111–21. doi:10.1016/S1074-7613(02)00262-5
117. Somersalo K, Anikeeva N, Sims TN, Thomas VK, Strong RK, Spies T, et al. Cytotoxic T lymphocytes form an antigen-independent ring junction. J Clin Invest (2004) 113:49–57. doi:10.1172/JCI19337
118. Franciszkiewicz K, Le Floc’h A, Boutet M, Vergnon I, Schmitt A, Mami-Chouaib F. CD103 or LFA-1 engagement at the immune synapse between cytotoxic T cells and tumor cells promotes maturation and regulates T-cell effector functions. Cancer Res (2013) 73:617–28. doi:10.1158/0008-5472.CAN-12-2569
119. Anikeeva N, Somersalo K, Sims TN, Thomas VK, Dustin ML, Sykulev Y. Distinct role of lymphocyte function-associated antigen-1 in mediating effective cytolytic activity by cytotoxic T lymphocytes. Proc Natl Acad Sci U S A (2005) 102:6437–42. doi:10.1073/pnas.0502467102
120. Schneider H, Valk E, Da Rocha Dias S, Wei B, Rudd CE. CTLA-4 up-regulation of lymphocyte function-associated antigen 1 adhesion and clustering as an alternate basis for coreceptor function. Proc Natl Acad Sci U S A (2005) 102:12861–6. doi:10.1073/pnas.0505802102
121. Kloog Y, Mor A. Cytotoxic-T-lymphocyte antigen 4 receptor signaling for lymphocyte adhesion is mediated by C3G and Rap1. Mol Cell Biol (2014) 34:978–88. doi:10.1128/MCB.01024-13
122. Hviid L, Odum N, Theander TG. The relation between T-cell expression of LFA-1 and immunological memory. Immunology (1993) 78:237–43.
123. Parameswaran N, Suresh R, Bal V, Rath S, George A. Lack of ICAM-1 on APCs during T cell priming leads to poor generation of central memory cells. J Immunol (2005) 175:2201–11. doi:10.4049/jimmunol.175.4.2201
124. Helft J, Jacquet A, Joncker NT, Grandjean I, Dorothee G, Kissenpfennig A, et al. Antigen-specific T-T interactions regulate CD4 T-cell expansion. Blood (2008) 112:1249–58. doi:10.1182/blood-2007-09-114389
125. McNamara HA, Cai Y, Wagle MV, Sontani Y, Roots CM, Miosge LA, et al. Up-regulation of LFA-1 allows liver-resident memory T cells to patrol and remain in the hepatic sinusoids. Sci Immunol (2017) 2:eaaj1996. doi:10.1126/sciimmunol.aaj1996
126. Badell IR, Russell MC, Thompson PW, Turner AP, Weaver TA, Robertson JM, et al. LFA-1-specific therapy prolongs allograft survival in rhesus macaques. J Clin Invest (2010) 120:4520–31. doi:10.1172/JCI43895
127. Setoguchi K, Schenk AD, Ishii D, Hattori Y, Baldwin WM, Tanabe K, et al. LFA-1 antagonism inhibits early infiltration of endogenous memory CD8 T cells into cardiac allografts and donor-reactive T cell priming. Am J Transplant (2011) 11:923–35. doi:10.1111/j.1600-6143.2011.03492.x
128. Kitchens WH, Haridas D, Wagener ME, Song M, Kirk AD, Larsen CP, et al. Integrin antagonists prevent costimulatory blockade-resistant transplant rejection by CD8(+) memory T cells. Am J Transplant (2012) 12:69–80. doi:10.1111/j.1600-6143.2011.03762.x
129. Kwun J, Farris AB, Song H, Mahle WT, Burlingham WJ, Knechtle SJ. Impact of leukocyte function-associated antigen-1 blockade on endogenous allospecific T cells to multiple minor histocompatibility antigen mismatched cardiac allograft. Transplantation (2015) 99:2485–93. doi:10.1097/TP.0000000000000805
130. Ray SJ, Franki SN, Pierce RH, Dimitrova S, Koteliansky V, Sprague AG, et al. The collagen binding alpha1beta1 integrin VLA-1 regulates CD8 T cell-mediated immune protection against heterologous influenza infection. Immunity (2004) 20:167–79. doi:10.1016/S1074-7613(04)00021-4
131. Chapman TJ, Topham DJ. Identification of a unique population of tissue-memory CD4+ T cells in the airways after influenza infection that is dependent on the integrin VLA-1. J Immunol (2010) 184:3841–9. doi:10.4049/jimmunol.0902281
132. Chang JT, Palanivel VR, Kinjyo I, Schambach F, Intlekofer AM, Banerjee A, et al. Asymmetric T lymphocyte division in the initiation of adaptive immune responses. Science (2007) 315:1687–91. doi:10.1126/science.1139393
133. Chang JT, Ciocca ML, Kinjyo I, Palanivel VR, Mcclurkin CE, Dejong CS, et al. Asymmetric proteasome segregation as a mechanism for unequal partitioning of the transcription factor T-bet during T lymphocyte division. Immunity (2011) 34:492–504. doi:10.1016/j.immuni.2011.03.017
134. Kong F, Garcia AJ, Mould AP, Humphries MJ, Zhu C. Demonstration of catch bonds between an integrin and its ligand. J Cell Biol (2009) 185:1275–84. doi:10.1083/jcb.200810002
135. Chen W, Lou J, Zhu C. Forcing switch from short- to intermediate- and long-lived states of the alphaA domain generates LFA-1/ICAM-1 catch bonds. J Biol Chem (2010) 285:35967–78. doi:10.1074/jbc.M110.155770
136. Nordenfelt P, Elliott HL, Springer TA. Coordinated integrin activation by actin-dependent force during T-cell migration. Nat Commun (2016) 7:13119. doi:10.1038/ncomms13119
137. Nordenfelt P, Moore TI, Mehta SB, Kalappurakkal JM, Swaminathan V, Koga N, et al. Direction of actin flow dictates integrin LFA-1 orientation during leukocyte migration. Nat Commun (2017) 8:2047. doi:10.1038/s41467-017-01848-y
138. Lafouresse F, Cotta-De-Almeida V, Malet-Engra G, Galy A, Valitutti S, Dupre L. Wiskott-Aldrich syndrome protein controls antigen-presenting cell-driven CD4+ T-cell motility by regulating adhesion to intercellular adhesion molecule-1. Immunology (2012) 137:183–96. doi:10.1111/j.1365-2567.2012.03620.x
139. Houmadi R, Guipouy D, Rey-Barroso J, Vasconcelos Z, Cornet J, Manghi M, et al. The Wiskott-Aldrich syndrome protein contributes to the assembly of the LFA-1 nanocluster belt at the lytic synapse. Cell Rep (2018) 22:979–91. doi:10.1016/j.celrep.2017.12.088
140. Na BR, Kim HR, Piragyte I, Oh HM, Kwon MS, Akber U, et al. TAGLN2 regulates T cell activation by stabilizing the actin cytoskeleton at the immunological synapse. J Cell Biol (2015) 209:143–62. doi:10.1083/jcb.201407130
141. Comrie WA, Babich A, Burkhardt JK. F-actin flow drives affinity maturation and spatial organization of LFA-1 at the immunological synapse. J Cell Biol (2015) 208:475–91. doi:10.1083/jcb.201406121
142. Basu R, Whitlock BM, Husson J, Le Floc’h A, Jin W, Oyler-Yaniv A, et al. Cytotoxic T cells use mechanical force to potentiate target cell killing. Cell (2016) 165:100–10. doi:10.1016/j.cell.2016.01.021
143. Basu R, Huse M. Mechanical communication at the immunological synapse. Trends Cell Biol (2017) 27:241–54. doi:10.1016/j.tcb.2016.10.005
144. Sun Z, Guo SS, Fassler R. Integrin-mediated mechanotransduction. J Cell Biol (2016) 215:445–56. doi:10.1083/jcb.201609037
Keywords: T cell migration, integrins, LFA-1, chemokines, T cell activation, T cell differentiation
Citation: Walling BL and Kim M (2018) LFA-1 in T Cell Migration and Differentiation. Front. Immunol. 9:952. doi: 10.3389/fimmu.2018.00952
Received: 13 December 2017; Accepted: 17 April 2018;
Published: 03 May 2018
Edited by:
Andrea Sant, David H. Smith Center for Vaccine Biology and Immunology, United StatesReviewed by:
Craig Michael Walsh, University of California, Irvine, United StatesLoïc Dupré, Institut National de la Santé et de la Recherche Médicale (INSERM), France
Copyright: © 2018 Walling and Kim. This is an open-access article distributed under the terms of the Creative Commons Attribution License (CC BY). The use, distribution or reproduction in other forums is permitted, provided the original author(s) and the copyright owner are credited and that the original publication in this journal is cited, in accordance with accepted academic practice. No use, distribution or reproduction is permitted which does not comply with these terms.
*Correspondence: Minsoo Kim, minsoo_kim@urmc.rochester.edu