- 1Department of Graduate and Scientific Research, Zhuhai Campus of Zunyi Medical University, Zhuhai, China
- 2Department of Otolaryngology, Longgang Otolaryngology Hospital & Shenzhen Key Laboratory of Otolaryngology, Institute of Otolaryngology Shenzhen, Shenzhen, China
- 3Department of Gastroenterology, Beijing University of Chinese Medicine Shenzhen Hospital (Longgang), Shenzhen, China
- 4Clinical Laboratory Department of The Second Affiliated Hospital, School of Medicine, The Chinese University of Hong Kong, Shenzhen & Longgang District People’s Hospital of Shenzhen, Shenzhen, China
The epithelial barrier serves as a critical defense mechanism separating the human body from the external environment, fulfilling both physical and immune functions. This barrier plays a pivotal role in shielding the body from environmental risk factors such as allergens, pathogens, and pollutants. However, since the 19th century, the escalating threats posed by environmental pollution, global warming, heightened usage of industrial chemical products, and alterations in biodiversity have contributed to a noteworthy surge in allergic disease incidences. Notably, allergic diseases frequently exhibit dysfunction in the epithelial barrier. The proposed epithelial barrier hypothesis introduces a novel avenue for the prevention and treatment of allergic diseases. Despite increased attention to the role of barrier dysfunction in allergic disease development, numerous questions persist regarding the mechanisms underlying the disruption of normal barrier function. Consequently, this review aims to provide a comprehensive overview of the epithelial barrier’s role in allergic diseases, encompassing influencing factors, assessment techniques, and repair methodologies. By doing so, it seeks to present innovative strategies for the prevention and treatment of allergic diseases.
1 Introduction
Allergic diseases, characterized by inflammatory responses mediated by type 2 immune reactions, encompass conditions such as asthma, atopic rhinitis (AR), atopic dermatitis (AD), and food allergy (FA), arising from the intricate interplay of genetic and environmental factors (1). Since the 19th century, the intensification of industrialization and modernization has contributed to escalating environmental pollution, particularly in urban areas, exerting a pronounced impact on human health (2). Current research unequivocally affirms the pivotal role of environmental factors in the initiation and progression of allergic diseases. The adverse effects of industrialization, urbanization, and modernization on the environment, spanning air pollution, global warming, climate alterations, and biodiversity depletion, have led to a marked surge in allergic disease prevalence (2, 3). Recent studies underscore the involvement of the epithelial barrier in the pathogenesis of allergic diseases. In 2017, Pothoven and Schleimer introduced the epithelial barrier hypothesis for type 2 inflammatory diseases, positing that dysfunction in the epithelial barrier could catalyze the development of allergic diseases (4). Akdis subsequently expanded upon this hypothesis, suggesting that alterations in the environment and lifestyle resulting from industrialization and urbanization disrupt the epithelial barrier across the skin, upper and lower respiratory tracts, and intestinal mucosa. Epithelial barrier compromise leads to microbial dysregulation, bacterial translocation between epithelial and subepithelial regions, and the onset of tissue microinflammation. Epithelial barrier impairment not only forms the foundation for allergic and autoimmune diseases but also underlies a spectrum of conditions that elicit immunoinflammatory responses to bacteria, viruses, and opportunistic pathogens (5). Current research categorizes diseases marked by compromised epithelial barriers into three main types: 1) chronic conditions stemming from localized barrier defects, resulting in histopathological changes in affected skin and mucous membranes, such as allergic diseases and inflammatory bowel disease (6–8); 2) chronic autoimmune and metabolic diseases, including diabetes, multiple sclerosis, autoimmune hepatitis, etc. (9–12); and 3) Neurodegenerative diseases linked to intestinal barrier deficits and microbial translocations, such as Parkinson’s disease and Alzheimer’s disease (13, 14).
The epithelial barrier hypothesis introduces a novel avenue for investigating the prevention and treatment of allergic diseases. Despite the emphasis on the role of barrier dysfunction in the pathogenesis of allergic diseases, numerous questions persist regarding the mechanisms underlying the disruption of normal barrier function. Therefore, the objective of this review is to furnish a comprehensive overview of the epithelial barrier’s role in allergic diseases, encompassing influencing factors, evaluation techniques, and repair methodologies. By doing so, it aims to pave the way for exploring innovative therapeutic strategies for allergic diseases.
2 Epithelial barrier dysfunction and allergic diseases
2.1 The epithelial barrier
2.1.1 Composition of the epithelial barrier
The epithelial barriers of the skin, gastrointestinal system, and respiratory tract exhibit both similarities and differences in terms of structure, function, and biochemical properties. For instance, the skin provides a robust physical barrier, while the epithelial ciliary movement in the airways continuously clears particulate matter, facilitating gas exchange. In the gastrointestinal system, the epithelium facilitates a broad range of nutrient and water exchange while concurrently offering protection against microbes, toxins, and environmental exposures (15). Consequently, epithelial tissues serve multifaceted roles encompassing physical, chemical, and immune barrier functions. Notably, the epithelial cells at each site collaboratively form a cohesive epithelial barrier through cellular connections (Figure 1). These connections are established by tight junctions (TJs), adherens junctions (AJs), and desmosome (16). AJs consist of diverse components such as E-cadherin, actinin, vinculin, α-catenin, and β-catenin, while TJs form a complex involving claudins, occludins, and junctional adhesion molecules (17). TJs create a barrier by sealing the apical boundary, preventing the unhindered entry of microorganisms, toxins, and pollutants, and regulating the paracellular transport of ions and certain small molecules (18). AJs play a crucial role in initiating and maintaining intercellular adhesion, contributing to the establishment and regulation of the apicobasolateral membrane structure (18). Desmosomes, characterized by a symmetrical structure comprising two adjacent plasma membranes separated by a 30-nanometer cellular gap, possess a central dense layer or midline flanked by mirrored triple-electron dense plaques (19). Desmosomes play a pivotal role in establishing and maintaining stable cell junctions.
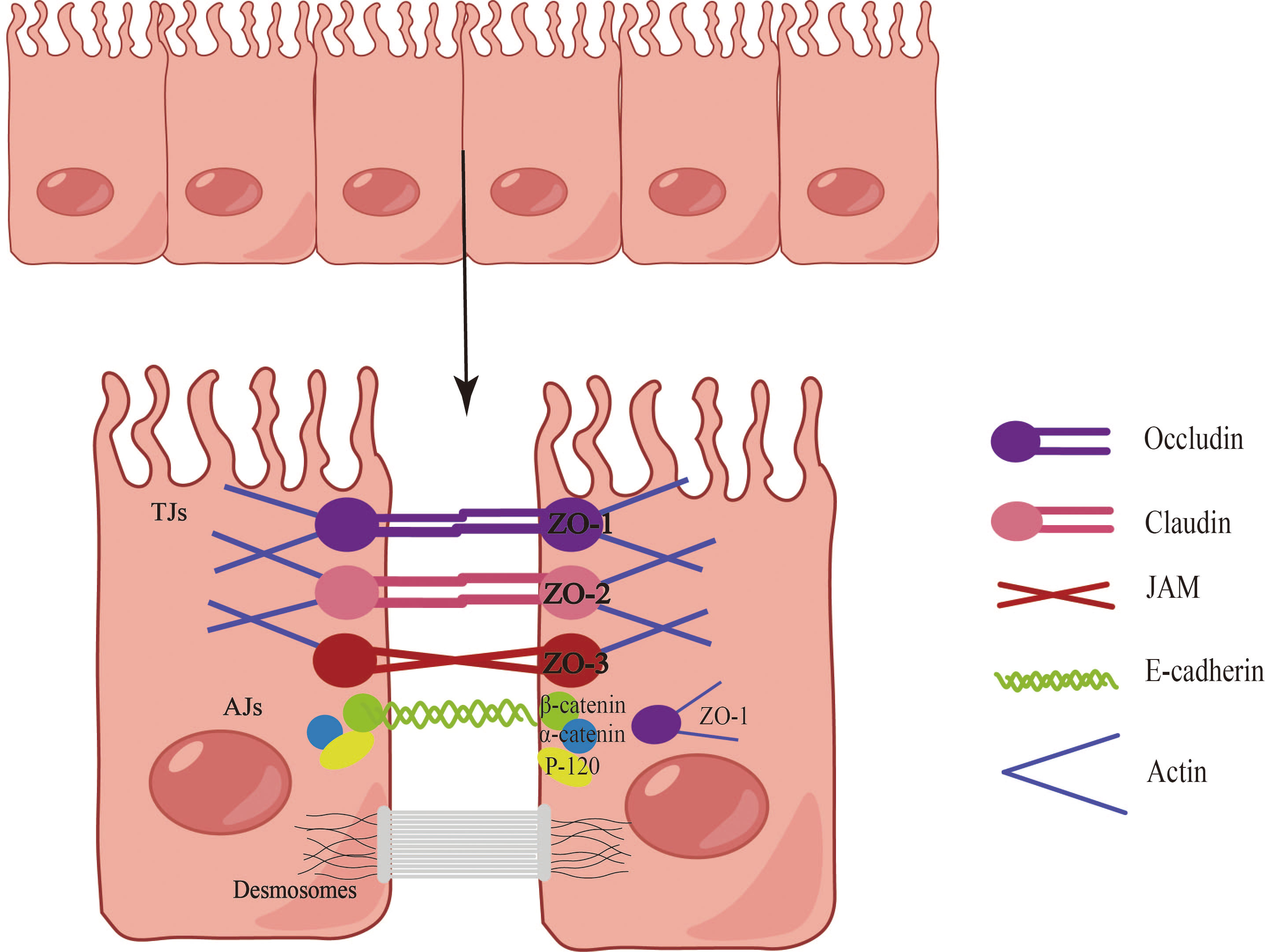
Figure 1 Composition of the epithelial barrier. Epithelial cells establish barriers through the intricate interplay of tight junctions (TJs), adherens junctions (AJs), and desmosomes. The TJ complex is comprised of claudins, occludins, and junctional adhesion molecules, with major cytoplasmic proteins including ZO-1, ZO-2, and ZO-3. AJs, positioned directly below the TJ, encompass E-cadherin, actin, vinculin, α-catenin, and β-catenin. Desmosomes, characterized by a symmetrical structure involving two adjacent plasma membranes, play a vital role in establishing and maintaining stable cellular junctions.
2.1.2 Epithelial barrier and type 2 immune response
Disruption of the epithelial barrier emerges as a common pathway contributing to the initiation and exacerbation of allergic diseases (20, 21). Research has unequivocally established the involvement of the epithelial barrier in the pathological progression of allergic diseases (5). Dysfunction in the epithelial barrier across various human tissues is characterized by cell differentiation, compromised junction integrity, and impaired innate defenses. Genetic predisposition, environmental influences, and aberrant inflammation collectively promote the dysfunction and breakdown of the epithelial barrier, thereby precipitating the onset and progression of allergic diseases (22). Studies indicate that compromised epithelial barriers activate epithelial cells, leading to the release of alarm factors such as interleukin-25 (IL-25), IL-33, and thymic stromal lymphopoietin (TSLP). This, in turn, promotes cytokine release by type 2 innate immune cells, triggering and exacerbating type 2 immune responses (23, 24). Epithelial cells play a pivotal role in the initiation and exacerbation of allergic diseases. Key cytokines, including IL-4, IL-13, IL-5, IL-9, TSLP, and IL-33, feature prominently in allergic diseases, as they stimulate the development of inflammatory responses and tissue repair (25–27). However, inflammatory cytokines such as IL-4, IL-3, IFN-γ, and TNF-α have been demonstrated to disrupt epithelial barrier function through various mechanisms. In primary bronchial epithelial cells (PBEC) cultured at the gas-liquid interface, IFN-γ and TNF-α collaboratively impair barrier function, leading to decreased expression of ZO-1 and JAM (28). IL-4 and IL-13 induce epithelial barrier dysfunction by inhibiting the expression of ZO-1, occludin, E-cadherin, and β-catenin (Figure 2) (29).
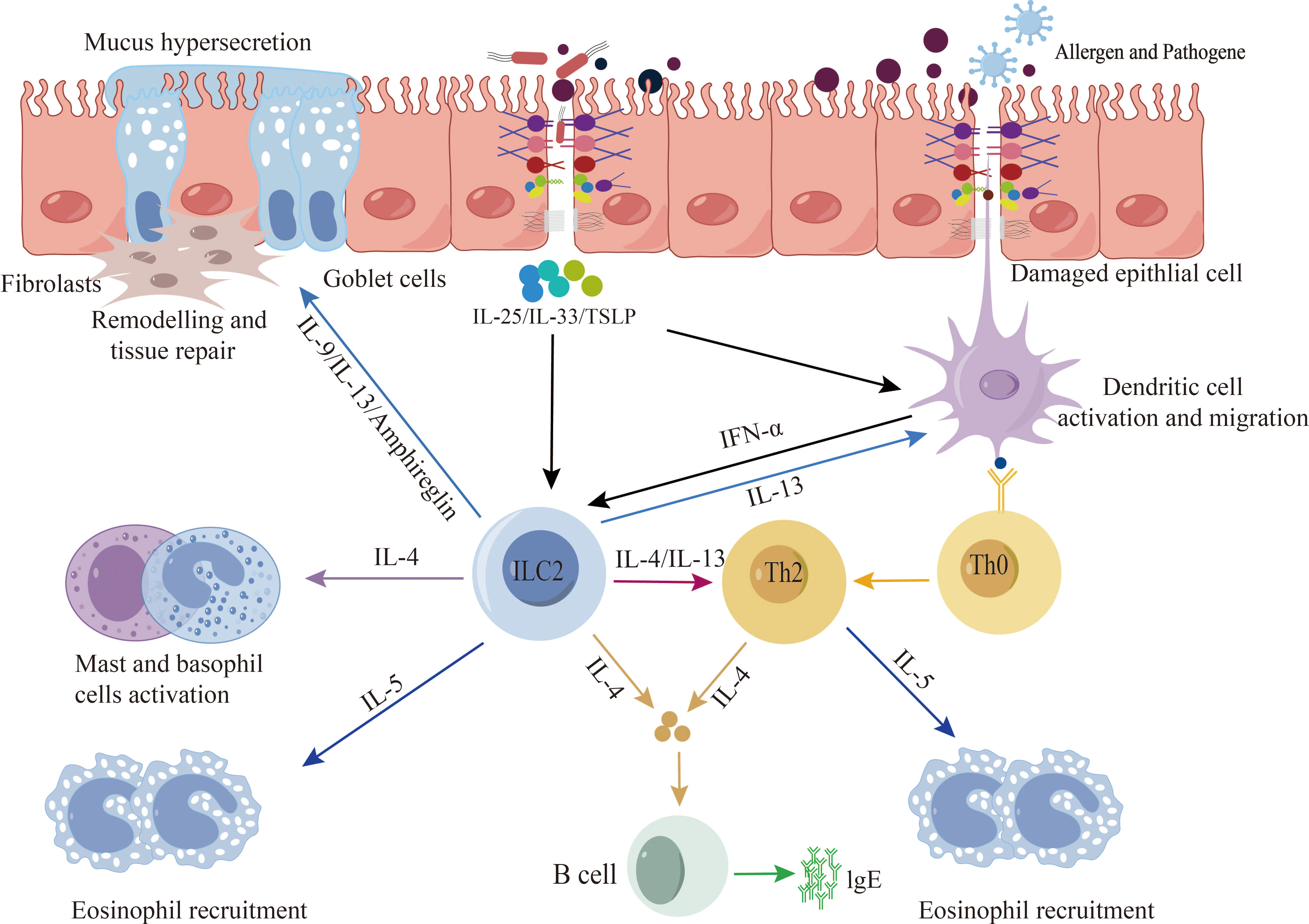
Figure 2 Epithelial barrier and type 2 immune response. When exposed to allergens, pathogenic bacteria, pollutants, etc., a compromised epithelial barrier undergoes activation, leading to the release of alarm factors by epithelial cells, including interleukin-25 (IL-25), IL-33, and thymic stromal lymphopoietin (TSLP). Additionally, activation may occur through the stimulation of dendritic cells, subsequently presenting antigens to Th2 cells and ILC2s cells. This activation process promotes the release of type 2 cytokines, thereby triggering and exacerbating type 2 immune responses.
2.2 Epithelial barrier dysfunction and allergic diseases
2.2.1 Epithelial barrier dysfunction and asthma
Asthma, characterized as a chronic inflammatory disease of the airways, heavily relies on the integrity of respiratory epithelial cells, which collectively establish a physical, functional, and immune barrier. This barrier is crucial in safeguarding the host from potential hazards associated with inhaled environmental risk factors, thereby maintaining the overall health of the host. Prolonged exposure to external stimuli, however, can lead to the manifestation of epithelial barrier dysfunction within the asthma phenotype (30). The human airway epithelium is composed of predominant ciliated epithelial cells, mucous-secreting goblet cells, club cells, and airway basal cells (6). In a healthy airway, TJs and AJs establish connections between epithelial cells, forming a closed barrier that effectively prevents the invasion of risk factors. This cell barrier is naturally coated with mucus containing antimicrobial agents, peptides, and antibodies, serving as a protective measure against the intrusion of pathogenic bacteria and allergens (17, 31). However, patients with asthma often exhibit airway epithelial barrier disorders characterized by an increase in basal and goblet cells and a decrease in terminally differentiated ciliated cell (32), This is frequently accompanied by basement membrane thickening and epithelial exfoliation, resulting in the formation of Creola bodies composed of exfoliated epithelial clusters (4). Research indicates that during the course of asthma pathology, the number of MUC5B decreases, while the expression of MUC5AC increases, leading to mucus overproduction, airway obstruction, and the accumulation of mucus materials, ultimately inducing chronic inflammation (33). In addition, studies highlight the disruption of tight junctions and adherent junctions in the epithelial barrier as typical features of asthma. Similarly, deficiencies and dysfunction of E-cadherin, α-catenin, ZO-1, and occludin have been identified in patients with asthma (34), resulting in impaired barrier function. Moreover, a damaged airway epithelial barrier activates cytokines (IL-4, IL-5, and IL-13) released by ILC2s, further exacerbating the damage to the epithelial barrier (20, 35). Beyond the damage to epithelial barrier function caused by inflammation in asthma, several recent studies indicate the potential existence of barrier function abnormalities in airway epithelial cells in asthma patients (36). Further in-depth research is still needed to elucidate the mechanism of action of the epithelial barrier during asthma pathogenesis.
In summary, sustained exposure to risk factors such as allergens, pathogenic bacteria, and viruses, coupled with immune inflammatory responses, can lead to severe damage to epithelial barrier components. This damage results in the disruption of epithelial barrier function and structure, ultimately increasing barrier permeability and contributing to the onset and exacerbation of asthma.
2.2.2 Epithelial barrier dysfunction and AR
AR, characterized as an inflammatory condition affecting the nasal mucosa and mediated by allergens, exhibits an escalating incidence, particularly in industrialized and modernized regions, influenced by diverse factors. Recent investigations have brought attention to the role of nasal mucosal epithelium in the pathogenesis of AR (6). The nasal epithelium, acting as the primary interface for allergens, pollutants, and pathogens, anatomically comprises two segments: the initial third of the nasal cavity features stratified squamous epithelium, while the remaining two-thirds possess a pseudostratified columnar ciliary epithelium with goblet cells covering the basement membrane (17). In a healthy state, coordinated ciliary movement and mucus secretion constitute the primary defense mechanism, preventing compromise of the nasal mucosal barrier by various risk or irritant factors. However, in the pathological context of allergic rhinitis, the central role played by the dysfunction and disruption of TJs becomes evident, compromising the integrity of the nasal epithelial barrier and subsequently hindering ciliary motility and mucus secretion (37, 38). Under normal conditions, nasal epithelial cells are bound together by TJs, forming a complex known as an occlusion zone (ZO), inclusive of claudin, occludin, and junction adhesion molecules (37). Notably, studies have revealed diminished expression and immunoreactivity of E-cadherin and ZO-1 in the nasal epithelium of individuals with allergic rhinitis (39). Furthermore, Protease-activated receptor-2 (PAR-2) has been implicated in diminishing the expression of ZO-1 and claudin-1, with the latter involved in epithelial barrier dysfunction in AR (40). Subsequent in vitro assessments of barrier function using primary epithelial cells from AR patients demonstrated diminished barrier function and reduced expression of tight junction proteins occludin and ZO-1 in comparison to healthy controls (41). Additionally, type 2 cytokines (IL-4, IL-5, IL-13, TNF-α, etc.) produced during immune-inflammatory responses can directly impact the nasal epithelial barrier, resulting in decreased expression of ZO-1 and E-cadherin (28, 39). Furthermore, proteases from certain allergens have been demonstrated to disrupt epithelial TJs and induce AR disease (4).
In summary, current studies suggest a pervasive disruption of epithelial barrier function and increased permeability in AR patients. This accelerates the penetration of allergens through the nasal epithelial barrier, contributing to the onset and development of AR. Multiple pathways in the pathogenic process of AR lead to damage to the epithelial barrier, necessitating further in-depth research to comprehensively understand the underlying mechanisms.
2.2.3 Epithelial barrier dysfunction and AD
AD stands as the most prevalent chronic inflammatory skin disease influenced by a combination of genetic and environmental factors (42, 43). Current insights into AD pathogenesis encompass skin barrier dysfunction, epigenetic alterations, immune factors, skin, and intestinal dysbiosis, alongside interactions with external risk factors (2, 44, 45). The pathology of AD is distinguished by a disruption of skin barrier function. The human epidermis comprises the stratum basale (SB), stratum spinosum (SS), stratum granulosum (SG), and stratum corneum (SC) (43). The SC, situated as the outermost layer above keratinocytes and Langerhans cells (LC), plays a pivotal role in skin barrier function (46). Studies reveal that the SC consists of proteins such as filaggrin, involucrin, loricin, and an outer lipid layer. Particularly, filaggrin, encoded by the FLG gene, serves as the primary source of natural moisturizing factor (NMF) in SC (47). Mutations in FLG can significantly reduce NMF in AD patients, closely correlating with disease severity and emphasizing NMF reduction as a common feature in AD (47, 48). Currently, FLG gene mutations resulting in epithelial dysfunction stand as the most prevalent and severe genetic risk factor for AD (49). Notably, epithelial injury can trigger innate immune responses, activating the release of pro-inflammatory cytokines and chemokines by keratinocytes, and enhancing antigen presentation by Langerhans cells and dermal dendritic cells. Even in subjects with FLG null-mutation, type 2 immune inflammation can lead to decreased FLG expression in AD-afflicted skin (50–52). Additionally, FLG mutations elevate skin pH and activate skin proteases, inducing skin barrier dysfunction (53). Additionally, FLG mutations elevate skin pH and activate skin proteases, inducing skin barrier dysfunction (54). Jungersted et al. have indicated that the pathogenesis of AD extends beyond FLG mutations (55). During epidermal differentiation, keratinocytes sequentially alter gene expression programs, culminating in terminal differentiation and the formation of a mature corneal layer. In AD-afflicted skin, the loss of corneodesmosin disrupts barrier lipids, impairing skin barrier and antimicrobial function (56). Furthermore, AD alters other proteins in the corneal layer, including corneal adhesion, loricrin (LOR), and involucrin (IVL), resulting in compromised skin barrier function and type 2 immune response (56–59). Additionally, lipids such as ceramides are reduced, and the release of inflammatory cytokines during allergic reactions can further decrease the lipid content of SC, exacerbating barrier dysfunction in AD patients (60–62). Various environmental factors contribute to the disruption of skin barrier function, including detergents, microplastics, particulate matter (PM), etc., confirmed to alter the integrity of the skin barrier (51). Specifically, SC tight junctions can be disrupted by anionic surfactants in commercial detergents, while other components can release inflammatory cytokines that aggravate impairment of barrier function (1, 63). Furthermore, dysregulation of the skin microbiome exacerbates damage to the skin’s barrier function (51, 64, 65). Analyses of bacterial communities in AD states reveal that Staphylococcus aureus colonization contributes to the pathogenesis and aggravation of AD, with intestinal microbiota dysbiosis also considered a significant factor in AD pathogenesis (1, 66). According to existing research, AD can progress from skin diseases to food allergies, allergic rhinitis, and later asthma, a phenomenon commonly referred to as the atopic march (43, 67, 68).
In summary, the skin structure is compromised by a range of environmental and genetic factors, including FLG mutations, skin microbiota colonization, intestinal microbial dysbiosis, and environmental pollution hazards. Skin barrier dysfunction allows exposure to allergens, bacteria, and fungi, triggering immune responses and leading to the onset of AD. Moreover, the progression of AD can further induce the development of allergic diseases such as allergic rhinitis and food allergy.
2.2.4 Epithelial barrier dysfunction and FA
FA represents an immune-inflammatory-mediated adverse reaction to specific foods (69, 70). The underlying mechanism across various food allergies involves immune activation and the disruption of tolerance to the intake of specific foods. Dysfunctions of the gastrointestinal tract and skin barriers can contribute to food sensitivities (71). Notably, CD103+ DCs mediate immune tolerance in the gastrointestinal tract, while CD11b+ dermal DCs and Langerhans cells (LCs) mediate cutaneous tolerance (72, 73). In the pathogenic process of food allergy, impaired development of regulatory T (Treg) cells is observed, being replaced by the production of helper T2 (Th2) cells. These Th2 cells drive IgE conversion and the expansion of allergic effector cells, thereby exacerbating the immune response associated with food allergy (72). The intestinal epithelial barrier is not a static physical barrier but maintains a dynamic balance with the intestinal microbiome and immune cells (74). Comprising a single layer of columnar epithelium interspersed with goblet cells, encased by a layer of mucus, the intestinal barrier protects the host from penetration by digestive enzymes and microorganisms (67). Intestinal epithelial cells selectively allow the absorption of nutrients, electrolytes, and water while defending against the invasion of harmful microorganisms and toxins (75). The mucus layer, the outermost part of the intestinal epithelium, effectively blocks harmful substances and tissues (74). It consists of an outermost mucus rich in antimicrobial peptides and immunoglobulin A, and an inner layer of mucus adhering directly to neighboring epithelial cells (74, 76). The inner layer of mucus, primarily composed of glycocalyx produced by goblet cells, prevents antigens from invading the lamina propria of the intestinal mucosa (74, 77). Moreover, studies have highlighted the role of TJs as a key component in controlling intestinal barrier permeability in epithelial cell junctions (76). In the context of FA, impaired function of the intestinal epithelial intercellular junctions leads to barrier dysfunction and loss of osmotic function. This results in increased permeability, allowing allergens to penetrate the intestinal barrier and stimulate the submucosal immune system. Damaged epithelial cells release inflammatory cytokines, such as TSLP, IL-25, and IL-33, inducing cutaneous DCs and other cell types to shift the immune response from tolerance to hypersensitivity. This process is involved in allergic sensitization and triggering reactions by activating innate lymphoid cells type 2 (ILC2) and producing IL-4 and IL-13 (69).
In summary, when stimulated by allergens, epithelial cells release alarmins, activating immune cells and antigen-presenting cells. This promotes the occurrence of immune responses and the release of cytokines and inflammatory mediators, further exacerbating the destruction of the epithelial barrier. This, in turn, increases the permeability of the intestinal barrier, forming a vicious circle and aggravating the degree of food allergy disease (78).
2.2.5 Microbial dysbiosis and the epithelial barrier in allergic diseases
With the advancement of industrialization, modernization, and urbanization, various factors such as environmental pollution, increased consumption of processed foods, reduced contact with animals, and excessive hygiene practices have contributed to a rising incidence of allergic diseases. Changes in the environment, health, and lifestyles have significantly impacted microbial diversity and homeostasis. Recent research indicates that alterations in the gut, oral, and skin microbiota may trigger immune-mediated diseases, including autoimmunity, allergies, and chronic inflammatory conditions (79). Host-microbiota interactions play a fundamental role in immune system development (79). The local immune system not only adapts to the presence of host-beneficial microbiota, maintaining internal homeostasis, but also responds appropriately to pathogenic microorganisms (79). Microbial dysbiosis, a key focus in the field of epithelial barrier dysfunction (5, 80). refers to the phenomenon where changes and loss of biodiversity result in an unstable microbial ecosystem dominated by one or several microorganisms. This alteration disrupts the immune balance maintained by the gut, skin, and respiratory microbiota, leading to disease development (81, 82). Beneficial microbiota on mucosal surfaces regulating various aspects of barrier homeostasis, including barrier permeability, TJ expression, angiogenesis, local microinflammation, and mucosal tolerance (2). However, microbial dysregulation and epithelial barrier leakage can disturb immune homeostasis. When the epithelial barrier is compromised, the microbiota within the epithelium (beneficial flora, viruses, conditionally pathogenic bacteria, etc.) can migrate between affected epithelial cells or transfer to other sites (11, 83, 84). The occurrence and development of allergic diseases are closely linked to the imbalance of microbial flora in the epithelium. For instance, studies have demonstrated an association between microbiota dysbiosis and asthma (85). Multiple risk factors contribute to asthma development, with dysregulation of external microorganisms and host microbiota playing a significant role (86). Host microorganisms participate in asthma pathogenesis through the metabolites of gut microbes, such as Lachnospira, Veillonella, Faecalibacterium, and Rothia (87). The “gut-lung axis” proposes that metabolites produced by gut-derived microbiota reach the lungs, shifting the Th2-Treg balance to Treg, protecting against asthma development (79). Similarly, the composition and function of upper respiratory tract microbiota influence asthma pathogenesis, with variations observed at different ages (88). Microbial flora dysbiosis is also implicated in allergic rhinitis (AR). Studies have noted changes in nasal mucosal microbiota associated with AR, including increased abundance of Staphylococcus aureus, Propionibacterium, Corynebacterium, and Bacteroidetes, and decreased abundance of Prevotella and Streptococcus (89). Environmental microbiota exposure in early life may be biologically linked to allergic manifestations, emphasizing the importance of microbial interactions in AR pathogenesis (90). The skin, as the body’s largest organ, is in constant contact with the external environment and harbors a variety of microorganisms between epithelial cells. Disruption of skin function increases skin leakage, allowing resident bacteria and opportunistic pathogens to enter epithelial tissue, inducing inflammatory responses or exerting protective effects. Studies suggest that decreased microbiome diversity in AD patients is associated with increased disease severity and colonization of pathogenic bacteria such as Staphylococcus aureus. Topical application of commensal organisms can potentially reduce AD severity (51, 91). The gastrointestinal tract, closely interacting with environmental microorganisms through food ingestion and excretion, is rich in microbiota crucial for maintaining body microenvironment homeostasis. However, damage to the intestinal epithelial barrier increases mucosal permeability, allowing the invasion of pathogenic bacteria and causing diseases (92). Dynamic changes in gut microbiota are implicated in the development of FA (93), with age and diet influencing intestinal flora abundance and FA occurrence (94).
In summary, the interaction between dysbiosis of the microbiota and epithelial barrier dysfunction can contribute to the occurrence and progression of allergic diseases. Microbial dysbiosis alters the microenvironment balance maintained by microorganisms in respiratory tract, skin, and intestinal tissues, leading to increased epithelial barrier permeability, heightened sensitivity to allergens, and the onset and exacerbation of various allergic diseases.
3 Factors that cause epithelial barrier damage
With the ongoing processes of modernization, urbanization, and industrialization, various factors have emerged as potential inducers and exacerbators of allergic diseases. These include climate change, environmental pollution, dietary habits, and biodiversity loss, among others. Exposure to these factors can lead to alterations in the structure of the epithelial barrier, impacting the development of allergic diseases by disrupting normal immunomodulation. Numerous studies highlight the prevalent role of impaired epithelial barrier function in the pathogenesis of allergic diseases, emphasizing that the function of the epithelial barrier influences both innate and adaptive immune responses. Currently, a wealth of research has identified several risk factors contributing to epithelial barrier damage in the contemporary environment (18, 95), and detailed information is shown in Table 1.
3.1 Environment factors and the epithelial barrier
3.1.1 Particulate matter
Particulate matter (PM), also known as dust, is a variety of solid or liquid particles that are uniformly dispersed in an aerosol system. PM can be divided into primary particulate matter and secondary particulate matter. Primary particulate matter is particulate matter that is released into the atmosphere from a direct source of pollution, such as combustion soot, automobile exhaust, etc. Secondary particles are formed by some polluting gas components in the atmosphere, or these components are formed by photochemical oxidation reactions with normal components in the atmosphere. According to its aerodynamic diameter, it can be mainly divided into three types (PM0.1, with a diameter of < 0.1μm; PM 2.5, diameter< 2.5 μm; PM10 has a diameter of <10 μm) and due to its small size and toxicity, it is easy to cause diseases, especially respiratory diseases (147, 148). PM can significantly alter epithelial barrier structure. Studies have shown that PM may disrupt the integrity of the airway epithelial barrier by degrading TJ proteins such as occludin, claudin-1, and ZO-1 (96–99). In addition, in mouse models, exposure to PM2.5 inhibits E-cadherin levels in lung tissue, increasing IFN-γ, IL-2, IL-4, IL-6, and IL-10 in bronchoalveolar lavage fluid (100). Likewise, at lower combustion temperatures, aromatic compounds chemically adhere to the surface of metal oxide-containing PMs, resulting in the formation of surface-stable environmentally persistent free radicals (EPFRs). The reactive oxygen species (ROS) are produced by the EPFR redox cycle containing PM, and the antioxidant and inflammatory responses triggered by ROS after inhalation are closely related to the degradation of claudin-1 and occludin proteins in the airway epithelium and mouse lung tissues induced by PM (97, 101). Likewise, at lower combustion temperatures, aromatic compounds chemically adhere to the surface of metal oxide-containing PMs, resulting in the formation of surface-stable environmentally persistent free radicals (EPFRs). The ROS are produced by the EPFR redox cycle containing PM, and the antioxidant and inflammatory responses triggered by ROS after inhalation are closely related to the degradation of claudin-1 and occludin proteins in the airway epithelium and mouse lung tissues induced by PM (102). Furthermore, PM2.5 and PM0.1 can cause increased lysosomal membrane permeability, oxidative stress and lipid peroxidation at low doses, and epithelial cell necrosis at high doses (103). Recent studies have shown that PM can lead to DNA damage, protein carbonation, and loss of cytokeratin and filaggrin in epidermal structural proteins (104–106).
3.1.2 Nanoparticles
Nanoparticles (NPs), also known as ultrafine particles, typically range in size between 1 and 100 nm. Over the past few decades, the prevalence of nanoparticles in environmental pollution has been steadily increasing, contributing significantly to air pollution. Common nanoparticles include titanium dioxide (TiO2) and silicon dioxide (SiO2), frequently utilized as additives in various products such as food, cosmetics, paints, and catalysts. Research has demonstrated that nanoparticles exhibit a high affinity for lipids, leading to the encapsulation and disruption of phospholipid membranes. This disruptive action extends to various lipid-rich environments, including pulmonary surfactants and endothelial cell junctions in lung blood vessels. Additionally, nanoparticles have been shown to compromise the stability of lysosomal membranes, triggering cell death (107). Specifically, TiO2-NP has been implicated in enhancing the synthesis of interleukin-1a (IL-1a) by inducing an unbalanced overexpression of immature neurotrophic factors. This process, mediated through p75NTR signaling, is associated with apoptosis of epithelial cells (108). Needle-like TiO2-NP has also been found to stimulate the release of pro-inflammatory cytokines such as IL-1a, IL-1b, IL-6, TNF-a, and IL-8, disrupting cellular connections and compromising the skin barrier (109). Moreover, nanoparticles tend to accumulate on the surface of intestinal epithelial cells, M cells, and Peyer patches. Through endocytosis by M cells or increased cell membrane integrity, nanoparticles can disrupt the epithelial barrier, leading to cell leakage and increased intestinal epithelial permeability (110). Similarly, NPs have been observed to raise human intestinal epithelial paracellular permeability (111).
3.1.3 Ozone
Ozone, an oxygen allotrope (O3), exhibits robust oxidizing properties and finds applications as a bleach, air purifier, and disinfectant. It serves as an alternative to catalytic oxidants in chemical production and is utilized as a rocket fuel oxidizer in its liquid form. Despite its beneficial uses, ozone poses a threat to human health as a harmful air pollutant. Recent studies have revealed that prolonged ozone exposure can lead to various respiratory issues, including bronchial hyperresponsiveness, asthma, chronic obstructive pulmonary disease, pulmonary fibrosis, and, in severe cases, death (112). Acute exposure to ozone is known to impair epithelial barrier function, inducing airway inflammation, peribronchial collagen deposition, and airway hyperreactivity (AHR) (113). This acute exposure manifests as asthma symptoms, reduced lung function, increased inflammatory cell infiltration, and heightened AHR (114). Notably, ozone exposure detrimentally affects key molecules involved in epithelial barrier function (149). The inflammatory response induced by ozone in human lungs is reliant on the production of ROS (115), leading to acute epithelial barrier injury and subsequent disruption (116). Upon acute ozone stimulation, cell stress, desquamation, and death occur through reactive ROS. This process is followed by sustained injury and death of bronchiolar epithelial cells, resulting in protein leakage, infiltration of neutrophils and macrophages, and the production of IL-1a and IL-33 by epithelial and myeloid cells (117). Emerging data indicate that, under the control of the IL-33/ST2 axis, ozone directly instigates barrier damage prior to the inflammatory damage mediated by myeloid cells (117).
3.1.4 Tobacco and e-cigarettes
Tobacco stands out as one of the most prevalent toxic substances in the environment, containing approximately 5,000 chemicals notorious for their toxicity to the respiratory system. Research conducted by Burns et al. has elucidated that exposure to cigarette smoke induces an elevation in the permeability of the alveolar epithelial barrier (118). Furthermore, the enduring exposure to tobacco not only leads to diminished gene and protein expression levels of various TJs and AJs proteins but also culminates in the disruption of intercellular junctions (119, 120). In recent years, e-cigarettes have gained prominence, initially marketed as aids for smoking cessation. Despite their advertised potential for assisting in quitting smoking, there is insufficient evidence supporting this claim. E-cigarettes release evaporated nicotine and flavoring, both of which harbor numerous known toxicities capable of causing damage to the epithelial barrier (121).
3.1.5 Enzymes in allergens that disrupt the epithelial barrier
Enzymes present in allergens serve as enhancers of allergic reactions (122). Numerous studies have provided further validation to the notion that the protease activity inherent in allergens, including molds, pollen, cockroaches, house dust mites (HDMs), and certain foods, plays a crucial role in disrupting epithelial integrity and triggering innate immune responses (150). For instance, investigations have demonstrated that mite allergens contribute to the breakdown of TJs by cleaving occludin, leading to intracellular ZO-1 proteolysis and the consequent disruption of the epithelial barrier (123, 124). Matsumura et al. propose that allergen-derived proteases induce TJs disruption among airway epithelial cells, activate protease-activated receptor-2, and generate TSLP, thereby activating the immune response (122). Additionally, Vinhas et al. identified high-molecular-weight proteases with serine and/or aminopeptidase activity in various sensitized pollens, such as Olea europaea, Dactylis glomerata, Cupressus sempervirens, and Pinus sylvestris. These proteases were found to increase Calu-3 transepithelial permeability by disrupting transmembrane adhesion proteins, including occludin, claudin-1, and E-cadherin (125). Aspergillus, known for its abundant production of proteases, induces the release of cytokines like IL-6, IL-8, and MCP-1 in airway epithelial cells. These enzymes also disrupt tight epithelial junctions and prompt cell desquamation (126). Furthermore, Grozdanovic et al. posit that cysteine proteases in food may contribute to the sensitization process of food allergies by disrupting tight junctions (127). In summary, the enzymes present in various active allergens play a pivotal role in disrupting epithelial barrier function, resulting in heightened epithelial barrier permeability. This, in turn, facilitates the invasion of allergens, triggers immune responses, and contributes to the development of allergic diseases.
3.1.6 Micro and nanoplastics
Microplastics (MPs) are water-insoluble polymer particles with a size of less than 5 mm, while nanoplastic particles have a diameter ranging from 1 nm to 1 μm. Recognized as emerging international pollutants due to their small particle size, microplastics are derived from petroleum and are extensively utilized in various aspects of daily life, presenting potential risks to human health (151). The minute dimensions of micro and nanoplastics facilitate their penetration into tissues, allowing interactions with cells and cellular structural molecules (151). Prolonged exposure to these particles has been associated with various degrees of damage to human health (152). Research by Hollóczki et al. has demonstrated the propensity of nanoplastics to interact with proteins, fundamentally altering the secondary structure of these biological macromolecules critical to their function (128). Molecular dynamics simulations have revealed that polyethylene nanoparticles dissolve into an incoherent single polymer chain network within the hydrophobic core of the lipid bilayer, inducing structural and dynamic changes that impact the cell membrane (129). Recent studies evaluating the effects of polystyrene nanoparticles, with diameters of 25 nm and 70 nm, on human alveolar epithelial A549 cell lines indicated that the toxicological effects of PS-NPs on alveolar epithelial cells were dependent on exposure time, diameter, and concentration (130). Jin et al. further observed that polystyrene MPs could induce dysbiosis in intestinal microbiota, leading to intestinal barrier dysfunction and metabolic disorders in mice (131). Additionally, compared with spherical fluorescent polystyrene (PS) particles of different sizes, particles with a diameter of less than 1.5 nm could directly penetrate the gastrointestinal barrier, causing impaired intestinal function (132). Similarly, nanomaterials have been linked to oxidative stress, inducing autophagy in human lung epithelial cells (133). Amidst rapid development, the escalating use of microplastics and nanoplastics raises serious concerns regarding environmental pollution, with concomitant hidden health hazards for human beings.
3.2 Industrial products and epithelial barriers
3.2.1 Detergents
The rise of modernization and industrialization has reinforced people’s emphasis on hygiene, particularly highlighted during the outbreak of the new coronavirus pneumonia epidemic. This attention to hygiene has led to widespread use of detergents and sanitizing products. However, excessive contact and utilization of these cleaning agents can contribute to various diseases, especially skin and respiratory conditions. Research indicates that detergents can play a role in triggering the development of allergic diseases by influencing epithelial barrier disorders (20, 153). Even at minimal concentrations, anionic surfactants and detergents have been shown to directly compromise the integrity of cutaneous keratinocytes and bronchial epithelial barriers by disrupting TJs and associated molecules (63, 134). Long-term use of cleansers, as demonstrated by Douwes et al., can elevate transepidermal water loss (TEWL) in the stratum corneum of the skin, subsequently increasing the risk of contact dermatitis (135). The heightened risk of airway inflammation is linked to the interaction of airway epithelium with environmental air pollutants. Residues of detergents on recently cleaned clothing and floor surfaces can be easily inhaled into the airways, impairing airway barrier function and bronchial epithelial cells. Studies have revealed that emulsifiers can thicken mucosal surface fluid, entrap commensal bacteria, disrupt healthy interactions between epithelial cells and commensal bacteria, alter the microbiota, and interfere with mucosa-bacterial interactions, thereby inducing intestinal inflammation (136). Current research is ongoing to determine whether detergent residues from cleaned dishes can damage the esophageal or gastrointestinal epithelial barrier. Identification of such effects would necessitate the implementation of appropriate avoidance measures (20).
3.3 Pathogen and epithelial barriers
3.3.1 Bacteria
In the pathological mechanisms of epithelial barrier dysfunction, dysbiosis of the microbiota has been reported (5, 154). Among them, bacteria were significantly associated with epithelial barrier dysfunction. Bacterium infections mainly rely on its ability to disrupt the epithelial barrier by affecting cell-cell junctions and altering the expression of TJ and AJ proteins. For example, Staphylococcus aureus colonization and release of enterotoxin B (SEB) can reduce epithelial cell integrity and increase mucosal permeability by decreasing the expression of occlusin and the expression of ZO-1 protein during ALI culture of polyp epithelial cells (137). Similarly, the addition of purified Staphylococcus aureus V8 protease to ALI-cultured human nasal epithelial cells (HNECs) also found the same barrier integrity impairment and discontinuous expression of ZO-1 (155). Studies also have shown that Staphylococcus aureus secreted α-hemolysin interacts with the metalloproteinase domain-containing protein 10 (ADAM10), which can lead to the cleavage of AJ protein E-cadherin and disrupt the lung epithelial barrier in mice (138). Furthermore, Peter et al., when studying human lung tissue with Streptococcus pneumoniae infection, found that pneumococcal infection reduced the levels of alveolar occludin, ZO-1, claudin-5, and VE-cadherin (139). In dextran sulfate sodium (DSS)-induced mouse models of colitis and Caco-2 cell lines, Fusobacterium nucleatum was found to disrupt epithelial integrity and increase permeability by regulating the expression and distribution of tight junction proteins ZO-1 and occulin, causing an aberrant inflammatory response and aggravating colonic inflammation (140). Besides, bacteria can also cause dysfunction of the epithelial barrier through other pathways. Studies have shown that Pseudomonas aeruginosa mediates the disruption of the epithelial barrier by using four effectors: protein exosomes (Exo) S, ExoT, ExoU and ExoY (141). For example, ExoS and ExoT disrupt the host actin cytoskeleton and induce barrier disruption by impairing cell-to-cell adhesion, where ExoY disrupts barrier integrity without cytotoxicity, while another effector protein, ExoU, produces rapid necrotic cytotoxicity (141). In the human body, beneficial commensal bacteria are able to protect and maintain the homeostatic balance of the microenvironment in the body, and the destruction of the epithelial barrier function by pathogenic bacteria leads to an increase in its permeability, which not only enables pathogenic bacteria to reach the subepithelial tissue, but also promotes the secondary invasion of other allergens and pathogens.
3.3.2 Viruses
Viruses are a non-cellular organism that is tiny, simple in structure, contains only one nucleic acid (DNA or RNA), and must parasitize and replicate in living cells. Some studies have found that epithelial dysfunction has a certain correlation with the invasion of viruses. Sajjan et al. found the loss of ZO-1 in the tight junction complex of rhinovirus (RV)-infected cells in a mouse model, and pointed out that RV promotes bacterial binding and translocation by disrupting airway epithelial barrier function, leading to disease occurrence (142). It is worth noting that RV can stimulate the production of ROS and destroy the epithelial barrier, and can also disrupt the epithelial barrier function through dsRNA produced during RV replication (143). In addition, The E protein of SARS coronaviruses and novel coronaviruses interacts with PALS1, a tight junction-related protein, and alter tight junction formation and epithelial morphology, which is manifested by the loss of PALS1 leading to the destruction of TJs and AJs (144, 145). Similarly, Smallcombe et al. found that the molecular composition of TJs in the Respiratory syncytial virus (RSV)-infected airways was altered, as evidenced by significant upregulation of claudin-2 expression and downregulation of ZO-1, occludin, and claudin-1 protein expression, suggesting that increased claudin-2 expression contributes to airway epithelial barrier leakage (146). Different viruses have different mechanisms of epithelial barrier damage, but all of them can increase epithelial barrier permeability and lead to disease infection. Therefore, strategies to maintain barrier function have great potential in the development of antiviral drugs.
4 Evaluation and restoration of epithelial barriers
4.1 Evaluation of epithelial barrier function
Currently, various methods are employed to assess epithelial barrier function, encompassing the measurement of biomarkers, epithelial barrier permeability assays, tissue biopsy, and epithelial cytology, as detailed in Table 2. Transepidermal water loss (TEWL) has emerged as a widely utilized tool for evaluating epithelial barrier dysfunction (156). This process involves a sensor making contact with the skin surface to measure the amount of moisture evaporating from the skin. TEWL measurements, when used in conjunction with stratum corneum stripping (STS), offer insights into the integrity of the skin barrier (43). Tissue biopsy and specific analyses of epithelial cytology serve as additional approaches to assess cell junction structures and associated protein status (156). Ongoing research explores innovative methods for analyzing the skin barrier, including minimally invasive and scarless STS analysis combined with proteomics (43). Mass spectrometry-based protein analysis has revealed significantly lower expression levels of proteins linked to the skin barrier (filaggrin-2, corneodesmosin, desmoglein-1, desmocollin-1, and transglutaminase-3) and natural moisturizing factors (arginase-1, caspase-14, and gamma-glutamyl cyclotransferase) at lesion sites in patients with atopic dermatitis, both with and without a history of herpes eczema (157). Evaluation of gut barrier permeability employs various chemicals, such as sugar, polyethylene glycol, and 51Cr-EDTA, directly assessing barrier function (158). Indirect assessments of mucosal integrity through potential blood biomarkers are also employed. Clara cell protein (CC16) serves as a potential biomarker for airway epithelial injury, with increased serum CC16 observed in acute or chronic lung disorders characterized by elevated airways permeability (159). Strategies for indirect mucosal integrity assessment involve detecting molecules present in the blood, normally found in the intestinal lumen (e.g., LPS), or identifying elevated levels of proteins constituting the intestinal barrier (intestinal fatty-acid binding protein (I-FABP) or tight-junction molecules). These markers indicate intestinal wall damage or elevated concentrations of signaling barrier regulators, such as zonulin, in the blood (158). Moreover, the gold standard for evaluating epithelial barrier function at present involves an assessment combining electrophysiological measurements and probes of varying molecular sizes (158). Rinaldi et al. conducted direct in vivo assessments of epidermal barrier function using electrical impedance (EI) spectroscopy, emphasizing the rapid and reliable diagnostic capabilities of electrical impedance spectroscopy in detecting skin barrier defects (160).
4.2 Epithelial based interventions for allergic diseases therapy
Presently, restoring epithelial barrier function is recognized as a novel strategy for the treatment and prevention of allergic diseases. Notably, Hagner et al. discovered reduced adrenomedullin expression in airway epithelial cells of asthma patients, and supplementation with adrenomedullin promoted the repair of airway epithelial damage (161). Pim1 kinase activity has been identified as crucial for maintaining airway epithelial integrity and preventing pro-inflammatory cytokine secretion induced by HDMs (162). Histone deacetylase (HDAC) activity is implicated in allergic inflammation and tight junction dysfunction between epithelial cells. Increased HDAC activity is an underlying mechanism leading to dysregulation of epithelial cell repair. Inhibition of HDAC activity emerges as a potential strategy to restore nasal epithelium integrity, offering a new avenue for allergic rhinitis therapy (163). Moreover, treatments for allergic diseases play a role in repairing epithelial barrier function. Yuan et al. reported that allergen-specific immunotherapy (SIT) reduced airway inflammatory infiltration and hyperresponsiveness in allergic mice, restoring airway epithelial integrity. This treatment also attenuated Der f-induced airway epithelial endoplasmic reticulum (ER) stress and epithelial apoptosis. Importantly, 4-PBA, an ER stress inhibitor, was found to inhibit IL-25-induced apoptosis of airway epithelial cells dependent on PERK activity (164). Research on intestinal and cutaneous epithelial barrier repair has gained attention. Sun et al. demonstrated that AMP-activated protein kinase (AMPK) enhanced intestinal barrier function and epithelial differentiation by promoting the expression of CDX2 (165). Additionally, He et al. highlighted that different concentrations of vitamin A weakened LPS-induced intestinal epithelial permeability, enhanced the expression of tight junction proteins, and improved intestinal barrier function (166). Various approaches exist to prevent and restore skin barrier integrity, encompassing the use of emollients, moisturizers, occlusive agents, environmental control, and more. Emollients have been reported to reduce the incidence of AD by approximately 50%, exhibiting a protective effect on the skin barrier (167, 168). Emollients and moisturizers in AD serve to protect the skin, form a physical barrier, and retain moisture. Environmental factors, such as allergens, temperature and humidity, skin irritants, and PM2.5, can contribute to skin barrier damage. Effective prevention of skin damage involves controlling these environmental factors. Studies indicate that increased colonization of Staphylococcus aureus on the skin in AD is linked to the loss of commensal bacteria (169). This implies that skin barrier repair can be achieved through microbiota regulation. Current research suggests that human-derived bacteria can reduce Staphylococcus aureus colonization in AD, pointing to the potential of microbiome balance regulation as a therapeutic and preventive approach for allergic diseases (43).
5 Conclusion
The epithelial barrier hypothesis has emerged as a promising avenue for disease prevention and treatment. In the context of contemporary developments in modernization, industrialization, and commercialization, various external risk factors, such as environmental pollution, global warming, widespread detergent use, and biodiversity changes, continually stimulate the epithelial barriers in the skin, respiratory tract, and intestines. This persistent stimulation compromises the integrity of the epithelial barrier, leading to dysbiosis, microbiota translocation in interepithelial and subepithelial regions, and the development of tissue microinflammation. Impaired epithelial barrier function increases permeability, elevating the risk of diverse diseases, especially allergic conditions.
The physical barrier of the epithelial barrier acts as a defense against stimuli like allergens and pathogenic bacteria. Breaches in this physical barrier prompt epithelial cells to release alarm signals (IL-25, IL-33, and TSLP) and activate antigen-presenting cells like dendritic cells. Subsequently, ILC2s cells and Th2 cells are activated, initiating a type 2 immune response. Conversely, the secretion of type 2 immune factors can influence epithelial cells, exacerbating or repairing epithelial barrier dysfunction. Consequently, strategies aimed at treating and restoring epithelial barrier function can be integrated into allergic disease treatment approaches, contributing to disease prevention and management. This review highlights several measures to prevent epithelial barrier dysfunction and address allergic diseases: (1) Control of Environmental Factors: Minimize or avoid exposure to risk factors that harm the epithelial barrier. (2) Development and Use of Safe Products: Adopt safe cleaning products, emollients, and other related items. (3) Mastery of Epithelial Barrier Assessment: Detect epithelial barrier damage promptly through effective assessment methods for preventive purposes. (4) Strengthening the Mucosal Barrier: Prevent bacterial translocation, block colonization by opportunistic pathogens, and reinforce the mucosal barrier. Understanding the role of the epithelial barrier in the mechanisms of allergic diseases can pave the way for innovative preventive and therapeutic methods in future research.
Author contributions
HL: Data curation, Formal analysis, Writing – original draft. YZ: Data curation, Formal analysis, Writing – original draft. LY: Writing – review & editing. QZ: Writing – review & editing. XW: Writing – review & editing. SQ: Funding acquisition, Supervision, Writing – review & editing. BC: Funding acquisition, Methodology, Project administration, Supervision, Writing – review & editing. XZ: Funding acquisition, Project administration, Supervision, Writing – review & editing.
Funding
The author(s) declare financial support was received for the research, authorship, and/or publication of this article. This work was supported by grants Natural Science Foundation of China (No. 81973915 and 81773978, 82004046 and 81773978), Guangdong Basic and Applied Basic Research Foundation (2023A1515012207), Shenzhen Innovation of Science and Technology Commission (No. JCYJ20200109144625016 and JCYJ20220531091602005), Longgang District Science and Technology Plan (No. LGKCYLWS2022010, LGKCYLWS2020046, LGKCYLWS2022003 and LWGJ2022-110), and Shenzhen Key Medical Discipline Construction Fund (No. SZXK039).
Conflict of interest
The authors declare that the research was conducted in the absence of any commercial or financial relationships that could be construed as a potential conflict of interest.
Publisher’s note
All claims expressed in this article are solely those of the authors and do not necessarily represent those of their affiliated organizations, or those of the publisher, the editors and the reviewers. Any product that may be evaluated in this article, or claim that may be made by its manufacturer, is not guaranteed or endorsed by the publisher.
References
1. Wang J, Zhou Y, Zhang H, Hu L, Liu J, Wang L, et al. Pathogenesis of allergic diseases and implications for therapeutic interventions. Signal Transduct Target Ther (2023) 8(1):138. doi: 10.1038/s41392-023-01344-4
2. Celebi SZ, Ozdel OB, Cerci P, Turk M, Gorgulu AB, Akdis M, et al. Epithelial barrier hypothesis: effect of the external exposome on the microbiome and epithelial barriers in allergic disease. Allergy (2022) 77(5):1418–49. doi: 10.1111/all.15240
3. Xing Y, Wong GW. Environmental influences and allergic diseases in the asia-pacific region: what will happen in next 30 years? Allergy Asthma Immunol Res (2022) 14(1):21–39. doi: 10.4168/aair.2022.14.1.21
4. Schleimer RP, Berdnikovs S. Etiology of epithelial barrier dysfunction in patients with type 2 inflammatory diseases. J Allergy Clin Immunol (2017) 139(6):1752–61. doi: 10.1016/j.jaci.2017.04.010
5. Akdis CA. Does the epithelial barrier hypothesis explain the increase in allergy, autoimmunity and other chronic conditions? Nat Rev Immunol (2021) 21(11):739–51. doi: 10.1038/s41577-021-00538-7
6. Hellings PW, Steelant B. Epithelial barriers in allergy and asthma. J Allergy Clin Immunol (2020) 145(6):1499–509. doi: 10.1016/j.jaci.2020.04.010
7. Soyka MB, Wawrzyniak P, Eiwegger T, Holzmann D, Treis A, Wanke K, et al. Defective epithelial barrier in chronic rhinosinusitis: the regulation of tight junctions by ifn-gamma and il-4. J Allergy Clin Immunol (2012) 130(5):1087–96. doi: 10.1016/j.jaci.2012.05.052
8. Wawrzyniak P, Wawrzyniak M, Wanke K, Sokolowska M, Bendelja K, Ruckert B, et al. Regulation of bronchial epithelial barrier integrity by type 2 cytokines and histone deacetylases in asthmatic patients. J Allergy Clin Immunol (2017) 139(1):93–103. doi: 10.1016/j.jaci.2016.03.050
9. Sharma S, Tripathi P. Gut microbiome and type 2 diabetes: where we are and where to go? J Nutr Biochem (2019) 63:101–08. doi: 10.1016/j.jnutbio.2018.10.003
10. Sorini C, Cosorich I, Lo CM, De Giorgi L, Facciotti F, Luciano R, et al. Loss of gut barrier integrity triggers activation of islet-reactive t cells and autoimmune diabetes. Proc Natl Acad Sci U.S.A. (2019) 116(30):15140–49. doi: 10.1073/pnas.1814558116
11. Mouries J, Brescia P, Silvestri A, Spadoni I, Sorribas M, Wiest R, et al. Microbiota-driven gut vascular barrier disruption is a prerequisite for non-alcoholic steatohepatitis development. J Hepatol (2019) 71(6):1216–28. doi: 10.1016/j.jhep.2019.08.005
12. Buscarinu MC, Fornasiero A, Romano S, Ferraldeschi M, Mechelli R, Renie R, et al. The contribution of gut barrier changes to multiple sclerosis pathophysiology. Front Immunol (2019) 10:1916. doi: 10.3389/fimmu.2019.01916
13. Dutta SK, Verma S, Jain V, Surapaneni BK, Vinayek R, Phillips L, et al. Parkinson's disease: the emerging role of gut dysbiosis, antibiotics, probiotics, and fecal microbiota transplantation. J Neurogastroenterol Motil (2019) 25(3):363–76. doi: 10.5056/jnm19044
14. van IJzendoorn S, Derkinderen P. The intestinal barrier in parkinson's disease: current state of knowledge. J Parkinsons Dis (2019) 9(s2):S323–29. doi: 10.3233/JPD-191707
15. Agache I, Miller R, Gern JE, Hellings PW, Jutel M, Muraro A, et al. Emerging concepts and challenges in implementing the exposome paradigm in allergic diseases and asthma: a practall document. Allergy (2019) 74(3):449–63. doi: 10.1111/all.13690
16. Zihni C, Mills C, Matter K, Balda MS. Tight junctions: from simple barriers to multifunctional molecular gates. Nat Rev Mol Cell Biol (2016) 17(9):564–80. doi: 10.1038/nrm.2016.80
17. Ghezzi M, Pozzi E, Abbattista L, Lonoce L, Zuccotti GV, D'Auria E. Barrier impairment and type 2 inflammation in allergic diseases: the pediatric perspective. Children (Basel) (2021) 8(12). doi: 10.3390/children8121165
18. Georas SN, Rezaee F. Epithelial barrier function: at the front line of asthma immunology and allergic airway inflammation. J Allergy Clin Immunol (2014) 134(3):509–20. doi: 10.1016/j.jaci.2014.05.049
19. Godsel LM, Getsios S, Huen AC, Green KJ. The molecular composition and function of desmosomes. Handb Exp Pharmacol (2004) 165):137–93. doi: 10.1007/978-3-540-68170-0_6
20. Celebi SZ, Cevhertas L, Nadeau K, Akdis M, Akdis CA. Environmental factors in epithelial barrier dysfunction. J Allergy Clin Immunol (2020) 145(6):1517–28. doi: 10.1016/j.jaci.2020.04.024
21. Singh N, Diebold Y, Sahu SK, Leonardi A. Epithelial barrier dysfunction in ocular allergy. Allergy (2022) 77(5):1360–72. doi: 10.1111/all.15174
22. Warners MJ, van Rhijn BD, Verheij J, Smout A, Bredenoord AJ. Disease activity in eosinophilic esophagitis is associated with impaired esophageal barrier integrity. Am J Physiol Gastrointest Liver Physiol (2017) 313(3):G230–38. doi: 10.1152/ajpgi.00058.2017
23. Mohapatra A, Van Dyken SJ, Schneider C, Nussbaum JC, Liang HE, Locksley RM. Group 2 innate lymphoid cells utilize the irf4-il-9 module to coordinate epithelial cell maintenance of lung homeostasis. Mucosal Immunol (2016) 9(1):275–86. doi: 10.1038/mi.2015.59
24. Camelo A, Rosignoli G, Ohne Y, Stewart RA, Overed-Sayer C, Sleeman MA, et al. Il-33, il-25, and tslp induce a distinct phenotypic and activation profile in human type 2 innate lymphoid cells. Blood Adv (2017) 1(10):577–89. doi: 10.1182/bloodadvances.2016002352
25. Vivier E, Artis D, Colonna M, Diefenbach A, Di Santo JP, Eberl G, et al. Innate lymphoid cells: 10 years on. Cell (2018) 174(5):1054–66. doi: 10.1016/j.cell.2018.07.017
26. van der Ploeg EK, Carreras MA, Huylebroeck D, Hendriks RW, Stadhouders R. Group 2 innate lymphoid cells in human respiratory disorders. J Innate Immun (2020) 12(1):47–62. doi: 10.1159/000496212
27. Grainge CL, Davies DE. Epithelial injury and repair in airways diseases. Chest (2013) 144(6):1906–12. doi: 10.1378/chest.12-1944
28. Coyne CB, Vanhook MK, Gambling TM, Carson JL, Boucher RC, Johnson LG. Regulation of airway tight junctions by proinflammatory cytokines. Mol Biol Cell (2002) 13(9):3218–34. doi: 10.1091/mbc.e02-03-0134
29. Saatian B, Rezaee F, Desando S, Emo J, Chapman T, Knowlden S, et al. Interleukin-4 and interleukin-13 cause barrier dysfunction in human airway epithelial cells. Tissue Barriers (2013) 1(2):e24333. doi: 10.4161/tisb.24333
30. Papi A, Brightling C, Pedersen SE, Reddel HK. Asthma. Lancet (2018) 391(10122):783–800. doi: 10.1016/S0140-6736(17)33311-1
31. Fahy JV, Dickey BF. Airway mucus function and dysfunction. N Engl J Med (2010) 363(23):2233–47. doi: 10.1056/NEJMra0910061
32. Ordonez CL, Khashayar R, Wong HH, Ferrando R, Wu R, Hyde DM, et al. Mild and moderate asthma is associated with airway goblet cell hyperplasia and abnormalities in mucin gene expression. Am J Respir Crit Care Med (2001) 163(2):517–23. doi: 10.1164/ajrccm.163.2.2004039
33. Frey A, Lunding LP, Ehlers JC, Weckmann M, Zissler UM, Wegmann M. More than just a barrier: the immune functions of the airway epithelium in asthma pathogenesis. Front Immunol (2020) 11:761. doi: 10.3389/fimmu.2020.00761
34. de Boer WI, Sharma HS, Baelemans SM, Hoogsteden HC, Lambrecht BN, Braunstahl GJ. Altered expression of epithelial junctional proteins in atopic asthma: possible role in inflammation. Can J Physiol Pharmacol (2008) 86(3):105–12. doi: 10.1139/y08-004
35. Celebi SZ, Ozbey YU, Altiner S, Ozdel OB, Cerci P, Turk M, et al. The external exposome and allergies: from the perspective of the epithelial barrier hypothesis. Front Allergy (2022) 3:887672. doi: 10.3389/falgy.2022.887672
36. Gon Y, Hashimoto S. Role of airway epithelial barrier dysfunction in pathogenesis of asthma. Allergol Int (2018) 67(1):12–7. doi: 10.1016/j.alit.2017.08.011
37. Nur HS, Tan HT, Md SN, Mohd AN, Wong KK. Nasal epithelial barrier integrity and tight junctions disruption in allergic rhinitis: overview and pathogenic insights. Front Immunol (2021) 12:663626. doi: 10.3389/fimmu.2021.663626
38. Zhang R, Zhang L, Li P, Pang K, Liu H, Tian L. Epithelial barrier in the nasal mucosa, related risk factors and diseases. Int Arch Allergy Immunol (2023) 184(5):481–501. doi: 10.1159/000528969
39. Lee HJ, Kim B, Im NR, Lee DY, Kim HK, Lee SH, et al. Decreased expression of e-cadherin and zo-1 in the nasal mucosa of patients with allergic rhinitis: altered regulation of e-cadherin by il-4, il-5, and tnf-alpha. Am J Rhinol Allergy (2016) 30(3):173–78. doi: 10.2500/ajra.2016.30.4295
40. Wang MJ, Kang MX, Huang MZ, Shen ML, Luo MQ, Li MM, et al. Protease-activated receptor-2 decreased zonula occlidens-1 and claudin-1 expression and induced epithelial barrier dysfunction in allergic rhinitis. Am J Rhinol Allergy (2021) 35(1):26–35. doi: 10.1177/1945892420932486
41. Steelant B, Farre R, Wawrzyniak P, Belmans J, Dekimpe E, Vanheel H, et al. Impaired barrier function in patients with house dust mite-induced allergic rhinitis is accompanied by decreased occludin and zonula occludens-1 expression. J Allergy Clin Immunol (2016) 137(4):1043–53. doi: 10.1016/j.jaci.2015.10.050
42. Leung DY, Guttman-Yassky E. Deciphering the complexities of atopic dermatitis: shifting paradigms in treatment approaches. J Allergy Clin Immunol (2014) 134(4):769–79. doi: 10.1016/j.jaci.2014.08.008
43. Goleva E, Berdyshev E, Leung DY. Epithelial barrier repair and prevention of allergy. J Clin Invest (2019) 129(4):1463–74. doi: 10.1172/JCI124608
44. Leung DY. New insights into atopic dermatitis: role of skin barrier and immune dysregulation. Allergol Int (2013) 62(2):151–61. doi: 10.2332/allergolint.13-RAI-0564
45. Stefanovic N, Irvine AD, Flohr C. The role of the environment and exposome in atopic dermatitis. Curr Treat Options Allergy (2021) 8(3):222–41. doi: 10.1007/s40521-021-00289-9
46. Strid J, Hourihane J, Kimber I, Callard R, Strobel S. Disruption of the stratum corneum allows potent epicutaneous immunization with protein antigens resulting in a dominant systemic th2 response. Eur J Immunol (2004) 34(8):2100–09. doi: 10.1002/eji.200425196
47. Kezic S, O'Regan GM, Yau N, Sandilands A, Chen H, Campbell LE, et al. Levels of filaggrin degradation products are influenced by both filaggrin genotype and atopic dermatitis severity. Allergy (2011) 66(7):934–40. doi: 10.1111/j.1398-9995.2010.02540.x
48. Kezic S, Kemperman PM, Koster ES, de Jongh CM, Thio HB, Campbell LE, et al. Loss-of-function mutations in the filaggrin gene lead to reduced level of natural moisturizing factor in the stratum corneum. J Invest Dermatol (2008) 128(8):2117–19. doi: 10.1038/jid.2008.29
49. Irvine AD, McLean WH, Leung DY. Filaggrin mutations associated with skin and allergic diseases. N Engl J Med (2011) 365(14):1315–27. doi: 10.1056/NEJMra1011040
50. Howell MD, Kim BE, Gao P, Grant AV, Boguniewicz M, Debenedetto A, et al. Cytokine modulation of atopic dermatitis filaggrin skin expression. J Allergy Clin Immunol (2007) 120(1):150–55. doi: 10.1016/j.jaci.2007.04.031
51. Leung D, Berdyshev E, Goleva E. Cutaneous barrier dysfunction in allergic diseases. J Allergy Clin Immunol (2020) 145(6):1485–97. doi: 10.1016/j.jaci.2020.02.021
52. Brough HA, Nadeau KC, Sindher SB, Alkotob SS, Chan S, Bahnson HT, et al. Epicutaneous sensitization in the development of food allergy: what is the evidence and how can this be prevented? Allergy (2020) 75(9):2185–205. doi: 10.1111/all.14304
53. Kezic S, O'Regan GM, Lutter R, Jakasa I, Koster ES, Saunders S, et al. Filaggrin loss-of-function mutations are associated with enhanced expression of il-1 cytokines in the stratum corneum of patients with atopic dermatitis and in a murine model of filaggrin deficiency. J Allergy Clin Immunol (2012) 129(4):1031–39. doi: 10.1016/j.jaci.2011.12.989
54. Wollenberg A, Bieber T. Proactive therapy of atopic dermatitis–an emerging concept. Allergy (2009) 64(2):276–78. doi: 10.1111/j.1398-9995.2008.01803.x
55. Jungersted JM, Scheer H, Mempel M, Baurecht H, Cifuentes L, Hogh JK, et al. Stratum corneum lipids, skin barrier function and filaggrin mutations in patients with atopic eczema. Allergy (2010) 65(7):911–18. doi: 10.1111/j.1398-9995.2010.02326.x
56. Oji V, Eckl KM, Aufenvenne K, Natebus M, Tarinski T, Ackermann K, et al. Loss of corneodesmosin leads to severe skin barrier defect, pruritus, and atopy: unraveling the peeling skin disease. Am J Hum Genet (2010) 87(2):274–81. doi: 10.1016/j.ajhg.2010.07.005
57. Marenholz I, Esparza-Gordillo J, Lee YA. The genetics of the skin barrier in eczema and other allergic disorders. Curr Opin Allergy Clin Immunol (2015) 15(5):426–34. doi: 10.1097/ACI.0000000000000194
58. Kim BE, Leung DY, Boguniewicz M, Howell MD. Loricrin and involucrin expression is down-regulated by th2 cytokines through stat-6. Clin Immunol (2008) 126(3):332–37. doi: 10.1016/j.clim.2007.11.006
59. Sugita K, Akdis CA. Recent developments and advances in atopic dermatitis and food allergy. Allergol Int (2020) 69(2):204–14. doi: 10.1016/j.alit.2019.08.013
60. Lowe AJ, Leung D, Tang M, Su JC, Allen KJ. The skin as a target for prevention of the atopic march. Ann Allergy Asthma Immunol (2018) 120(2):145–51. doi: 10.1016/j.anai.2017.11.023
61. Danso MO, van Drongelen V, Mulder A, van Esch J, Scott H, van Smeden J, et al. Tnf-alpha and th2 cytokines induce atopic dermatitis-like features on epidermal differentiation proteins and stratum corneum lipids in human skin equivalents. J Invest Dermatol (2014) 134(7):1941–50. doi: 10.1038/jid.2014.83
62. Berdyshev E, Goleva E, Bronova I, Dyjack N, Rios C, Jung J, et al. Lipid abnormalities in atopic skin are driven by type 2 cytokines. JCI Insight (2018) 3(4). doi: 10.1172/jci.insight.98006
63. Xian M, Wawrzyniak P, Ruckert B, Duan S, Meng Y, Sokolowska M, et al. Anionic surfactants and commercial detergents decrease tight junction barrier integrity in human keratinocytes. J Allergy Clin Immunol (2016) 138(3):890–93. doi: 10.1016/j.jaci.2016.07.003
64. Luger T, Amagai M, Dreno B, Dagnelie MA, Liao W, Kabashima K, et al. Atopic dermatitis: role of the skin barrier, environment, microbiome, and therapeutic agents. J Dermatol Sci (2021) 102(3):142–57. doi: 10.1016/j.jdermsci.2021.04.007
65. Bjerre RD, Bandier J, Skov L, Engstrand L, Johansen JD. The role of the skin microbiome in atopic dermatitis: a systematic review. Br J Dermatol (2017) 177(5):1272–78. doi: 10.1111/bjd.15390
66. Kong HH, Oh J, Deming C, Conlan S, Grice EA, Beatson MA, et al. Temporal shifts in the skin microbiome associated with disease flares and treatment in children with atopic dermatitis. Genome Res (2012) 22(5):850–59. doi: 10.1101/gr.131029.111
67. Zhu TH, Zhu TR, Tran KA, Sivamani RK, Shi VY. Epithelial barrier dysfunctions in atopic dermatitis: a skin-gut-lung model linking microbiome alteration and immune dysregulation. Br J Dermatol (2018) 179(3):570–81. doi: 10.1111/bjd.16734
68. Brough HA, Liu AH, Sicherer S, Makinson K, Douiri A, Brown SJ, et al. Atopic dermatitis increases the effect of exposure to peanut antigen in dust on peanut sensitization and likely peanut allergy. J Allergy Clin Immunol (2015) 135(1):164–70. doi: 10.1016/j.jaci.2014.10.007
69. Eiwegger T, Hung L, San DK, O'Mahony L, Upton J. Recent developments and highlights in food allergy. Allergy (2019) 74(12):2355–67. doi: 10.1111/all.14082
70. Sicherer SH, Sampson HA. Food allergy: a review and update on epidemiology, pathogenesis, diagnosis, prevention, and management. J Allergy Clin Immunol (2018) 141(1):41–58. doi: 10.1016/j.jaci.2017.11.003
71. Galand C, Leyva-Castillo JM, Yoon J, Han A, Lee MS, McKenzie A, et al. Il-33 promotes food anaphylaxis in epicutaneously sensitized mice by targeting mast cells. J Allergy Clin Immunol (2016) 138(5):1356–66. doi: 10.1016/j.jaci.2016.03.056
72. Tordesillas L, Berin MC, Sampson HA. Immunology of food allergy. Immunity (2017) 47(1):32–50. doi: 10.1016/j.immuni.2017.07.004
73. De Martinis M, Sirufo MM, Suppa M, Ginaldi L. New perspectives in food allergy. Int J Mol Sci (2020) 21(4). doi: 10.3390/ijms21041474
74. Takiishi T, Fenero C, Camara N. Intestinal barrier and gut microbiota: shaping our immune responses throughout life. Tissue Barriers (2017) 5(4):e1373208. doi: 10.1080/21688370.2017.1373208
75. Groschwitz KR, Hogan SP. Intestinal barrier function: molecular regulation and disease pathogenesis. J Allergy Clin Immunol (2009) 124(1):3–20. doi: 10.1016/j.jaci.2009.05.038
76. Niewiem M, Grzybowska-Chlebowczyk U. Intestinal barrier permeability in allergic diseases. Nutrients (2022) 14(9). doi: 10.3390/nu14091893
77. Turner JR. Intestinal mucosal barrier function in health and disease. Nat Rev Immunol (2009) 9(11):799–809. doi: 10.1038/nri2653
78. Salinas E, Reyes-Pavon D, Cortes-Perez NG, Torres-Maravilla E, Bitzer-Quintero OK, Langella P, et al. Bioactive compounds in food as a current therapeutic approach to maintain a healthy intestinal epithelium. Microorganisms (2021) 9(8). doi: 10.3390/microorganisms9081634
79. Ruff WE, Greiling TM, Kriegel MA. Host-microbiota interactions in immune-mediated diseases. Nat Rev Microbiol (2020) 18(9):521–38. doi: 10.1038/s41579-020-0367-2
80. Huffnagle GB, Dickson RP, Lukacs NW. The respiratory tract microbiome and lung inflammation: a two-way street. Mucosal Immunol (2017) 10(2):299–306. doi: 10.1038/mi.2016.108
81. Fiuza B, Fonseca HF, Meirelles PM, Marques CR, Da ST, Figueiredo CA. Understanding asthma and allergies by the lens of biodiversity and epigenetic changes. Front Immunol (2021) 12:623737. doi: 10.3389/fimmu.2021.623737
82. Lunjani N, Satitsuksanoa P, Lukasik Z, Sokolowska M, Eiwegger T, O'Mahony L. Recent developments and highlights in mechanisms of allergic diseases: microbiome. Allergy (2018) 73(12):2314–27. doi: 10.1111/all.13634
83. Kim JW, Kwok SK, Choe JY, Park SH. Recent advances in our understanding of the link between the intestinal microbiota and systemic lupus erythematosus. Int J Mol Sci (2019) 20(19). doi: 10.3390/ijms20194871
84. Earley ZM, Akhtar S, Green SJ, Naqib A, Khan O, Cannon AR, et al. Burn injury alters the intestinal microbiome and increases gut permeability and bacterial translocation. PloS One (2015) 10(7):e129996. doi: 10.1371/journal.pone.0129996
85. Valverde-Molina J, Garcia-Marcos L. Microbiome and asthma: microbial dysbiosis and the origins, phenotypes, persistence, and severity of asthma. Nutrients (2023) 15(3). doi: 10.3390/nu15030486
86. Tang H, Teo SM, Sly PD, Holt PG, Inouye M. The intersect of genetics, environment, and microbiota in asthma-perspectives and challenges. J Allergy Clin Immunol (2021) 147(3):781–93. doi: 10.1016/j.jaci.2020.08.026
87. Arrieta MC, Stiemsma LT, Dimitriu PA, Thorson L, Russell S, Yurist-Doutsch S, et al. Early infancy microbial and metabolic alterations affect risk of childhood asthma. Sci Transl Med (2015) 7(307):307ra152. doi: 10.1126/scitranslmed.aab2271
88. Lee JJ, Kim SH, Lee MJ, Kim BK, Song WJ, Park HW, et al. Different upper airway microbiome and their functional genes associated with asthma in young adults and elderly individuals. Allergy (2019) 74(4):709–19. doi: 10.1111/all.13608
89. Lal D, Keim P, Delisle J, Barker B, Rank MA, Chia N, et al. Mapping and comparing bacterial microbiota in the sinonasal cavity of healthy, allergic rhinitis, and chronic rhinosinusitis subjects. Int Forum Allergy Rhinol (2017) 7(6):561–69. doi: 10.1002/alr.21934
90. Ruokolainen L, Paalanen L, Karkman A, Laatikainen T, von Hertzen L, Vlasoff T, et al. Significant disparities in allergy prevalence and microbiota between the young people in finnish and Russian karelia. Clin Exp Allergy (2017) 47(5):665–74. doi: 10.1111/cea.12895
91. Paller AS, Kong HH, Seed P, Naik S, Scharschmidt TC, Gallo RL, et al. The microbiome in patients with atopic dermatitis. J Allergy Clin Immunol (2019) 143(1):26–35. doi: 10.1016/j.jaci.2018.11.015
92. Bisgaard H, Li N, Bonnelykke K, Chawes BL, Skov T, Paludan-Muller G, et al. Reduced diversity of the intestinal microbiota during infancy is associated with increased risk of allergic disease at school age. J Allergy Clin Immunol (2011) 128(3):646–52. doi: 10.1016/j.jaci.2011.04.060
93. Savage JH, Lee-Sarwar KA, Sordillo J, Bunyavanich S, Zhou Y, O'Connor G, et al. A prospective microbiome-wide association study of food sensitization and food allergy in early childhood. Allergy (2018) 73(1):145–52. doi: 10.1111/all.13232
94. Azad MB, Konya T, Guttman DS, Field CJ, Sears MR, HayGlass KT, et al. Infant gut microbiota and food sensitization: associations in the first year of life. Clin Exp Allergy (2015) 45(3):632–43. doi: 10.1111/cea.12487
95. Dong X, Ding M, Zhang J, Ogulur I, Pat Y, Akdis M, et al. Involvement and therapeutic implications of airway epithelial barrier dysfunction in type 2 inflammation of asthma. Chin Med J (Engl) (2022) 135(5):519–31. doi: 10.1097/CM9.0000000000001983
96. Caraballo JC, Yshii C, Westphal W, Moninger T, Comellas AP. Ambient particulate matter affects occludin distribution and increases alveolar transepithelial electrical conductance. Respirology (2011) 16(2):340–49. doi: 10.1111/j.1440-1843.2010.01910.x
97. Liu J, Chen X, Dou M, He H, Ju M, Ji S, et al. Particulate matter disrupts airway epithelial barrier via oxidative stress to promote pseudomonas aeruginosa infection. J Thorac Dis (2019) 11(6):2617–27. doi: 10.21037/jtd.2019.05.77
98. Zhao R, Guo Z, Zhang R, Deng C, Xu J, Dong W, et al. Nasal epithelial barrier disruption by particulate matter </=2.5 mum via tight junction protein degradation. J Appl Toxicol (2018) 38(5):678–87. doi: 10.1002/jat.3573
99. Xian M, Ma S, Wang K, Lou H, Wang Y, Zhang L, et al. Particulate matter 2.5 causes deficiency in barrier integrity in human nasal epithelial cells. Allergy Asthma Immunol Res (2020) 12(1):56–71. doi: 10.4168/aair.2020.12.1.56
100. Chuang HC, Ho KF, Cao JJ, Chuang KJ, Ho SS, Feng PH, et al. Effects of non-protein-type amino acids of fine particulate matter on e-cadherin and inflammatory responses in mice. Toxicol Lett (2015) 237(3):174–80. doi: 10.1016/j.toxlet.2015.06.013
101. Harmon AC, Hebert VY, Cormier SA, Subramanian B, Reed JR, Backes WL, et al. Particulate matter containing environmentally persistent free radicals induces ahr-dependent cytokine and reactive oxygen species production in human bronchial epithelial cells. PloS One (2018) 13(10):e205412. doi: 10.1371/journal.pone.0205412
102. Prunicki M, Stell L, Dinakarpandian D, de Planell-Saguer M, Lucas RW, Hammond SK, et al. Exposure to no(2), co, and pm(2.5) is linked to regional dna methylation differences in asthma. Clin Epigenet (2018) 10:2. doi: 10.1186/s13148-017-0433-4
103. Thevenot PT, Saravia J, Jin N, Giaimo JD, Chustz RE, Mahne S, et al. Radical-containing ultrafine particulate matter initiates epithelial-to-mesenchymal transitions in airway epithelial cells. Am J Respir Cell Mol Biol (2013) 48(2):188–97. doi: 10.1165/rcmb.2012-0052OC
104. Abayalath N, Malshani I, Ariyaratne R, Zhao S, Zhong G, Zhang G, et al. Characterization of airborne pahs and metals associated with pm10 fractions collected from an urban area of Sri Lanka and the impact on airway epithelial cells. Chemosphere (2022) 286(Pt 2):131741. doi: 10.1016/j.chemosphere.2021.131741
105. Piao MJ, Ahn MJ, Kang KA, Ryu YS, Hyun YJ, Shilnikova K, et al. Particulate matter 2.5 damages skin cells by inducing oxidative stress, subcellular organelle dysfunction, and apoptosis. Arch Toxicol (2018) 92(6):2077–91. doi: 10.1007/s00204-018-2197-9
106. Pan TL, Wang PW, Aljuffali IA, Huang CT, Lee CW, Fang JY. The impact of urban particulate pollution on skin barrier function and the subsequent drug absorption. J Dermatol Sci (2015) 78(1):51–60. doi: 10.1016/j.jdermsci.2015.01.011
107. Urbancic I, Garvas M, Kokot B, Majaron H, Umek P, Cassidy H, et al. Nanoparticles can wrap epithelial cell membranes and relocate them across the epithelial cell layer. Nano Lett (2018) 18(8):5294–305. doi: 10.1021/acs.nanolett.8b02291
108. Chakraborty S, Castranova V, Perez MK, Piedimonte G. Nanoparticles-induced apoptosis of human airway epithelium is mediated by prongf/p75(ntr) signaling. J Toxicol Environ Health A (2017) 80(1):53–68. doi: 10.1080/15287394.2016.1238329
109. Hiroike M, Sakabe J, Kobayashi M, Shimauchi T, Ito T, Hirakawa S, et al. Acicular, but not globular, titanium dioxide nanoparticles stimulate keratinocytes to produce pro-inflammatory cytokines. J Dermatol (2013) 40(5):357–62. doi: 10.1111/1346-8138.12132
110. Vita AA, Royse EA, Pullen NA. Nanoparticles and danger signals: oral delivery vehicles as potential disruptors of intestinal barrier homeostasis. J Leukoc Biol (2019) 106(1):95–103. doi: 10.1002/JLB.3MIR1118-414RR
111. Abudayyak M, GUzel E, Ozhan G. Cytotoxic, genotoxic, and apoptotic effects of nickel oxide nanoparticles in intestinal epithelial cells. Turk J Pharm Sci (2020) 17(4):446–51. doi: 10.4274/tjps.galenos.2019.76376
112. Kim SY, Kim E, Kim WJ. Health effects of ozone on respiratory diseases. Tuberc Respir Dis (Seoul) (2020) 83(Supple 1):S6–11. doi: 10.4046/trd.2020.0154
113. Sokolowska M, Quesniaux V, Akdis CA, Chung KF, Ryffel B, Togbe D. Acute respiratory barrier disruption by ozone exposure in mice. Front Immunol (2019) 10:2169. doi: 10.3389/fimmu.2019.02169
114. Miller RL, Peden DB. Environmental effects on immune responses in patients with atopy and asthma. J Allergy Clin Immunol (2014) 134(5):1001–08. doi: 10.1016/j.jaci.2014.07.064
115. Voter KZ, Whitin JC, Torres A, Morrow PE, Cox C, Tsai Y, et al. Ozone exposure and the production of reactive oxygen species by bronchoalveolar cells in humans. Inhal Toxicol (2001) 13(6):465–83. doi: 10.1080/08958370151131837
116. Sunil VR, Vayas KN, Massa CB, Gow AJ, Laskin JD, Laskin DL. Ozone-induced injury and oxidative stress in bronchiolar epithelium are associated with altered pulmonary mechanics. Toxicol Sci (2013) 133(2):309–19. doi: 10.1093/toxsci/kft071
117. Michaudel C, Mackowiak C, Maillet I, Fauconnier L, Akdis CA, Sokolowska M, et al. Ozone exposure induces respiratory barrier biphasic injury and inflammation controlled by il-33. J Allergy Clin Immunol (2018) 142(3):942–58. doi: 10.1016/j.jaci.2017.11.044
118. Burns AR, Hosford SP, Dunn LA, Walker DC, Hogg JC. Respiratory epithelial permeability after cigarette smoke exposure in Guinea pigs. J Appl Physiol (1985) (1989) 66(5):2109–16. doi: 10.1152/jappl.1989.66.5.2109
119. Tatsuta M, Kan-O K, Ishii Y, Yamamoto N, Ogawa T, Fukuyama S, et al. Effects of cigarette smoke on barrier function and tight junction proteins in the bronchial epithelium: protective role of cathelicidin ll-37. Respir Res (2019) 20(1):251. doi: 10.1186/s12931-019-1226-4
120. Aghapour M, Raee P, Moghaddam SJ, Hiemstra PS, Heijink IH. Airway epithelial barrier dysfunction in chronic obstructive pulmonary disease: role of cigarette smoke exposure. Am J Respir Cell Mol Biol (2018) 58(2):157–69. doi: 10.1165/rcmb.2017-0200TR
121. Fetterman JL, Weisbrod RM, Feng B, Bastin R, Tuttle ST, Holbrook M, et al. Flavorings in tobacco products induce endothelial cell dysfunction. Arterioscler Thromb Vasc Biol (2018) 38(7):1607–15. doi: 10.1161/ATVBAHA.118.311156
122. Matsumura Y. Role of allergen source-derived proteases in sensitization via airway epithelial cells. J Allergy (Cairo) (2012) 2012:903659. doi: 10.1155/2012/903659
123. Takai T, Ikeda S. Barrier dysfunction caused by environmental proteases in the pathogenesis of allergic diseases. Allergol Int (2011) 60(1):25–35. doi: 10.2332/allergolint.10-RAI-0273
124. Wan H, Winton HL, Soeller C, Taylor GW, Gruenert DC, Thompson PJ, et al. The transmembrane protein occludin of epithelial tight junctions is a functional target for serine peptidases from faecal pellets of dermatophagoides pteronyssinus. Clin Exp Allergy (2001) 31(2):279–94. doi: 10.1046/j.1365-2222.2001.00970.x
125. Vinhas R, Cortes L, Cardoso I, Mendes VM, Manadas B, Todo-Bom A, et al. Pollen proteases compromise the airway epithelial barrier through degradation of transmembrane adhesion proteins and lung bioactive peptides. Allergy (2011) 66(8):1088–98. doi: 10.1111/j.1398-9995.2011.02598.x
126. Chaudhary N, Marr KA. Impact of aspergillus fumigatus in allergic airway diseases. Clin Transl Allergy (2011) 1(1):4. doi: 10.1186/2045-7022-1-4
127. Grozdanovic MM, Cavic M, Nesic A, Andjelkovic U, Akbari P, Smit JJ, et al. Kiwifruit cysteine protease actinidin compromises the intestinal barrier by disrupting tight junctions. Biochim Biophys Acta (2016) 1860(3):516–26. doi: 10.1016/j.bbagen.2015.12.005
128. Holloczki O, Gehrke S. Nanoplastics can change the secondary structure of proteins. Sci Rep (2019) 9(1):16013. doi: 10.1038/s41598-019-52495-w
129. Holloczki O, Gehrke S. Can nanoplastics alter cell membranes? Chemphyschem (2020) 21(1):9–12. doi: 10.1002/cphc.201900481
130. Xu M, Halimu G, Zhang Q, Song Y, Fu X, Li Y, et al. Internalization and toxicity: a preliminary study of effects of nanoplastic particles on human lung epithelial cell. Sci Total Environ (2019) 694:133794. doi: 10.1016/j.scitotenv.2019.133794
131. Jin Y, Lu L, Tu W, Luo T, Fu Z. Impacts of polystyrene microplastic on the gut barrier, microbiota and metabolism of mice. Sci Total Environ (2019) 649:308–17. doi: 10.1016/j.scitotenv.2018.08.353
132. Stock V, Bohmert L, Lisicki E, Block R, Cara-Carmona J, Pack LK, et al. Uptake and effects of orally ingested polystyrene microplastic particles. Vitro vivo. Arch Toxicol (2019) 93(7):1817–33. doi: 10.1007/s00204-019-02478-7
133. Lim SL, Ng CT, Zou L, Lu Y, Chen J, Bay BH, et al. Targeted metabolomics reveals differential biological effects of nanoplastics and nanozno in human lung cells. Nanotoxicology (2019) 13(8):1117–32. doi: 10.1080/17435390.2019.1640913
134. Wang M, Tan G, Eljaszewicz A, Meng Y, Wawrzyniak P, Acharya S, et al. Laundry detergents and detergent residue after rinsing directly disrupt tight junction barrier integrity in human bronchial epithelial cells. J Allergy Clin Immunol (2019) 143(5):1892–903. doi: 10.1016/j.jaci.2018.11.016
135. Douwes J, Slater T, Shanthakumar M, McLean D, Firestone RT, Judd L, et al. Determinants of hand dermatitis, urticaria and loss of skin barrier function in professional cleaners in New Zealand. Int J Occup Environ Health (2017) 23(2):110–19. doi: 10.1080/10773525.2018.1427307
136. Viennois E, Chassaing B. First victim, later aggressor: how the intestinal microbiota drives the pro-inflammatory effects of dietary emulsifiers? Gut Microbes (2018) 9(3):1–04. doi: 10.1080/19490976.2017.1421885
137. Martens K, Seys SF, Alpizar YA, Schrijvers R, Bullens D, Breynaert C, et al. Staphylococcus aureus enterotoxin b disrupts nasal epithelial barrier integrity. Clin Exp Allergy (2021) 51(1):87–98. doi: 10.1111/cea.13760
138. Inoshima I, Inoshima N, Wilke GA, Powers ME, Frank KM, Wang Y, et al. A staphylococcus aureus pore-forming toxin subverts the activity of adam10 to cause lethal infection in mice. Nat Med (2011) 17(10):1310–14. doi: 10.1038/nm.2451
139. Peter A, Fatykhova D, Kershaw O, Gruber AD, Rueckert J, Neudecker J, et al. Localization and pneumococcal alteration of junction proteins in the human alveolar-capillary compartment. Histochem Cell Biol (2017) 147(6):707–19. doi: 10.1007/s00418-017-1551-y
140. Liu H, Hong XL, Sun TT, Huang XW, Wang JL, Xiong H. Fusobacterium nucleatum exacerbates colitis by damaging epithelial barriers and inducing aberrant inflammation. J Dig Dis (2020) 21(7):385–98. doi: 10.1111/1751-2980.12909
141. Gao N, Rezaee F. Airway epithelial cell junctions as targets for pathogens and antimicrobial therapy. Pharmaceutics (2022) 14(12). doi: 10.3390/pharmaceutics14122619
142. Sajjan U, Wang Q, Zhao Y, Gruenert DC, Hershenson MB. Rhinovirus disrupts the barrier function of polarized airway epithelial cells. Am J Respir Crit Care Med (2008) 178(12):1271–81. doi: 10.1164/rccm.200801-136OC
143. Comstock AT, Ganesan S, Chattoraj A, Faris AN, Margolis BL, Hershenson MB, et al. Rhinovirus-induced barrier dysfunction in polarized airway epithelial cells is mediated by nadph oxidase 1. J Virol (2011) 85(13):6795–808. doi: 10.1128/JVI.02074-10
144. Teoh KT, Siu YL, Chan WL, Schluter MA, Liu CJ, Peiris JS, et al. The SARS coronavirus e protein interacts with pals1 and alters tight junction formation and epithelial morphogenesis. Mol Biol Cell (2010) 21(22):3838–52. doi: 10.1091/mbc.E10-04-0338
145. Shepley-McTaggart A, Sagum CA, Oliva I, Rybakovsky E, DiGuilio K, Liang J, et al. SARS-cov-2 envelope (e) protein interacts with pdz-domain-2 of host tight junction protein zo1. PloS One (2021) 16(6):e251955. doi: 10.1371/journal.pone.0251955
146. Smallcombe CC, Linfield DT, Harford TJ, Bokun V, Ivanov AI, Piedimonte G, et al. Disruption of the airway epithelial barrier in a murine model of respiratory syncytial virus infection. Am J Physiol Lung Cell Mol Physiol (2019) 316(2):L358–68. doi: 10.1152/ajplung.00345.2018
147. Yang L, Li C, Tang X. The impact of pm(2.5) on the host defense of respiratory system. Front Cell Dev Biol (2020) 8:91. doi: 10.3389/fcell.2020.00091
148. Wang C, Feng L, Chen K. The impact of ambient particulate matter on hospital outpatient visits for respiratory and circulatory system disease in an urban chinese population. Sci Total Environ (2019) 666:672–79. doi: 10.1016/j.scitotenv.2019.02.256
149. Hollingsworth JW, Kleeberger SR, Foster WM. Ozone and pulmonary innate immunity. Proc Am Thorac Soc (2007) 4(3):240–46. doi: 10.1513/pats.200701-023AW
150. Jacquet A. Interactions of airway epithelium with protease allergens in the allergic response. Clin Exp Allergy (2011) 41(3):305–11. doi: 10.1111/j.1365-2222.2010.03661.x
151. Yee MS, Hii LW, Looi CK, Lim WM, Wong SF, Kok YY, et al. Impact of microplastics and nanoplastics on human health. Nanomaterials (Basel) (2021) 11(2). doi: 10.3390/nano11020496
152. Wright SL, Kelly FJ. Plastic and human health: a micro issue? Environ Sci Technol (2017) 51(12):6634–47. doi: 10.1021/acs.est.7b00423
153. Cecchi L, D'Amato G, Annesi-Maesano I. External exposome and allergic respiratory and skin diseases. J Allergy Clin Immunol (2018) 141(3):846–57. doi: 10.1016/j.jaci.2018.01.016
154. Levy M, Kolodziejczyk AA, Thaiss CA, Elinav E. Dysbiosis and the immune system. Nat Rev Immunol (2017) 17(4):219–32. doi: 10.1038/nri.2017.7
155. Murphy J, Ramezanpour M, Stach N, Dubin G, Psaltis AJ, Wormald PJ, et al. Staphylococcus aureus v8 protease disrupts the integrity of the airway epithelial barrier and impairs il-6 production. vitro. Laryngoscope (2018) 128(1):E8–15. doi: 10.1002/lary.26949
156. Alexander H, Brown S, Danby S, Flohr C. Research techniques made simple: transepidermal water loss measurement as a research tool. J Invest Dermatol (2018) 138(11):2295–300. doi: 10.1016/j.jid.2018.09.001
157. Broccardo CJ, Mahaffey S, Schwarz J, Wruck L, David G, Schlievert PM, et al. Comparative proteomic profiling of patients with atopic dermatitis based on history of eczema herpeticum infection and staphylococcus aureus colonization. J Allergy Clin Immunol (2011) 127(1):186–93. doi: 10.1016/j.jaci.2010.10.033
158. Vanuytsel T, Tack J, Farre R. The role of intestinal permeability in gastrointestinal disorders and current methods of evaluation. Front Nutr (2021) 8:717925. doi: 10.3389/fnut.2021.717925
159. Broeckaert F, Clippe A, Knoops B, Hermans C, Bernard A. Clara cell secretory protein (cc16): features as a peripheral lung biomarker. Ann N Y Acad Sci (2000) 923:68–77. doi: 10.1111/j.1749-6632.2000.tb05520.x
160. Rinaldi AO, Morita H, Wawrzyniak P, Dreher A, Grant S, Svedenhag P, et al. Direct assessment of skin epithelial barrier by electrical impedance spectroscopy. Allergy (2019) 74(10):1934–44. doi: 10.1111/all.13824
161. Hagner S, Welz H, Kicic A, Alrifai M, Marsh LM, Sutanto EN, et al. Suppression of adrenomedullin contributes to vascular leakage and altered epithelial repair during asthma. Allergy (2012) 67(8):998–1006. doi: 10.1111/j.1398-9995.2012.02851.x
162. de Vries M, Hesse L, Jonker MR, van den Berge M, van Oosterhout AJ, Heijink IH, et al. Pim1 kinase activity preserves airway epithelial integrity upon house dust mite exposure. Am J Physiol Lung Cell Mol Physiol (2015) 309(11):L1344–53. doi: 10.1152/ajplung.00043.2015
163. Steelant B, Wawrzyniak P, Martens K, Jonckheere AC, Pugin B, Schrijvers R, et al. Blocking histone deacetylase activity as a novel target for epithelial barrier defects in patients with allergic rhinitis. J Allergy Clin Immunol (2019) 144(5):1242–53. doi: 10.1016/j.jaci.2019.04.027
164. Yuan X, Wang J, Li Y, He X, Niu B, Wu D, et al. Allergy immunotherapy restores airway epithelial barrier dysfunction through suppressing il-25 -induced endoplasmic reticulum stress in asthma. Sci Rep (2018) 8(1):7950. doi: 10.1038/s41598-018-26221-x
165. Sun X, Yang Q, Rogers CJ, Du M, Zhu MJ. Ampk improves gut epithelial differentiation and barrier function via regulating cdx2 expression. Cell Death Differ (2017) 24(5):819–31. doi: 10.1038/cdd.2017.14
166. He C, Deng J, Hu X, Zhou S, Wu J, Xiao D, et al. Vitamin a inhibits the action of lps on the intestinal epithelial barrier function and tight junction proteins. Food Funct (2019) 10(2):1235–42. doi: 10.1039/c8fo01123k
167. Simpson EL, Chalmers JR, Hanifin JM, Thomas KS, Cork MJ, McLean WH, et al. Emollient enhancement of the skin barrier from birth offers effective atopic dermatitis prevention. J Allergy Clin Immunol (2014) 134(4):818–23. doi: 10.1016/j.jaci.2014.08.005
168. Horimukai K, Morita K, Narita M, Kondo M, Kitazawa H, Nozaki M, et al. Application of moisturizer to neonates prevents development of atopic dermatitis. J Allergy Clin Immunol (2014) 134(4):824–30. doi: 10.1016/j.jaci.2014.07.060
Keywords: epithelial barrier, allergic diseases, barrier dysfunction, type 2 inflammation, prevention and treatment
Citation: Lu H-F, Zhou Y-C, Yang L-T, Zhou Q, Wang X-J, Qiu S-Q, Cheng B-H and Zeng X-H (2024) Involvement and repair of epithelial barrier dysfunction in allergic diseases. Front. Immunol. 15:1348272. doi: 10.3389/fimmu.2024.1348272
Received: 02 December 2023; Accepted: 15 January 2024;
Published: 01 February 2024.
Edited by:
Nicola Ivan Lorè, IRCCS San Raffaele Scientific Institute, Milan, ItalyReviewed by:
Wendy Fonseca, University of Michigan, United StatesShradha Wali, McGill University Health Centre, Canada
Copyright © 2024 Lu, Zhou, Yang, Zhou, Wang, Qiu, Cheng and Zeng. This is an open-access article distributed under the terms of the Creative Commons Attribution License (CC BY). The use, distribution or reproduction in other forums is permitted, provided the original author(s) and the copyright owner(s) are credited and that the original publication in this journal is cited, in accordance with accepted academic practice. No use, distribution or reproduction is permitted which does not comply with these terms.
*Correspondence: Xian-Hai Zeng, zxhklwx@163.com; Shu-Qi Qiu, qiuqi66858@163.com; Bao-Hui Cheng, chengbaohui@sina.com
†These authors have contributed equally to this work and share first authorship