Variability in the Net Ecosystem Productivity (NEP) of Seaweed Farms
- 1Bio-Resources Business Development Division, Riken Food Co., Ltd., Tagajyo, Japan
- 2RIKEN Nishina Center for Accelerator-Based Science, Wako, Japan
- 3Organization for Marine Science and Technology, Institute for East China Sea Research, Nagasaki University, Nagasaki, Japan
- 4Graduate School of Fisheries and Environmental Sciences, Nagasaki University, Nagasaki, Japan
- 5Department of Chemistry, Biology and Marine Science, Faculty of Science, University of the Ryukyus, Nishihara, Japan
- 6Kuroshio Biological Research Foundation, Otsuki, Japan
- 7Graduate School of Engineering and Science, University of the Ryukyus, Nishihara, Japan
- 8United Graduate School of Agricultural Sciences, Kagoshima University, Kagoshima, Japan
- 9Faculty of Fisheries, Kagoshima University, Kagoshima, Japan
The important role of vegetated ecosystems in the sequestration of carbon has gained strong interest across a wide variety of disciplines. With evidence growing of the potential for macroalgae ecosystems to capture carbon, there is burgeoning interest in applying newfound knowledge of carbon capture rates to better understand the potential for carbon sequestration. Seaweed farms are expected to play a significant role in carbon capture; advocates for the expansion of seaweed farms are increasing in many countries. In general, seaweed farms are expected to be highly productive, although whether they are autotrophic or heterotrophic ecosystems and hence potential exporters of carbon, is under debate. Therefore, we present our investigation of three seaweed farms, two in northern Japan and one in southern Japan. We examine the frequency of autotrophic days and compare potential rates of carbon capture of the seaweed farms with two natural macroalgae ecosystems and one degraded site. We estimated potential carbon capture rates by calculating the net ecosystem productivity from continuous recordings of dissolved oxygen concentrations under natural environmental conditions. The net ecosystem production rates for the natural ecosystems in Arikawa Bay and Omura Bay were equivalent to 0.043 and 0.054 [g C m-2 d-1] m-1, respectively. Whereas, for the degraded ecosystem in Tainoura Bay, it was -0.01 [g C m-2 d-1] m-1. We reveal that the Undaria pinnatifida farm in Matsushima Bay experience autotrophy more often than natural ecosystems, although for seaweed farms producing U. pinnatifida in Hirota Bay and Cladospihon okamuranus at Bise Point, autotrophy was less frequently observed. Nevertheless, up to 14.1 g C m-2 (0.110 g C m-2 d-1) was captured by the production of U. pinnatifida and 3.6 g C m-2 (0.034 g C m-2 d-1) was captured by C. okamuranus, and the total yield of carbon captured during 2021 production season for these farms was 43,385 kg C.
Introduction
Vegetated ecosystems are expected to play an important role in sequestering carbon. Interest and research in carbon sequestration by vegetated coastal ecosystems are driving the development of blue carbon strategies to mitigate the effects of climate change (McLeod et al., 2011; Siegel et al., 2021). Some evidence suggests that macroalgae ecosystems can capture carbon dioxide from the environment just as effectively as seagrass, mangrove, and salt marsh ecosystems (Hill et al., 2015; Trevathan-Tackett et al., 2015; Ortega et al., 2019; Gouvêa et al., 2020). Hence, it is expected that macroalgae ecosystems, which cover approximately 3.4 million km2 of coastal ocean area, can capture approximately 1.5 Gt C yr-1 (Krause-Jensen and Duarte, 2016). Estimates suggest that approximately 165 Mt C yr-1 may be sequestered for time-scales relevant to the mitigation of climate change (Frontier et al., 2021). Hence, interest in developing seaweed aquaculture as a carbon mitigation tool can be attributed to their global coverage, high rates of productivity, and the perception that there is a high social and economic cost of carbon removal in terrestrial ecosystems (Chung et al., 2011; Boysen et al., 2017).
Approximately 48 million km2 of ocean area is believed to be suitable for seaweed aquaculture and has the potential to create a carbon neutral industry by combining traditional fin-fish aquaculture with seaweed aquaculture (Froehlich et al., 2019). Based on annual landings of seaweed harvests from aquaculture farms, an estimated 0.7 Mt C yr-1 was captured in the Asian-Pacific region (Sondak et al., 2017). The global seaweed aquaculture harvest is expected to capture 680 Mt C yr-1 from the environment (Duarte, 2017).
In contrast to seagrass dominated ecosystems, which are expected to sequester some of the captured carbon locally within the sediment of their habitat, macroalgae dominated ecosystems are less able to sequester carbon locally because most macroalgae occur on rocky substrate. Macroalgae ecosystems are expected to export a large fraction of the carbon that is captured through photosynthesis and serve as a carbon source — captured carbon (i.e., about 11% of macroalgal net C production) is exported to adjacent environments and deposited in sediments for relevant time-scales (Krause-Jensen et al., 2018). Recent studies indicate that the biomass produced by macroalgae may continue to capture carbon through photosynthesis after becoming detached from the substrate or thallus as they are exported out of the local habitat and enhance the deposition of detritus away from rocky habitat and into sediments, which can facilitate carbon sequestration (Krumhansl and Scheibling, 2012; Pedersen et al., 2020; Frontier et al., 2021; Smale et al., 2021). The potential for mitigating greenhouse gases, especially carbon dioxide, with macroalgae ecosystems or macroalgae aquaculture is debatable (Muraoka, 2004; Hill et al., 2015; Fillbee-Dexter and Wernberg, 2020; Gallagher et al., 2021; Gallagher et al., 2022) and their status as climate mitigating carbon sinks requires careful inquiry (Howard et al., 2017). Nevertheless, macroalgae ecosystems and seaweed aquaculture are increasingly touted as a key blue carbon strategy (Krause-Jensen and Duarte, 2016; Duarte and Krause-Jensen, 2017; Duarte et al., 2017; Krause-Jensen et al., 2018; Froehlich et al., 2019; Ortega et al., 2019; Fillbee-Dexter and Wernberg, 2020).
There is little field evidence regarding the amount of carbon removed by seaweed aquaculture farms. Nevertheless, Saccharina japonica farms in the Yellow Sea increased dissolved oxygen concentrations and exhibited a deficit in pCO2 (Xiao et al., 2021). However, a recent study suggests that macroalgae ecosystems are generally heterotrophic and rather than exporting carbon to the deep ocean, these ecosystems are local producers of carbon dioxide due to the influence of allochthonous organic matter sources (Gallagher et al., 2022). Therefore, we explore the potential of seaweed farms in Japan to capture carbon and compare them to natural ecosystems dominated by macroalgae. We infer the potential of seaweed farms and natural ecosystems to capture carbon by estimating their net ecosystem production using continuous recordings of dissolved oxygen, evaluating the proportion of autotrophic days in these ecosystems, and by estimating carbon captured from the total yields of the farms. We expected that the seaweed farms were autotrophic more often than natural ecosystems, since natural ecosystems are expected to be primarily heterotrophic (Gallagher et al., 2022).
Materials and Methods
Study Sites, Period, and Macroalgae Taxa
Our data was collected from two natural seaweed ecosystems, one degraded seaweed ecosystem, and three seaweed farms from six geographic location across Japan (Figure 1). The natural ecosystems that were examined were seaweed beds dominated by a variety of Sargassum taxa. The degraded ecosystem is one that was previously a seaweed abundant ecosystem that has degraded into a state of isoyake (i.e., a state where a seaweed ecosystem degraded into an ecosystem devoid of habitat forming seaweed; Fujita, 2010; Vergés et al., 2014; Eger et al., 2022). Two clusters of seaweed farms were monitored: Undaria pinnatifida farms in the north and a Cladosiphon okamuranus farm in the south (Table 1 and Figure 1).
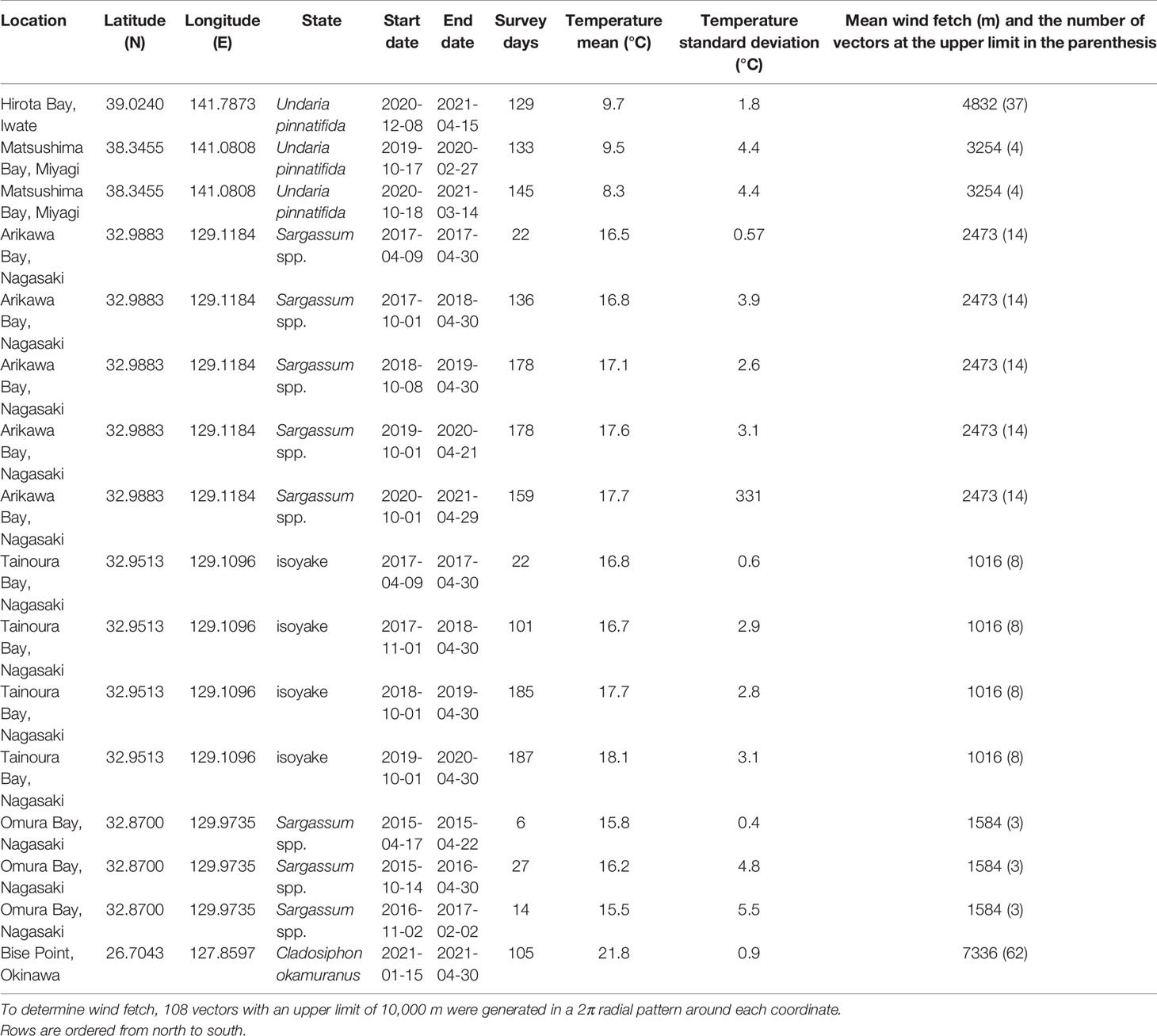
Table 1 Location, state, survey period, the number of recorded days (Survey days), the mean and standard deviation of the temperature during the survey period, and the mean fetch of the study sites.
Matsushima Bay and Hirota Bay are located in northern Japan and are the sites of the U. pinnatifida farms (Table 1 and Figure 1). Both bays have predominantly silty substrates. The seaweed farm at Matsushima Bay is in relatively shallow (ca. 5 m) and enclosed waters, whereas the seaweed farm at Hirota Bay is in deep water with depths ranging from 30 to 40 m. Both bays open to the east, where the mean fetch (number of vectors at the fixed limit) for Hirota Bay was 4832 m (37), whereas the mean fetch for Matsushima Bay was 3254 m (4). Both Matsushima Bay and Hirota Bay experience a similar range in salinity, ranging from 30 to 35 (Ichikawa et al., 2009; Jianxi et al., 2020). Besides U. pinnatifida, commercial cultivation of oysters, Pyropia spp., and Saccharina spp. are present in Matsushima Bay, while scallops are cultivated in Hirota Bay.
Bise Point is located in southern Japan and is the site of the C. okamuranus farm. Here, the farm is located within the lagoon of a coral reef in 3 to 6 m of water and faces the ocean to the east with a mean fetch of 7336 m (62). Cultivation of C. okamuranus occurs in the lagoon to minimize exposure to strong hydrodynamic forces. Corals and seagrasses such as Thalassia hemprichii, Cymodocea rotundata, and Halodule uninvervis can often be observed within the lagoon where salinity ranges from 32 to 35 (Higuchi et al., 2014).
Omura Bay, Arikawa Bay, and Tainoura Bay are located in Nagasaki Prefecture, in western Japan. Omura Bay is a highly enclosed bay, with a narrow and restricted entrance to the northeast. The study site has a mean fetch of 1584 m (3) and is a Sargassum spp. dominated ecosystem. The sediment at the site of Omura Bay is a mix of rock and sand. Salinity ranges from 30 to 35 (Nogami and Matsuno, 2001; Tsuchiya et al., 2018). Arikawa Bay and Tainoura Bay are in Nakadori Island, of the Goto Archipelago. Arikawa Bay is a wide north facing bay and is open to the ocean with a mean fetch of 2473 m (14). Tainoura Bay is a long narrow bay facing east with a mean fetch of 1016 (8) and is the site of an ecosystem degraded into an isoyake state, whereas Arikawa Bay is the site of a Sargassum spp. dominated ecosystem. Both bays have similar substrates, which is a mix of rock and sand.
All the sites examined in our study are located near large municipalities with armored shores. Although no major rivers drain into study sites, small streams and stormwater drains are present along the shore. Based on the mean fetch, we can rank the relative exposure of the sites from highest to lowest: Bise Point, Hirota Bay, Matsushima Bay, Arikawa Bay, Omura Bay, and Tainoura Bay. Data collection for the natural ecosystems occurred throughout the year; however, there are some gaps in the collection period due equipment malfunction and interruptions caused by tropical storms. Also, data collection for the seaweed farms were restricted to the production season (Table 1). The period of seaweed production is variable and occurs for less than 6 months of the year. Variations in the production period also occurs due to the cultivation of different species and the differences in the environmental conditions. Therefore the comparisons made in this study are made with knowledge of this limitation.
Temperature and Dissolved Oxygen
Water temperature and dissolved oxygen concentrations were recorded with dataloggers (U26-001, Onset Computer Corporation, Bourne, MA, USA) at a rate of one sample every ten minutes. The U26-001 is an optical sensor that uses fluorescence to measure dissolved oxygen concentrations. Loggers were calibrated before and after deployment and referenced against a hand-held optical dissolved oxygen sensor (ProODO, Xylem Inc., Yellow Springs, OH, USA). In the natural and degraded ecosystems, one datalogger was placed directly above the sediment, another 50 cm above the sediment, and third 50 cm below the water surface (i.e., a total of three instruments and mean depth of 3 m for Omura Bay, 5 m for Arikawa Bay, and 8 m for Tainoura Bay). The dataloggers at the seaweed aquaculture farms were placed on the cultivation ropes and 1 m (Bise Point) and 2 m (Hirota Bay and Matsushima Bay) below the cultivation ropes (i.e., a total of two instruments). The instruments were placed so that the majority of the biomass of the seaweed were between the data loggers. Recordings were carried out for four to twenty days before the instruments were retrieved for maintenance and data offloading (e.g., Hinode et al., 2020). Calculations of productivity (as described below) is based on the ensemble mean of the dissolved oxygen time-series recorded by the dataloggers, to account for vertical heterogeneity.
Light and Wind Speed
To determine daylength and to calculate the air-sea gas flux of oxygen, the photosynthetic photon flux density (S-LIA-M003, Onset Computer Corporation, Bourne, MA, USA) and wind speed (S-BPB-CM50, Onset Computer Corporation, Bourne, MA, USA) were recorded to a datalogger (USB Microstation, Onset Computer Corporation, Bourne, MA, USA) at a rate of one sample every ten minutes. One set of instruments were deployed at each site. At the natural ecosystems, the measurements were taken one meter above the seawater surface by placing the equipment on a floating raft; however, at the seaweed aquaculture farms, the instruments were placed 10 m above ground at nearby boat harbors. Data was recorded for four to twenty days (e.g., Hinode et al., 2020).
Estimating Productivity
The gross ecosystem production (GEP) rates and ecosystem respiration (ER) rates were estimated from the diurnal fluctuations of the dissolved oxygen concentration with the open-water method (Hanson et al., 2008; Staehr et al., 2010; Champenois and Borges, 2012; Berg et al., 2019; Hinode et al., 2020), therefore the data includes not only the physiological processes of photosynthetic organisms, but also includes the respiration of non-photosynthetic organisms (see Gallagher et al., 2022 for details on the processes occurring in open systems). Briefly, the net ecosystem production (NEP) rate can be defined as NEP = GEP + ER, where GEP are positive values and ER are negative values. NEP rates (NEPt) with respect to sampling time (i.e., Δt = 10 minutes) are calculated as the time rate-of-change of the dissolved oxygen concentrations determined from a smoother fitted with a generalized additive model (GAM) and the calculated flux (FO2,t) at the air-sea interface (Wanninkhof, 1992). The smoother for the GAM was a thin-plate spline, and the distribution was assumed to be Gaussian (Wood, 2011). Physical flux of dissolved oxygen within the water column cannot be evaluated by this method, however given the relative size of our measurement sites, our experience shows that any concentration gradients that could affect the measurements tend to sum to zero. Hence, we define the daily rates of NEP as the sum of the flux-corrected NEP rates (NEP=∑NEPt), and are approximations to NEP. The daily rates of ER are the sum of flux-corrected NEP rates occurring during the night (Hn), which were then normalized to 24 hours . We assume that ER occurring during the night is representative of ER occurring over 24 hours. It is important to note that at the spatial scale of this study, unmeasured processes such as the physical flux of oxygen and the calcium carbonate cycle should be expected to influence GEP estimates, regardless of methodology (Holtgrieve et al., 2010; Staehr et al., 2012; Smith, 2013; Macreadie et al., 2017). Nevertheless, after assuming a 1:1 relationship between O2 production and CO2 consumption (Gauthier et al., 2018), we refer to NEP interchangeably with carbon capture potential, where the conversion rate of O2 to C was 0.375. Units normalized to unit depth, given the vertical position of the data loggers, and are presented in [g m-2 d-1] m-1. It is important to recall that the vertical position of the data loggers was designed to maximize the fidelity of the dissolved oxygen fluctuations caused by photosynthetic and respiratory activity in the study system.
The total yield and carbon content of U. pinnatifida and C. okamuranus was determined for the 2021 production season. The percent carbon content for the seaweeds were determined from 5 haphazardly selected thalli for each taxa collected on 30 April 2021 for C. okamuranus, 14 March 2021 for U. pinnatifida from Matsushima Bay, and 15 April 2021 for U. pinnatifida from Hirota Bay. The whole thalli was analyzed for the C. okamuranus specimens. However, due to the large size of the U. pinnatifida specimens, a 20 mm diameter section of the frond was excised from the widest portion of the frond and used in the carbon content analysis. The seawater on the samples were carefully removed from their surfaces by blotting with a paper towel. The samples were dried in an oven (EYELA WFO-500, Tokyo Rikakikai Co., Ltd., Tokyo, Japan) for 12 hours at 80 °C. The dried samples were pulverized with a mortar and pestle, and the carbon content was measured with a CHN analyzer (Flash 2000, Thermofisher Scientific, Waltham, MA, USA).
Data Analysis
Inclement weather conditions caused periods of missing light and wind data. In the case of missing wind values, wind speeds were interpolated by fitting a generalized additive model to the wind, gust, and barometric pressure measurements publicly available from the Japan Meteorological Agency (https://www.jma.go.jp/). The smoother for the generalized additive model was a tensor smooth of the three variables (i.e., wind speed, gust speed, and barometric pressure) with a normal distribution and an identity link-function. The Matsushima Bay observations referenced the wind and gust speed data from the Shiogama station and the barometric pressure from the Ishinomaki station, the Hirota Bay observations referenced the wind and gust speed data from the Kesen’numa station and the barometric pressure from the Ishinomaki station, the Bise Point observations referenced Nago station, and Arikawa Bay and Tainoura Bay observations referenced the Fukue station. No interpolation was needed for the Omura Bay observations. Interpolating light values resulted in poor estimates; however, the light data was only required to estimate day length, therefore the crepuscule function from the R package maptools was used to calculate day length for each study site (Bivand and Lewkin-Koh, 2021).
The relative wind exposure of each site was estimated by calculating the wind fetch with the fetchR package (Blake, 2020). A total of 108 vectors radiating out from each GPS coordinate (Table 1) were initially generated in a 2π radial pattern (see Supplement for details). The upper limit of the vectors was initialized to 10,000 m. Vectors that reached an obstruction (i.e., shoreline) was truncated and the mean values of these vectors were determined. Vectors that were not truncated (i.e., 10,000 m), were included and the numbers of untruncated vectors recorded. The mean wind fetch and number of untruncated vectors is a proxy for the physical conditions (e.g., wind and wave exposure) of the sites.
A generalized linear model (GLM) was used to analyze the effect of site (i.e., Hirota Bay, Matsushima Bay, Arikawa Bay, Tainoura Bay, Omura Bay, and Bise Point) on the daily NEP rates. A Student’s t-distribution with an identity-link function was applied to the GLM. The variance for each site was modeled separately using a log-link function. The Student’s t-distribution with 3 degrees-of-freedom, a location of 0, and a scale of 2.5 was the prior distribution that was applied to all parameters of the GLM. However, and a Gamma distribution with a shape of 2 and an inverse scale of 0.1 was applied to the degrees-of-freedom parameter for the GLM.
A GLM was also used to analyze the effect of site (i.e., Hirota Bay, Matsushima Bay, Arikawa Bay, Tainoura Bay, Omura Bay, and Bise Point) on the frequency of autotrophic days (i.e., positive rates of net ecosystem production). A Bernoulli distribution with a logit-link function was applied to this model and the prior distributions for the model parameters were similar to those applied in the GEP analysis. We did not include temperature or wind fetch as predictors in either of the GLMs, since they would be confounded with site.
All analyses were done with R version 4.1.2 (R Core Team, 2021) and days with missing values or erroneous measurements were excluded from the analysis. We used the brms package (Bürkner, 2017; Bürkner, 2018) to fit the model and ran four Markov chains for at least 4000 iterations each. The posterior distribution and convergence of the Markov chains were assessed visually. We report the mean and standard deviation of the observed rates of GEP, NEP, and ER. These values are unconditional with respect to the model and hence are not restricted to distributional assumptions. As the data was not normally distributed, our discussion focuses on the conditional means estimated by the GLM, which are conditional on the model and data, and minimize the influence of the outliers. The value of the unconditional and conditional mean may differ in sign and magnitude (especially for values near zero) and likely indicates the effect of the scaled t-distribution of the model on the conditional mean.
Results
The mean temperature observed during the study period across the sites ranged from 8.3 to 21.8°C (Table 1) and was lowest at the northern sites; however, the mean temperatures do not reflect yearly means for the seaweed aquaculture farms, since they were only collected during production season of Undaria pinnatifida and Cladosiphon okamuranus. From the 1726 daily observations, 169 observations were excluded from the analyses if the calculated values of GEP was less than zero or ER was greater than zero. An examination of the daily NEP rates indicated a symmetrical distribution with long tails, hence the use of a scaled t-distribution to model the error term in the GLM. However, the observations for all sites, except for Hirota Bay, (Figure 2) had a light negative skew (i.e., long tails to the left) when compared to the results of the model.
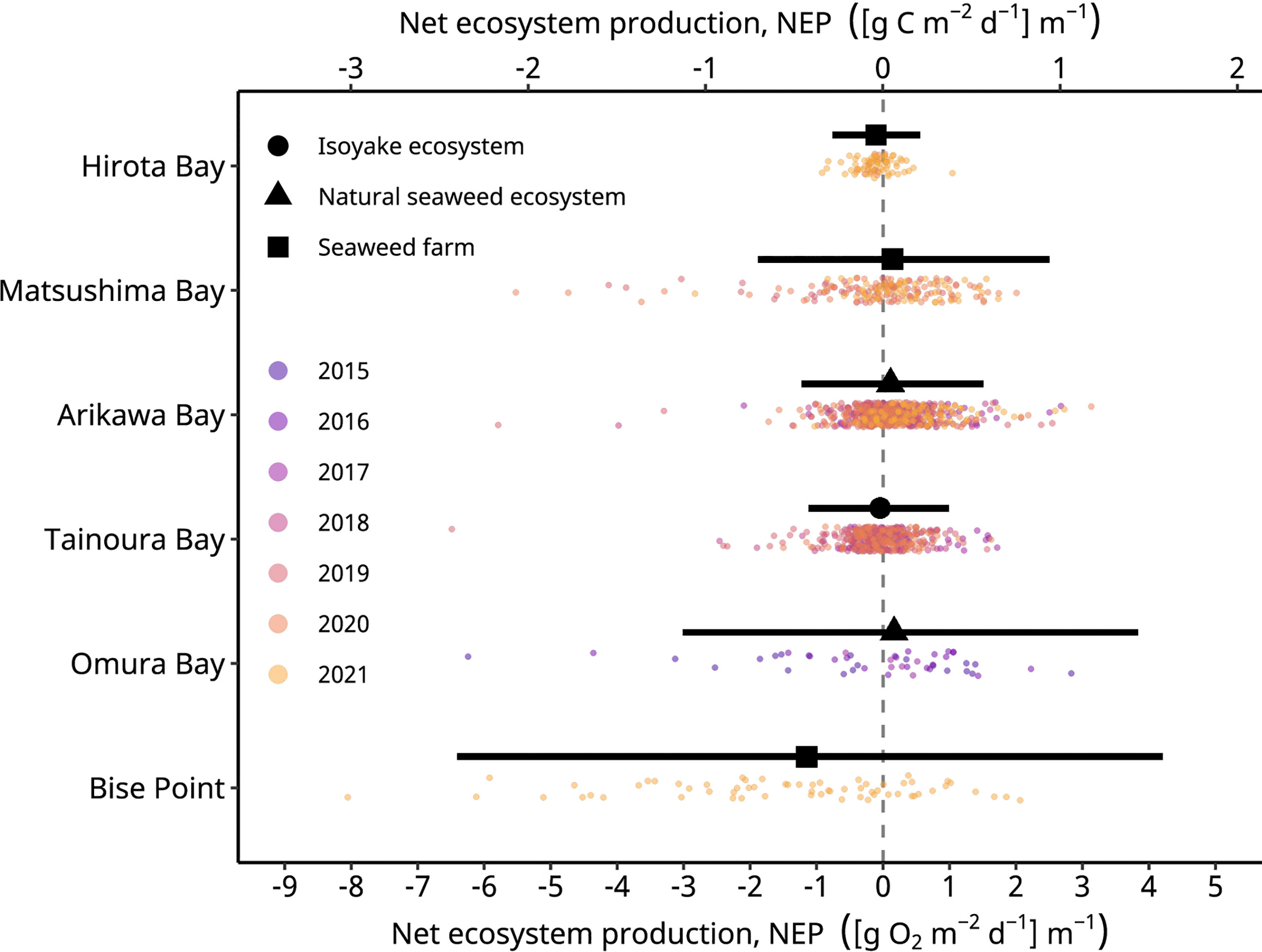
Figure 2 The observed (colored points) and conditional mean of the net ecosystem production (black symbols) rates of the study sites. The black horizontal lines indicate the 95% highest density credible interval of the predicted values. Hirota Bay and Matsushima Bay are the Undaria pinnatifida aquaculture farms, Bise Point is the Cladosiphon okamuranus aquaculture farm, Arikawa Bay and Omura Bay are sites of the natural Sargassum spp. ecosystem, and Tainoura Bay is a site where a seaweed ecosystem has degraded into an isoyake state. The net ecosystem production in O2 and C are calculated assuming a 1 to 1 molar ratio.
In general, the mean daily NEP rates of the observations were negative in all seaweed farms, the natural ecosystem of Omura Bay, and the degraded site of Tainoura Bay (Table 2). The NEP rates were most positive at Arikawa Bay and most negative at the C. okamuranus farm at Bise Point. The mean daily GEP rates of the observations were highest at Bise Point, whereas it was the lowest at the U. pinnatifida farm at Hirota Bay. Natural ecosystems and the U. pinnatifida farm at Matsushima Bay had intermediate values of GEP and ER and followed similar patterns. ER rates reflected the GEP observations.
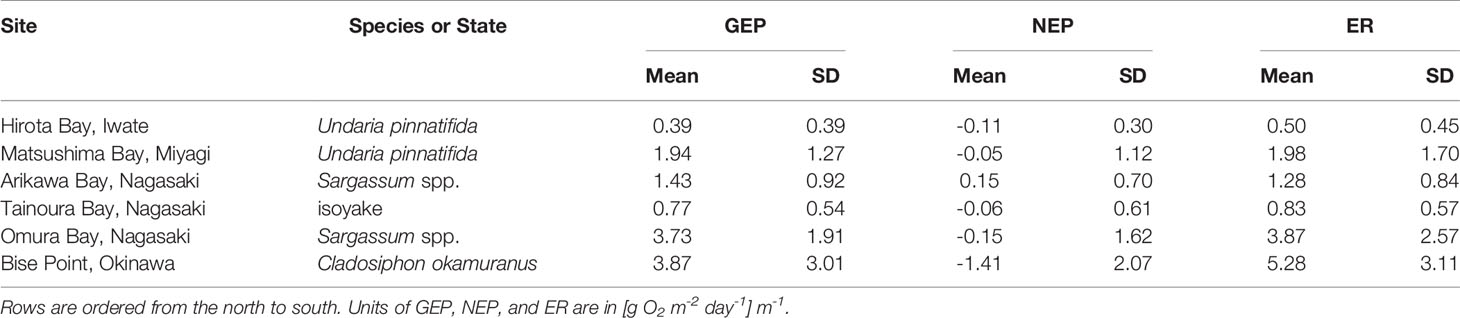
Table 2 The mean and one standard deviation (SD) of the observed rates of gross ecosystem production (GEP), net ecosystem production (NEP), and the ecosystem respiration (ER) determined from three seaweed aquaculture farms (Undaria pinnatifda and Cladosiphon okamuranus) and three natural ecosystems in Japan.
The GLMs clearly indicated that NEP and the frequency of autotrophic days differed among sites.
Unlike the mean values of the observations, the conditional means of the NEP from the GLM analysis followed a slightly different pattern. Applying a scaled t-distribution generally made little to no differences between the unconditional mean of the observation and the conditional means of the GLM. However, the long tails in the observations for Matsushima Bay and Omura Bay lead to slightly negative unconditional means (Table 2), whereas controlling for extreme values led to positive conditional means (Table 3).
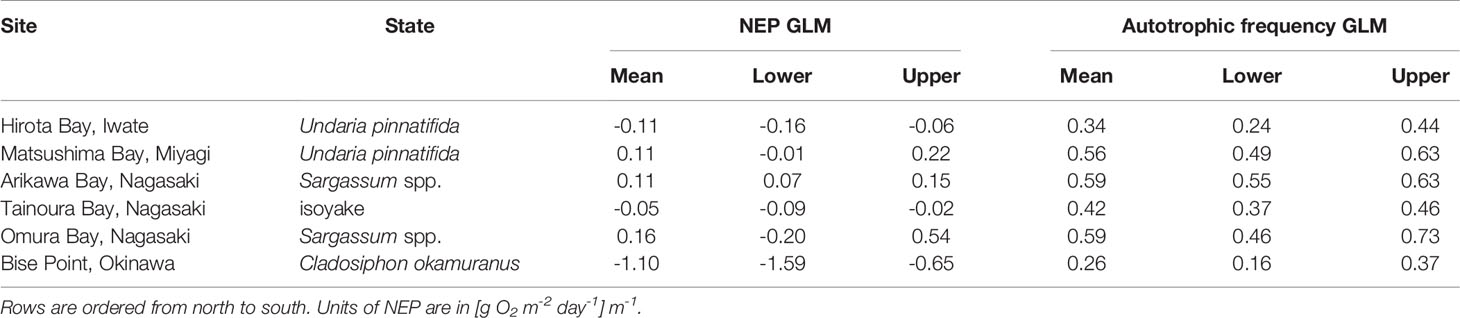
Table 3 The mean and 95% highest density credible interval for the expectations of the generalized linear model (GLM) of the net ecosystem production (NEP) and the autotrophic frequency.
These effects are observed because a scaled t-distribution dampens the effect of relatively extreme observations. The GLM results of the NEP observations indicated that the C. okamuranus farm at Bise Point had the least positive conditional mean value while the natural Sargassum spp. ecosystem at Omura Bay had the most positive conditional mean value (Figure 2). Leave-one-out cross validation indicated that the difference in the expected log pointwise predictive density (elpd) between the NEP GLM and a null model (i.e., no explanatory variables) was 143.2 ± 19.9 (mean ± one standard error), supporting the NEP GLM over the null model (Vehtari et al., 2017). The GLM of the frequency of autotrophy indicated that the C. okamuranus farm at Bise Point was most often heterotrophic (26%); the U. pinnatifida farm at Hirota Bay was the second most heterotrophic site (34%; Figure 3 and Table 3). In contrast, the two Sargassum sp. ecosystems at Arikawa Bay and Omura Bay, and the U. pinnatifida farm at Matsushima Bay were most often autotrophic (> 50%). The GLM of the autotrophic frequency had an elpd of 24.1 ± 7.6, indicating support over the null model.
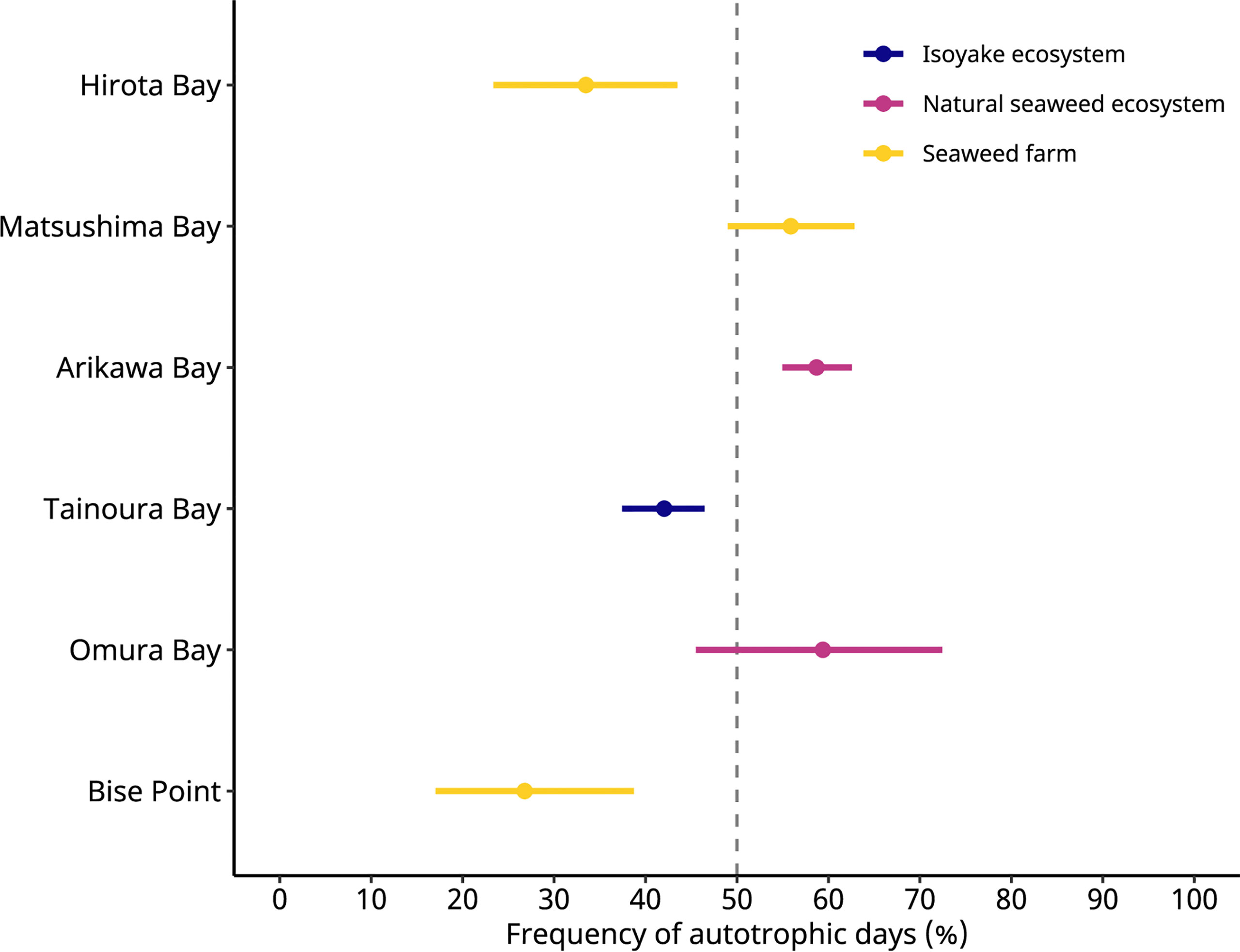
Figure 3 The frequency of autotrophic days predicted by a generalized linear model. The colored points indicate the predicted values, and the colored lines indicate the 95% highest density credible interval. Hirota Bay and Matsushima Bay are the Undaria pinnatifida aquaculture farms, Bise Point is the Cladosiphon okamuranus aquaculture farm, Arikawa Bay and Omura Bay are sites of the natural Sargassum spp. ecosystem, and Tainoura Bay is a site where a seaweed ecosystem has degraded into an isoyake state.
The total yield for each of the farms are known, and was 120, 40, and 6.8 tons for the U. pinnatifida farms in Hirota Bay, Matsushima Bay, and the C. okamuranus farm at Bise Point, respectively (Table 4). The intensity of the carbon yield was calculated after determining the % carbon content of the cultivated seaweeds and ranged from 3.7 to 14.1 g C m-2 (Table 4).

Table 4 The total yield and estimated amount of carbon captured by the seaweed farms at Hirota Bay, Matsushima Bay, and Bise Point during their respective cultivation season.
Discussion
An ultimate goal would be to estimate the carbon sequestration rate of seaweed farms; however, without information regarding the export and import of carbon from allochthonous sources (see Gallagher et al., 2022), as well as the knowing the fate of cultivated seaweed, we can only estimate the carbon sequestration potential of our study sites. Nevertheless, we provide field estimates of the carbon sequestration potential of seaweed farm sites in Japan and also provide estimates of natural ecosystems and a degraded ecosystem for context. We expected NEP to be more positive in seaweed farms compared to the natural and degraded ecosystems, since natural ecosystems are expected to be primarily heterotrophic (Gallagher et al., 2022) and degraded ecosystem are deficient in primary producers, but our results were mixed and given the limitations of the data the model results provided should not be interpreted strictly as one system functioning more efficiently than the other. Nevertheless, the key finding of this study was that the field data clearly shows how seaweed aquaculture may not necessarily lead to carbon capture, and the potential to capture carbon may depend on taxa. For example, the conditional means of NEP for the U. pinnatifida farm at Matsushima Bay was 0.041 [g C m-2 d-1] m-1 (Figure 2, Table 3). Among the seaweed farms, this site had the second highest rate of GEP (Table 2) and was autotrophic 56% of the time (Figure 3 and Table 3). In contrast, the C. okamuranus aquaculture farm at Bise Point had the highest rate of GEP among the aquaculture farms, however the NEP was most negative (Figure 1 and Tables 2, 3), and the aquaculture farm was only autotrophic 26% of the time (Figure 2 and Table 3).
Within taxa, the two U. pinnatifida seaweed farms clearly differed in NEP, where the conditional mean difference NEP between the Hirota Bay (-0.041 [g C m-2 d-1] m-1) and Matsushima Bay (+0.041 [g C m-2 d-1] m-1) differed by aquaculture farms was -0.083 (-0.13 to -0.038) [g C m-2 d-1] m-1 (95% highest density credible interval). However, the intensity of carbon yield at Hirota Bay was 3.8 times greater than at Matsushima Bay (Table 4). We speculate that greater carbon yield intensities occurring at the Hirota Bay farm stimulated the release of dissolved organic carbon and/or particulate organic carbon by U. pinnatifida (Wada et al., 2008; Chen et al., 2020). Although some of this material is exported out of the ecosystem (Krause-Jensen and Duarte, 2016), the release of this material can result in locally increased rates of ecosystem metabolism, through incorporation into the food-web (Sosik and Simenstad, 2013; Renaud et al., 2015; Paine et al., 2021). Additionally, it is unlikely that the U. pinnatifida produced at the aquaculture farms influenced the results, since both farms used the same cultivar provided by the authors (Sato et al., 2021a). Although the carbon yield intensity was also higher at Hirota Bay, compared to Matsushima Bay, evidence suggests that these differences were due to natural variation (Gao et al., 2014).
The GLM also indicated the C. okamuranus farm at Bise Point was heterotrophic (Table 3). In contrast to U. pinnatifida, which are cultivated on ropes, C. okamuranus are cultivated on nets (Sato et al., 2021b). However, the final yield intensity (i.e., 28 g m-2), was within the range of observed for U. pinnatifida at Hirota and Matsushima Bay (i.e., 52 g m-2 and 15 g m-2, respectively). Therefore, it is possible that stocking density may also influence the NEP of C. okamuranus farms, however since we could only examine one C. okamuranus farm site, the details remain to be revealed. Given that the C. okamuranus farm is deployed in the lagoon of a coral reef, characteristics of the ecosystem, such as the presence of corals and seagrasses nearby, may have led to a low NEP. Coral reef ecosystems were shown to be primarily heterotrophic in a number of studies (Falter et al., 2008; Miller et al., 2009; McGillis et al., 2011).
The potential for seaweed aquaculture farms to capture carbon from the environment and contribute to the mitigation of climate change was noted in a number of studies (Chung et al., 2017). It was suggested that seaweed aquaculture could potentially remove 1 g C m-2 d-1 from the environment based on aquaculture yields (Duarte et al., 2017; Froehlich et al., 2019), which is 1 to 2 orders of magnitude higher than our yield estimates (Table 4). A pioneering study on carbon flux in seaweed farms off the coast of the town Lidao, China, also suggested that seaweed farms could absorb 1.2 g C m-2 d-1 (Jiang et al., 2013). Based on the conditional mean of NEP from the GLM analysis, the potential carbon capture rate was -0.041, +0.041, -0.41 [g C m-2 d-1] m-1, for Hirota Bay, Matsushima Bay, and Bise Point, respectively.
There is an important caveat to these estimates of the seaweed farms examined in our study. Recall that measurements are limited to the production season of the seaweed farms (i.e., October to April), and do not provide a range of rates that would occur over a year. Inclusion of the off-season (i.e., summer to autumn), would likely lead to a decrease in the frequency of autotrophic days, since respiration rates are higher during this period (Bordeyne et al., 2020). Macroalgae ecosystems are generally heterotrophic (Gallagher et al., 2022), and the GLM examining the frequency of autotrophic days also provides some support to these observations (Figure 3). Hence, we suggest that the NEP of seaweed farms are not exceptionally superior to natural ecosystems. Moreover, the limited production period of seaweed aquaculture must be considered when estimating their potential as a carbon mitigating system.
Since we measured oxygen production did not assess lateral flux of carbon, calcification rates, or the production of dissolved organic carbon our estimates of the potential to capture carbon should be considered carefully. Consider that at Bise Point, metabolic activity of nearby seagrasses may have influenced our oxygen observations. In seaweed ecosystems a significant portion of captured carbon is expected to be exported to adjacent ecosystems or as far as the deep ocean (Duarte and Cebrián, 1996; Krause-Jensen and Duarte, 2016; Ortega et al., 2019). Indeed, up to 50% of carbon captured by a Sargassum horneri ecosystem is believed to be exported out of the ecosystem (Watanabe et al., 2020). Additionally, many calcifying species produce calcium carbonate, with a net release of CO2 into the environment during the calcification process, which can affect the net flux of CO2, but not O2 into the atmosphere (Frankignoulle et al., 1994).
In terms of blue carbon strategies, seaweed farms provides a large opportunity to mitigate climate change, given that it is easier and cheaper to scale-up, in contrast to the restoration of ecosystems, such as seagrass ecosystems (Gattuso et al., 2018; Lovelock and Duarte, 2019; Wu et al., 2020) and seaweed ecosystems (Eger et al., 2022). The net flux of carbon into seaweed farms was demonstrated in a number of studies (Tang et al., 2011; Jiang et al., 2013). However, to serve as a mitigation strategy, the carbon captured by seaweed aquaculture must stored over long time scales. If all the seaweed produced by the farms could have been protected from conversion to CO2, 43,384 kg of carbon would have been stored during the 2021 season (Table 4). Although we support past studies that suggests seaweed ecosystems can play a significant role in the mitigation of climate change and provide enough carbon capture capability to be included in carbon offset mechanisms, there remains a number of key questions that must be addressed before governments rush to develop seaweed farms to mitigate and offset carbon emissions. Uninformed expansion of seaweed farms can potentially cause a negative effect on surrounding and underlying ecosystems (Campbell et al., 2019). For example, in shallow water seaweed farms, the cultivation of carrageenophytes (e.g., Kappaphycus and Eucheuma) led to reductions in productivity and shoot density of the underlying seagrass ecosystems (Eklöf et al., 2005; Kelly et al., 2020; Moreira-Saporiti et al., 2021). Light reduction and the removal of nutrients by seaweed farms change the composition of phytoplankton (Jiang et al., 2020; Aldridge et al., 2021). Water flow and tidal exchange rates can decrease leading to increased sedimentation rates and poor water exchange (Zeng et al., 2015). The expansion of seaweed farms can be associated with increased flux of plastic materials from discarded and lost equipment (e.g., synthetic polymer ropes) and plastic particles, exacerbating existing problems with marine plastic pollution (Derraik, 2002; Andrady, 2011; Krüger et al., 2020). An increase in the albedo of the sea surface may also occur, since seaweeds are better able to reflect short-wave radiation than the sea surface, enhancing local cooling of the environment (Bach et al., 2021). Steps towards a compromise between environmental disturbance and carbon capture via seaweed farms can be found in the proper site selection and monitoring of environmental conditions to optimize the timing of the grow-out period. For example, kelps cultured in exposed conditions exhibit the best growth performance with increased carbon content in their tissues (Peteiro and Freire, 2013; Visch et al., 2020). On the other hand, culturing seaweeds in nutrient limited waters leads to nutrient stress and a subsequent loss in seaweed biomass and a net increase in dissolved organic matter in the culture area (Yoshikawa et al., 2001; Paine et al., 2021). For temperate species, timing the grow-out and harvest phase is critical as warmer surface temperatures promote the growth of fouling organisms (Visch et al., 2020).
Reliable long-term field observations of carbon capture rates by macroalgae ecosystems remains scarce. Presently, most assessments of carbon capture rates rely on extrapolating from chambered photosynthesis experiments of using phytoelements or the whole thallus of macroalgae, measuring the CO2 flux at the air-sea interface and the O2 flux at the water-sediment interface using eddy covariance techniques (e.g., Berg et al., 2022), extrapolate from simulations and limited in temporal scope (e.g., Watanabe et al., 2020), extrapolate from O2 mass-balances (e.g., Champenois and Borges, 2012; Hinode et al., 2020), measuring the O2 flux at the water-sediment, and isotopic measurements (e.g., Holtgrieve et al., 2010; Staehr et al., 2012; Hoellein et al., 2013). All methods have both advantages and disadvantages, that must be considered prior to data interpretation. The method we used was comparatively inexpensive and can provide a long and continuous time-series of measurements, but introduces bias due to the assumptions used to estimate air-water flux of oxygen, the horizontal and vertical heterogeneity of dissolved oxygen concentrations and flux (Berg et al., 2022), and the assumption that night-time respiration adequately describes respiration rates during the day. However, all methods suffer from the inability to assess the carbon flux into and out of the system of interest and are unable to precisely assess whether or not the system is a carbon donor or carbon receiver (Hill et al., 2015). Methods to correct for advection and include more ecosystem level processes could enhance the accuracy of carbon flux estimates (Chung et al., 2013; Gruber et al., 2017; Gallagher et al., 2022). More critically, macroalgae can secrete organic carbon and about 18 to 62% of their productivity may be secreted as dissolved carbon, which can be respired by microbes (Wada et al., 2007; Wada et al., 2008; Paine et al., 2021).
The potential of seaweed farms to mitigate climate change remains to be determined. The effectiveness of aquaculture farms to capture and remove carbon for relevant time-scales remains a challenge. Despite this, seaweed farms are still highly valuable for their contribution to the livelihood of coastal communities and have lesser detrimental impacts to the environment compared to other forms of aquaculture (Walls et al., 2017; Visch et al., 2020). We believe that the potential to capture carbon will not be homogenous across species and location, and that more studies to address carbon flux at organismal and ecosystem scales remain to be conducted. Despite these uncertainties, land-based aquaculture of seaweeds and the development of technology to lengthen the production season and yield should provide seaweed aquaculture with a unique opportunity to contribute to the mitigation of climate change (e.g., Sato et al., 2021a). However, the fate of the produced seaweeds will define the effectiveness of these strategies. Finally, detailed measurements of carbon capture from the aquaculture farms of carrageenophytes, chlorophytes such as Ulva spp. and Monostroma spp., some species of Pyropia spp., Saccharina spp., and Sargassum spp., are worthy of future investigation.
Data Availability Statement
The raw data supporting the conclusions of this article will be made available by the authors, without undue reservation.
Author Contributions
YS, GN, AT, RT, and HE conceptualized and supervised the research, YS, GN, AT, and RT acquired funding support, YS, GN, and DB prepared the original draft of the manuscript, YS, GN, AT, RT, HE, and DB reviewed and edited the manuscript. YS, GN, AT, DB, AK, YI, KH, ST, YM, and KT conducted the research and collected data, YS, GN, DB, AK, YI, KH, ST, YM, and KT analyzed the model and prepared figures for the manuscript. GN administered the project. All authors contributed to the article and approved the submitted version.
Funding
This work was supported by JSPS KAKENHI Grant Number 20H03076, 16H02939, and 25450260.
Conflict of Interest
Author YS is employed by Riken Food Co., Ltd.
The remaining authors declare that the research was conducted in absence of any commercial or financial relationships that could be construed as a potential conflict of interest.
Publisher’s Note
All claims expressed in this article are solely those of the authors and do not necessarily represent those of their affiliated organizations, or those of the publisher, the editors and the reviewers. Any product that may be evaluated in this article, or claim that may be made by its manufacturer, is not guaranteed or endorsed by the publisher.
Acknowledgments
The authors would like to thank the Omura Bay Fisheries Cooperative and Arikawa Bay Fisheries Cooperative of Nagasaki, Shiogama City Fisheries Cooperative of Miyagi, Hirota Bay Fisheries Cooperative of Iwate, and the Motobu Fisheries Cooperative of Okinawa for their insights and cooperation which allowed us to conduct the field surveys in their seaweed aquaculture farms.
Supplementary Material
The Supplementary Material for this article can be found online at: https://www.frontiersin.org/articles/10.3389/fmars.2022.861932/full#supplementary-material
References
Aldridge J. N., Mooney K., Dabrowski T., Capuzzo E. (2021). Modelling Effects of Seaweed Aquaculture on Phytoplankton and Mussel Production. Application to Strangford Lough (Northern Ireland). Aquaculture 536, 736400. doi: 10.1016/j.aquaculture.2021.736400
Andrady A. L. (2011). Microplastics in the Marine Environment. Mar. Poll. Bull. 62, 1596–1605. doi: 10.1016/j.marpolbul.2011.05.030
Bach L. T., Tamsitt V., Gower J., Hurd C. L., Raven J. A., Boyd P. W. (2021). Testing the Climate Intervention Potential of Ocean Afforestation Using the Great Atlantic Sargassum Belt. Nat. Commun. 12, 2556. doi: 10.1038/s41467-021-22837-2
Berg P., Delgard M. L., Polsenaere P., McGlathery K. J., Doney S. C., Berger A. C. (2019). Dynamics of Benthic Metabolism, O2, and Pco2 in a Temperate Seagrass Meadow. Limnol. Oceanogr. 64, 2586–2604. doi: 10.1002/lno.11236
Berg P., Huettel M., Glud R. N., Reimers C. E., Attard K. M. (2022). Aquatic Eddy Covariance: The Method and Its Contributions to Defining Oxygen and Carbon Fluxes in Marine Environments. Annu. Rev. Mar. Sci. 14431–455. doi: 10.1146/annurev-marine-042121-012329
Bivand R., Lewkin-Koh N. (2021) Maptools: Tools for Handling Spatial Objects. R Package Version 1.1-2. Available at: https://cran.r-project.org/package=maptools.
Blake S. (2020) Fetchr: Calculate Wind Fetch. R Package Version 2.1-2. Available at: https://cran.r-project.org/package=fetchR.
Bordeyne F., Migné A., Plus M., Davoult D. (2020). Modelling the Annual Primary Production of an Intertidal Brown Algal Community Based on in Situ Measurements. Mar. Ecol. Prog. Ser. 656, 95–107. doi: 10.3354/meps13450
Boysen L. R., Lucht W., Gerten D. (2017). Trade-Offs for Food Production, Nature Conservation and Climate Limit the Terrestrial Carbon Dioxide Removal Potential. Global Change Biol. 23, 4303–4317. doi: 10.1111/gcb.13745
Bürkner P.-C. (2017). Brms : An R Package for Bayesian Multilevel Models Using Stan. J. Stat. Softw. 80, 1–28. doi: 10.18637/jss.v080.i01
Bürkner P. C. (2018). Advanced Bayesian Multilevel Modeling With the R Package Brms. R. J. 10, 395–411. doi: 10.32614/rj-2018-017
Campbell I., Macleod A., Sahlmann C., Neves L., Funderud J., Øverland M., et al. (2019). The Environmental Risks Associated With the Development of Seaweed Farming in Europe - Prioritizing Key Knowledge Gaps. Front. Mar. Sci. 6. doi: 10.3389/fmars.2019.00107
Champenois W., Borges A. (2012). Seasonal and Interannual Variations of Community Metabolism Rates of a Posidonia Oceanica Seagrass Meadow. Limnol. Oceanogr. 57, 347–361. doi: 10.4319/lo.2012.57.1.0347
Chen S., Xu K., Ji D., Wang W., Xu Y., Chen C., et al. (2020). Release of Dissolved and Particulate Organic Organic Matter by Marine Macroalgae and its Biogeochemical Implications. Algal. Res. 52, 102096. doi: 10.1016/j.algal.2020.102096
Chung I. K., Beardall J., Mehta S., Sahoo D., Stojkovic S. (2011). Using Marine Macroalgae for Carbon Sequestration: A Critical Appraisal. J. Appl. Phycol. 23, 877–886. doi: 10.1007/s10811-010-9604-9
Chung I. K., Oak J. H., Lee J. A., Shin J. A., Kim J. G., Park K.-S. (2013). Installing Kelp Forests/Seaweed Beds for Mitigation and Adaptation Against Global Warming: Korean Project Overview. ICES. J. Mar. Sci. 70, 1038–1044. doi: 10.1093/icesjms/fss206
Chung I. K., Sondak C. F. A., Beardall J. (2017). The Future of Seaweed Aquaculture in a Rapidly Changing World. Eur. J. Phycol. 52, 495–505. doi: 10.1080/09670262.2017.1359678
Derraik J. G. B. (2002). The Pollution of the Marine Environment by Plastic Debris: A Review. Mar. Poll. Bull. 44, 842–852. doi: 10.1016/S0025-326X(02)00220-5
Duarte C. M. (2017). Reviews and Syntheses: Hidden Forests, the Role of Vegetated Coastal Habitats in the Ocean Carbon Budget. Biogeosciences 14, 301–310. doi: 10.5194/bg-14-301-2017
Duarte C. M., Cebrián J. (1996). The Fate of Marine Autotrophic Production. Limnol. Oceanogr. 41, 1758–1766. doi: 10.4319/lo.1996.41.8.1758
Duarte C. M., Krause-Jensen D. (2017). Export From Seagrass Meadows Contributes to Marine Carbon Sequestration. Front. Mar. Sci. 4. doi: 10.3389/fmars.2017.00013
Duarte C. M., Wu J., Xiao X., Bruhn A., Krause-Jensen D. (2017). Can Seaweed Farming Play a Role in Climate Change Mitigation and Adaptation? Front. Mar. Sci. 4. doi: 10.3389/fmars.2017.00100
Eger A. M., Marzinelli E. M., Christie H., Fagerli C. W., Fujita D., Gonzales A. P., et al. (2022). Global Kelp Forest Restoration: Past Lessons, Present Status, and Future Directions. Biol. Rev. doi: 10.1111/brv.12850
Eklöf J. S., de la Torre Castro M., Adelsköld L., Jiddawi N. S., Kautsky N. (2005). Differences in Macrofaunal and Seagrass Assemblages in Seagrass Beds With and Without Seaweed Farms. Estuar. Coast. Shelf. Sci. 63, 385–396. doi: 10.1016/j.ecss.2004.11.014
Falter J. L., Lowe R. J., Atkinson M. J., Monismith S. G. (2008). Continuous Measurements of Net Production Over a Shallow Reef Community Using a Modified Eulerian Approach. J. Geophys. Res. 113, C07035. doi: 10.1029/2007JC004663
Fillbee-Dexter K., Wernberg T. (2020). Substantial Blue Carbon in Overlooked Australian Kelp Forests. Sci. Rep. 10, 1–6. doi: 10.1038/s41598-020-69258-7
Frankignoulle M., Canon C., Gattuso J.-P. (1994). Marine Calcification as a Source of Carbon Dioxide: Positive Feedback of Increasing Atmospheric CO2. Limnol. Oceanogr. 39, 458–462. doi: 10.4319/lo.1994.39.2.0458
Froehlich H. E., Afflerbach J. C., Frazier M., Halpern B. S. (2019). Blue Growth Potential to Mitigate Climate Change Through Seaweed Offsetting. Curr. Biol. 29, 3087–3093.e3. doi: 10.1016/j.cub.2019.07.041
Frontier N., de Bettignies F., Foggo A., Davoult D. (2021). Sustained Productivity and Respiration of Degrading Kelp Detritus in the Shallow Benthos: Detached or Broken, But Not Dead. Mar. Environ. Res. 166, 105277. doi: 10.1016/j.marenvres.2021.105277
Fujita D. (2010). Current Status and Problems of Isoyake in Japan. Bull. Fish. Res. Agen. 32, 33–42.
Gallagher J. B., Shelamoff V., Layton C. (2021). Missing the Forest for the Trees: A Reappraisal of Global Seaweed Carbon Sequestration. bioRxiv. doi: 10.1101/2021.09.05.459038
Gallagher J. B., Shelamoff V., Layton C. (2022). Seaweed Ecosystems may Not Mitigate CO2 Emissions. ICES. J. Mar. Sci. 79, 585–592. doi: 10.1093/icesjms/fsac011
Gao X., Endo H., Taniguchi K., Agatsuma Y. (2014). Effects of Experimental Thinning on the Growth and Maturation of the Brown Alga Undaria Pinnatifida (Laminariales; Phaeophyta) Cultivated in Matsushima Bay, Northern Japan. J. Appl. Phycol. 26, 529–535. doi: 10.1007/s10811-013-0071-y
Gattuso J. P., Magnan A. K., Bopp L., Cheung W. W. L., Duarte C. M., Hinkel J., et al. (2018). Ocean Solutions to Address Climate Change and its Effects on Marine Ecosystems. Front. Mar. Sci. 5. doi: 10.3389/fmars.2018.00337
Gauthier P. P. G., Battle M. O., Griffin K. L., Bender M. L. (2018). Measurement of Gross Photosynthesis, Respiration in the Light, and Mesophyll Conductance Usingh2 18o Labeling. Plant Physiol. 177, 62–74. doi: 10.1104/pp.16.00741
Gouvêa L. P., Assis J., Gurgel C. F. D., Serrão E. A., Silveira T. C. L., Santos R., et al. (2020). Golden Carbon of Sargassum Forests Revealed as an Opportunity for Climate Change Mitigation. Sci. Tot. Environ. 729, 138745. doi: 10.1016/j.scitotenv.2020.138745
Gruber R. K., Lowe R. J., Falter J. L. (2017). Metabolism of a Tide-Dominated Reef Platform Subject to Extreme Diel Temperature and Oxygen Variations. Limnol. Oceanogr. 62, 1701–1717. doi: 10.1002/lno.10527
Hanson P. C., Carpenter S. R., Kimura N., Wu C., Cornelius S. P., Kratz T. K. (2008). Evaluation of Metabolism Models for Free-Water Dissolved Oxygen Methods in Lakes. Limnol. Oceanogr.: Methods 6, 454–465. doi: 10.4319/lom.2008.6.454
Higuchi T., Takagi K. K., Matoba K., Kobayashi S., Tsurumi R., Arakaki S., et al. (2014). The Nutrient and Carbon Dynamics That Mutually Benefit Coral and Seagrass in Mixed Habitats Under the Influence of Groundwater at Bise Coral Reef, Okinawa, Japan. Int. J. Mar. Sci. 4, 1–15. doi: 10.5376/ijms.2014.04.0001
Hill R., Bellgrove A., Macreadie P. I., Petrou K., Beardall J., Steven A., et al. (2015). Can Macroalgae Contribute to Blue Carbon? An Australian Perspective. Limnol. Oceanogr. 60, 1689–1706. doi: 10.1002/lno.10128
Hinode K., Punchai P., Saitsu M., Nishihara G. N., Inoue Y., Terada R. (2020). The Phenology of Gross Ecosystem Production in a Macroalga and Seagrass Canopy is Driven by Seasonal Temperature. Phycol. Res. 68, 298–312. doi: 10.1111/pre.12433
Hoellein T. J., Bruesewitz D. A., Richardson D. C. (2013). Revisiting Odu): A Synthesis of Aquatic Ecosystem Metabolism. Limnol. Oceanogr. 58, 2089–2100. doi: 10.4319/lo.2013.58.6.2089
Holtgrieve G. W., Schindlern D. E., Branch T. A., Teresa A’Mar Z. (2010). Simultaneous Quantification of Aquatic Ecosystem Metabolism and Reaeration Using a Bayesian Statistical Model of Oxygen Dynamics. Limnol. Oceanogr. 55, 1047–1063. doi: 10.4319/lo.2010.55.3.1047
Howard J., Sutton-Grier A., Herr D., Kleypas J., Landis E., Mcleod E., et al. (2017). Clarifying the Role of Coastal and Marine Systems in Climate Mitigation. Front. Ecol. Environ. 15, 42–50. doi: 10.1002/fee.1451
Ichikawa T., Sakai T., Morozumi K., Suzuki T. (2009). A Numerical Simulation of T-S Structure and Velocity Fields in Hirota Bay. J. Advanced Mar. Sci. Technol. Soc. 15, 125–135. doi: 10.14928/amstec.15.2_125
Jiang Z., Fang J., Mao Y., Han T., Wang G. (2013). Influence of Seaweed Aquaculture on Marine Inorganic Carbon Dynamics and Sea-Air CO 2 Flux. J. World Aquacult. Soc. 44, 133–140. doi: 10.1111/jwas.12000
Jiang Z., Liu J., Li S., Chen Y., Du P., Zhu Y., et al. (2020). Kelp Cultivation Effectively Improves Water Quality and Regulates Phytoplankton Community in a Turbid, Highly Eutrophic Bay. Sci. Tot. Environ. 707, 135561. doi: 10.1016/j.scitotenv.2019.135561
Jianxi H., Mizobata K., Kitade Y. (2020). Effect of Wind on Seawater Exchange in Matsushima Bay. La. Mer. 58, 17–33. doi: 10.32211/lamer.58.1-2_17
Kelly E. L. A., Cannon A. L., Smith J. E. (2020). Environmental Impacts and Implications of Tropical Carrageenophyte Seaweed Farming. Conserv. Biol. 34, 326–337. doi: 10.1111/cobi.13462
Krause-Jensen D., Duarte C. M. (2016). Substantial Role of Macroalgae in Marine Carbon Sequestration. Nat. Geosci. 9, 737–742. doi: 10.1038/ngeo2790
Krause-Jensen D., Lavery P., Serrano O., Marba N., Masque P., Duarte C. M. (2018). Sequestration of Macroalgal Carbon: The Elephant in the Blue Carbon Room. Biol. Lett. 14, 20180236. doi: 10.1098/rsbl.2018.0236
Krüger L., Casado-Coy N., Valle C., Ramos M., Sánchez-Jerez P., Gago J., et al. (2020). Plastic Debris Accumulation in the Seabed Derived From Coastal Fish Farming. Environ. Poll. 257, 113336. doi: 10.1016/j.envpol.2019.113336
Krumhansl K. A., Scheibling R. E. (2012). Production and Fate of Kelp Detritus. Mar. Ecol. Prog. Ser. 467, 281–302. doi: 10.3354/meps09940
Lovelock C. E., Duarte C. M. (2019). Dimensions of Blue Carbon and Emerging Perspectives. Biol. Lett. 15, 1–5. doi: 10.1098/rsbl.2018.0781
Macreadie P. I., Serrano O., Maher D. T., Duarte C. M., Beardall J. (2017). Addressing Calcium Carbonate Cycling in Blue Carbon Accounting. Limnol. Oceanogr. Lett. 2, 195–201. doi: 10.1002/lol2.10052
McGillis W. R., Langdon C., Loose B., Yates K. K., Corredor J. (2011). Productivity of a Coral Reef Using Boundary Layer and Enclosure Methods. Geophys. Res. Lett. 38, L03611. doi: 10.1029/2010GL046179
McLeod E., Chmura G. L., Bouillon S., Salm R., Björk M., Duarte C. M., et al. (2011). A Blueprint for Blue Carbon: Toward an Improved Understanding of the Role of Vegetated Coastal Habitats in Sequestering CO2. Front. Ecol. Environ. 9, 552–560. doi: 10.1890/110004
Miller R. J., Reed D. C., Brzezinski M. A. (2009). Community Structure and Productivity of Subtidal Turf and Foliose Algal Assemblages. Mar. Ecol. Prog. Ser. 388, 1–11. doi: 10.3354/meps08131
Moreira-Saporiti A., Hoeijmakers D., Msuya F. E., Reuter H., Teichberg M. (2021). Seaweed Farming Pressure Affects Seagrass and Benthic Macroalgae Dynamics in Chwaka Bay (Zanzibar, Tanzania). Reg. Environ. Change 21, 1–12. doi: 10.1007/s10113-020-01742-2
Muraoka D. (2004). Seaweed Resources as a Source of Carbon Fixation. Bull. Fish. Res. Agen. Suppl. 1, 59–63.
Nogami M., Matsuno T. (2001). The Relationship Between Ocean Structure and Formation of the Second Thermocline in Ohmura Bay. Oceanogr. Jap. 10, 191–202. doi: 10.5928/kaiyou.10.191
Ortega A., Geraldi N. R., Alam I., Kamau A. A., Acinas S. G., Logares R., et al. (2019). Important Contribution of Macroalgae to Oceanic Carbon Sequestration. Nat. Geosci. 12, 748–754. doi: 10.1038/s41561-019-0421-8
Paine E. R., Schmid M., Boyd P. W., Diaz-Pulido G., Hurd C. L. (2021). Rate and Fate of Dissolved Organic Carbon Release by Seaweeds: A Missing Link in the Coastal Ocean Carbon Cycle. J. Phycol. 57, 1375–1391. doi: 10.1111/jpy.13198
Pedersen M. F., Filbee-Dexter K., Norderhaug K. M., Fredriksen S., Frisk N. L., Fagerli C. W., et al. (2020). Detrital Carbon Production and Export in High Latitude Kelp Forests. Oecologia 192, 227–239. doi: 10.1007/s00442-019-04573-z
Peteiro C., Freire Ó. (2013). Biomass Yield and Morphological Features of the Seaweed Saccharina Latissima Cultivated at Two Different Sites in a Coastal Bay in the Atlantic Coast of Spain. J. Appl. Phycol. 25, 205–213. doi: 10.1007/s10811-012-9854-9
R Core Team (2021) R: A Language and Environment for Statistical Computing. Available at: https://www.r-project.org/.
Renaud P. E., Løkken T. S., Jørgensen L. L., Berge J., Johnson B. J. (2015). Macroalgal Detritus and Food-Web Subsidies Along an Arctic Fjord Depth-Gradient. Front. Mar. Sci. 2. doi: 10.3389/fmars.2015.00031
Sato Y., Hirano T., Ichida H., Fukunishi N., Abe T., Kawano S. (2021a). Extending the Cultivation Period of Undaria Pinnatifida by Using Regional Strains With Phenotypic Differentiation Along the Sanriku Coast in Northern Japan. Phycology 1, 129–142. doi: 10.3390/phycology1020010
Sato Y., Nagoe H., Ito M., Konishi T., Fujimura H., Nishihara G. N., et al. (2021b). Final Yield of the Brown Alga Cladosiphon Okamuranus (Chordariaceae, Phaeophyta) may Depend on Nursery Quality. Phycol. Res. 69, 159–165. doi: 10.1111/pre.12453
Siegel D. A., Devries T., Doney S. C., Bell T. (2021). Assessing the Sequestration Time Scales of Some Ocean-Based Carbon Dioxide Reduction Strategies. Environ. Res. Lett. 16, 104003. doi: 10.1088/1748-9326/ac0be0
Smale D. A., Pessarrodona A., King N., Moore P. J. (2021). Examining the Production, Export, and Immediate Fate of Kelp Detritus on Open-Coast Subtidal Reefs in the Northeast Atlantic. Limnol. Oceanogr. doi: 10.1002/lno.11970
Smith S. V. (2013). Parsing the Oceanic Calcium Carbonate Cycle: A Net Atmospheric Carbon Dioxide Source or a Sink? L&O e-Books. Association for the Sciences of Limnology and Oceanography (ASLO), Waco, TX. doi: 10.4319/svsmith.2013.978-0-9845591-2-1
Sondak C. F. A., Ang P. O., Beardall J., Bellgrove A., Boo S. M., Gerung G. S., et al. (2017). Carbon Dioxide Mitigation Potential of Seaweed Aquaculture Beds (SABs). J. Appl. Phycol. 29, 2363–2373. doi: 10.1007/s10811-016-1022-1
Sosik E. A., Simenstad C. A. (2013). Isotopic Evidence and Consequences of the Role of Microbes in Macroalgae Detritus-Based Food Webs. Mar. Ecol. Prog. Ser. 494, 107–119. doi: 10.3354/meps10544
Staehr P. A., Bade D., Van de Bogert M. C., Koch G. R., Williamson C., Hanson P., et al. (2010). Lake Metabolism and the Diel Oxygen Technique: State of the Science. Limnol. Oceanogr.: Methods 8, 628–644. doi: 10.4319/lom.2010.8.628
Staehr P. A., Testa J. M., Kemp W. M., Cole J. J., Sand-Jensen K., Smith S. V. (2012). The Metabolism of Aquatic Ecosystems: History, Applications, and Future Challenges. Aquat. Sci. 74, 15–29. doi: 10.1007/s00027-011-0199-2
Tang Q., Zhang J., Fang J. (2011). Shellfish and Seaweed Mariculture Increase Atmospheric CO2 Absorption by Coastal Ecosystems. Mar. Ecol. Prog. Ser. 424, 97–104. doi: 10.3354/meps08979
Trevathan-Tackett S. M., Kelleway J., Macreadie P. I., Beardall J., Ralph P., Bellgrove A. (2015). Comparison of Marine Macrophytes for Their Contributions to Blue Carbon Sequestration. Ecology 96, 3043–3057. doi: 10.1890/15-0149.1
Tsuchiya K., Ehama M., Yasunaga Y., Nakagawa Y., Hirahara M., Kishi M., et al. (2018). Seasonal and Spatial Variation of Nutrients in the Coastal Waters of the Northern Goto Islands , Japan. Bull. Coast. Oceanogr. 55, 125–138. doi: 10.32142/engankaiyo.55.2_125
Vehtari A., Gelman A., Gabry J. (2017). Practical Bayesian Model Evaluation Using Leave-One-Out Cross-Validation and WAIC. Stat Comput. 27, 1413–1432. doi: 10.1007/s11222-016-9696-4
Vergés A., Steinberg P. D., Hay M. E., Poore A. G. B., Campbell A. H., Ballesteros E., et al. (2014). The Tropicalization of Temperature Marine Ecosystems: Climate-Mediated Changes in Herbivory and Community Phase Shifts. Proc. R. Soc. B.: Biol. Sci. 281, 20140846. doi: 10.1098/rspb.2014.0846
Visch W., Nylund G. M., Pavia H. (2020). Growth and Biofouling in Kelp Aquaculture (Saccharina Latissima): The Effect of Location and Wave Exposure. J. Appl. Phycol. 32, 3199–3209. doi: 10.1007/s10811-020-02201-5
Wada S., Aoki M. N., Mikami A., Komatsu T., Tsuchiya Y., Sato T., et al. (2008). Bioavailability of Macroalgal Dissolved Organic Matter in Seawater. Mar. Ecol. Prog. Ser. 370, 33–44. doi: 10.3354/meps07645
Wada S., Aoki M. N., Tsuchiya Y., Sato T., Shinagawa H., Hama T. (2007). Quantitative and Qualitative Analyses of Dissolved Organic Matter Released From Ecklonia Cava Kjellman, in Oura Bay, Shimoda, Izu Peninsula, Japan. J. Exp. Mar. Biol. Ecol. 349, 344–358. doi: 10.1016/j.jembe.2007.05.024
Walls A. M., Kennedy R., Edwards M. D., Johnson M. P. (2017). Impact of Kelp Cultivation on the Ecological Status of Benthic Habitats and Zostera Marina Seagrass Biomass. Mar. Poll. Bull. 123, 19–27. doi: 10.1016/j.marpolbul.2017.07.048
Wanninkhof R. (1992). Relationship Between Wind Speed and Gas Exchange Over the Ocean. J. Geophys. Res. 97, 7373. doi: 10.1029/92JC00188
Watanabe K., Yoshida G., Hori M., Umezawa Y., Moki H., Kuwae T. (2020). Macroalgal Metabolism and Lateral Carbon Flows can Create Significant Carbon Sinks. Biogeosciences 17, 2425–2440. doi: 10.5194/bg-17-2425-2020
Wood S. N. (2011). Fast Stable Restricted Maximum Likelihood and Marginal Likelihood Estimation of Semiparametric Generalized Linear Models. J. R. Stat. Soc. (B.) 73, 3–36. doi: 10.1111/j.1467-9868.2010.00749.x
Wu J., Zhang H., Pan Y., Krause-Jensen D., He Z., Fan W., et al. (2020). Opportunities for Blue Carbon Strategies in China. Ocean. Coast. Manage. 194, 105241. doi: 10.1016/j.ocecoaman.2020.105241
Xiao X., Agustí S., Yu Y., Huang Y., Chen W., Hu J., et al. (2021). Seaweed Farms Provide Refugia From Ocean Acidification. Sci. Tot. Environ. 776, 145192. doi: 10.1016/j.scitotenv.2021.145192
Yoshikawa T., Takeuchi I., Furuya K. (2001). Active Erosion of Undaria Pinnatifida Suringar (Laminariales, Phaeophyceae) Mass-Cultured in Otsuchi Bay in Northeastern Japan. J. Exp. Mar. Biol. Ecol. 266, 51–65. doi: 10.1016/S0022-0981(01)00346-X
Keywords: climate change, aquaculture, blue carbon, dissolved oxygen, seaweed, macroalgae
Citation: Sato Y, Nishihara GN, Tanaka A, Belleza DFC, Kawate A, Inoue Y, Hinode K, Matsuda Y, Tanimae S, Tozaki K, Terada R and Endo H (2022) Variability in the Net Ecosystem Productivity (NEP) of Seaweed Farms. Front. Mar. Sci. 9:861932. doi: 10.3389/fmars.2022.861932
Received: 25 January 2022; Accepted: 22 April 2022;
Published: 24 May 2022.
Edited by:
Hilary Anne Kennedy, Bangor University, United KingdomReviewed by:
Victor Shelamoff, University of Tasmania, AustraliaJohn Gallagher, University of Tasmania, Australia
Copyright © 2022 Sato, Nishihara, Tanaka, Belleza, Kawate, Inoue, Hinode, Matsuda, Tanimae, Tozaki, Terada and Endo. This is an open-access article distributed under the terms of the Creative Commons Attribution License (CC BY). The use, distribution or reproduction in other forums is permitted, provided the original author(s) and the copyright owner(s) are credited and that the original publication in this journal is cited, in accordance with accepted academic practice. No use, distribution or reproduction is permitted which does not comply with these terms.
*Correspondence: Gregory N. Nishihara, greg@nagasaki-u.ac.jp
†These authors have contributed equally to this work and share first authorship