Microenvironments Designed to Support Growth and Function of Neuronal Cells
- 1INM – Leibniz Institute for New Materials, Saarbrücken, Germany
- 2Chemistry Department, Saarland University, Saarbrücken, Germany
Strategies for neural tissue repair heavily depend on our ability to temporally reconstruct the natural cellular microenvironment of neural cells. Biomaterials play a fundamental role in this context, as they provide the mechanical support for cells to attach and migrate to the injury site, as well as fundamental signals for differentiation. This review describes how different cellular processes (attachment, proliferation, and (directional) migration and differentiation) have been supported by different material parameters, in vitro and in vivo. Although incipient guidelines for biomaterial design become visible, literature in the field remains rather phenomenological. As in other fields of tissue regeneration, progress will depend on more systematic studies on cell-materials response, better understanding on how cells behave and understand signals in their natural milieu from neurobiology studies, and the translation of this knowledge into engineered microenvironments for clinical use.
Introduction
Disfunction in the nervous system due to aging, trauma or neurodegeneration leads to severe disabilities and is a major concern in an aging society. Regeneration of nerve functions involves migration and generation of new born cells at the damage site, the oriented growth and branching of axons to reform nerves, and the formation of functional synapses between adjacent neurons (Liu et al., 2011; Lee et al., 2014). Over the last two decades neural tissue regenerative therapies based on stem cell transplantation to the injury site have been explored (Lunn et al., 2011; Gage and Temple, 2013; Casarosa et al., 2014). Different biomaterials have been used as carriers for stem cell delivery in order to improve the viability and to guide differentiation of implanted cells (Roach et al., 2010). These biomaterials are conceived, in part, to reproduce the mechanical properties, morphology and composition of the extracellular matrix (ECM) around neuronal cells.
The next sections present relevant examples of biomaterial designs that support different neuronal processes (adhesion, proliferation, migration or (directional) differentiation) in 2D or 3D formats in vitro, and also nerve tissue regeneration in vivo. The sections in this review are organized attending to the biomaterial-elicited cellular response. Inside each section, the literature is structured along specific material properties exploited to support such response, i.e., (i) biofunctionalization with adhesive proteins, mimetic peptides and growth factors used to mediate specific interactions with cells, (ii) mechanical properties, and (iii) dimensionality and topographical features for guiding axon extension and directional growth. Within each section the response from different cell types is presented. At the end of this article the overall progress and remaining challenges for the future of this field are critically discussed.
Biomaterials that Support Neuronal Cell Adhesion
The most basic requirement for a synthetic biomaterial scaffold for cell growth and tissue regeneration (beyond toxicity) is its ability to support cell attachment (Cooke et al., 2010). For this purpose, synthetic biomaterials for neuronal regeneration are typically coated or functionalized with either (a) polymers which are able to interact with the negatively charged cell membrane, (b) reactive layers that can (covalently) bind to the cell surface, or (c) specific cell adhesive molecules able to interact with adhesive receptors at the cell membrane (i.e., integrins) (Berns et al., 2014; Lu et al., 2014; Akizawa et al., 2016; Hamsici et al., 2017). Positively charged polymers like polylysine (PL), poly(ornithine) (PO), poly(ethyleneimine) (PEI), poly(propyleneimine) (PPI) or poly(allylamine hydrochloride) are traditionally used in neuronal cell cultures (Roach et al., 2010). These positively charged layers interact with the negatively charged cellular membrane and allow cell attachment. Reactive polycatechol coatings like poly(dopamine) or poly(norepinephrine) (pNE) have also been used to immobilize neurons (Park et al., 2014; Kim et al., 2015). Such coatings can form on almost any kind of material. The polycatechol layer can covalently react with proteins from serum forming a protein layer onto the biomaterial that supports fast adhesion of human neural stem cells (hNSCs) (Figure 1A), or bind secreted adhesive proteins from the cells, or directly bind to the surface of the cells (Park et al., 2014).
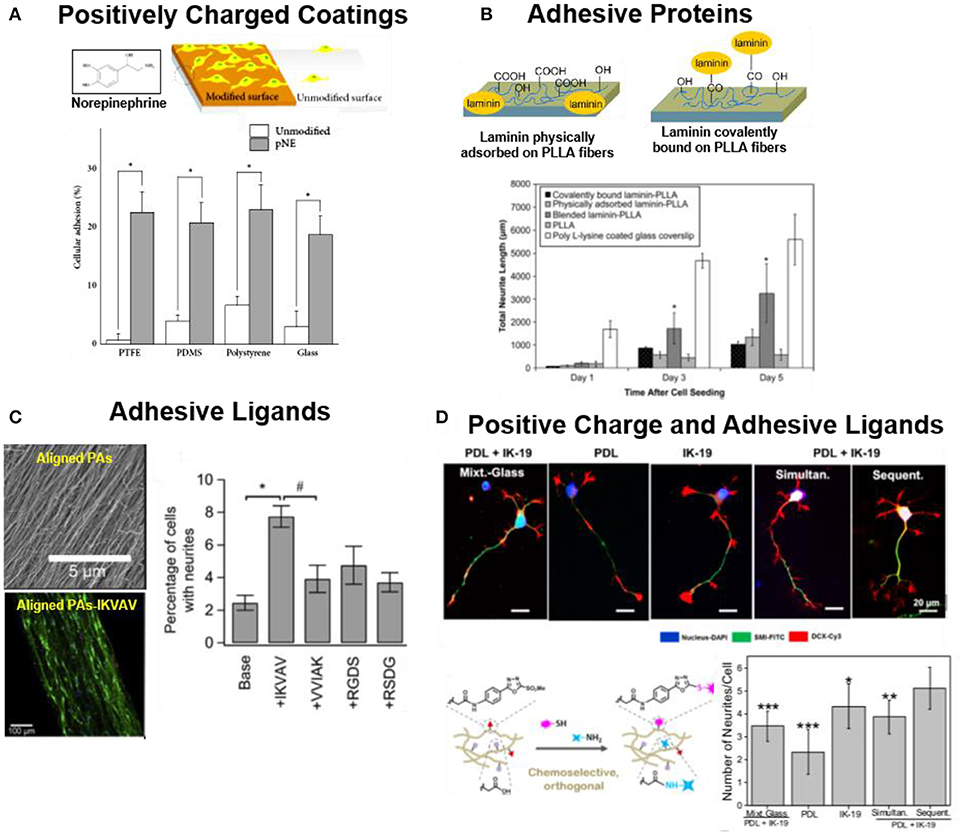
Figure 1. Strategies to support adhesion of neuronal cells to biomaterials (A) hNSCs on pNE coated and uncoated substrates. pNE coating after 2 h of cell seeding significantly enhance cell adhesion (Park et al., 2014) Copyright 2014, Minah Park et al.; (B) PLLA nanofibers coated or covalently functionalized with LN. LN coated substrates support adhesion and neurite extension of PC12 cells (Koh et al., 2008) Copyright 2008, Elsevier; (C) PAs (Palmitoyl-VVAAEE-NH2) functionalized with adhesive epitope to support adhesion of hippocampal neurons. Aligned PA-IKVAV significantly enhance cell adhesion and directional neurite extension during 2 days of culture (Berns et al., 2014) Copyright 2014, Elsevier; (D) IKVAV (IK-19) and PL functionalized polyacrylamide hydrogels either by mixed (simultaneous binding) or by orthogonal coupling chemistries (sequential binding). Sequential binding of PL/IKVAV enhance the cell neurite extension of NPCs, during 5 days of culture in comparison to simultaneous binding (Farrukh et al., 2017b) Copyright 2017, American Chemical Society.
Alternatively to positively charged or reactive coatings, biomaterials can be functionalized with cell adhesive ECM proteins, such as laminin (LN), collagen (CO) or fibronectin (FN) (Yamada, 1989; Belkin and Stepp, 2000; Guarnieri et al., 2007; Durbeej, 2010; Lei et al., 2012; Lee et al., 2014). These proteins offer specific binding sites for adhesion receptors at the cell membrane (i.e., integrins) that mediate cell adhesion. For example, poly(L-lactic acid) (PLLA) nanofibers coated with LN enhanced the attachment and proliferation of PC12 cells vs. unmodified fibers (Figure 1B) (Koh et al., 2008). In some cases, protein fragments containing the binding domains or peptidomimetica are used for surface functionalization instead of the whole protein (Mizuno et al., 2017). Short peptidomimetics are easier to control and manipulate in comparison to the full protein for biomaterial applications. Typical sequences used in neuronal cell cultures are IKVAV, RKRLQVQLSIRT or YIGSR peptides derived from LN, or the RGD binding site from FN (Frith et al., 2012; Kharkar et al., 2013). The YIGSR and RKRLQVQLSIRT peptides support neural cells adhesion, while IKVAV sequence is stated to support differentiation, migration and neurite extension of neural cells (Freitas et al., 2007; Mochizuki et al., 2007; Thid et al., 2007; Frith et al., 2012; Yamada et al., 2012). Functionalized PAs with IKVAV enhanced adhesion by two-folds and aligned neurite extension of hippocampal neurons in comparison to unmodified fibers (Figure 1C) (Berns et al., 2014). Neurotrophic factors (NF) and glial cell line-derived neurotrophic factor (GDNF) have also been combined with adhesion peptides to promote attachment of hNSCs (Kang et al., 2011; Yang et al., 2012; Taylor et al., 2017).
In many cases the nonspecific (electrostatic) and specific (receptor-mediated) functionalization approaches are used together to improve neural adhesion to surfaces. Poly(acrylamide) P(AAm) gels covalently derivatized with IKVAV and PL mixture through orthogonal thiol and amine coupling chemistries enhanced neuronal maturation of mouse embryonic neural progenitor cells (NPCs) by 3-fold in comparison to hydrogels modified only with IKVAV or PL (Figure 1D) (Farrukh et al., 2017b). Neuronal cells behaved differently when seeded on laminin micropatterns on glass or on PEI coated surfaces. Cells on glass/laminin surface extended neurites only on micropatterned laminin lines, while on PEI/laminin surface neurites grew randomly and did not follow the laminin pattern (Liu et al., 2006).
The attachment of the adhesive proteins or ligands to the biomaterial can be performed either by physical adsorption, or by covalent reaction of the adhesive molecule with reactive groups on the materials surface. Physical adsorption is the simplest method, which only requires incubation of the surface with a concentrated protein solution. However, physical interactions are reversible and this might limit the stability of the coating during cell culture time. In addition, immobilization by physical interactions lacks spatial orientation of the absorbed protein and can lead to loss of protein function in the immobilized state. Covalent binding of the adhesive molecules is expected to lead to more homogenous and stable coatings, and eventually allow specific orientation of ligands and more effective binding (Ho et al., 2006). Amines and thiols are the most common reactive groups present in peptides or proteins used for derivatization of functional surfaces. Typically glycidyl, carboxyl or maleimide groups are the reactive functionalities at the surface (Taylor et al., 2017). The performance of covalent vs. physical immobilization strategies for neuronal cell attachment has been addressed in some reports. NSCs seeded on soft chitosan hydrogels (0.5–0.7 kPa) covalently functionalized with LN showed enhanced cell spreading vs. hydrogels with physically adsorbed LN (Wilkinson et al., 2014). In a different study, adult hippocampal NSCs were cultured on phospholipid bilayers supported on glass functionalized with different RGD-containing peptides. NSCs attached to RGD functionalized bilayers in a similar way to glass substrates coated with LN, and underwent differentiation into neurons and astrocytes (Ananthanarayanan et al., 2010).
Typically, neural cells are attached to flat plastic/glass coated surfaces or thin hydrogel coatings for 2D culture. Alternative, more complex or conductive materials can be used in order to implement additional functions to the interface. For example, LN coated graphene films support adhesion of hNSCs during long term cell culture (3–4 weeks) and promote neuronal differentiation (Figure 2A) (Park et al., 2011). Such 2D cell culture environments are attractive due to their simple preparation and facile imaging. Eventually a topology, i.e., a patterned distribution of adhesive molecules, can be superposed on the substrate using established micropatterning methods (typically microcontactprinting), in order to provide directionality or site-specificity to neuronal attachment (Mammadov et al., 2013). For example, polydimethylsiloxane (PDMS) substrates were microcontactprinted with FN, N-cadherin, and Jagged1 proteins to promote spatially resolved adhesion of NSCs (Figure 2B) (Wang et al., 2014). In contrast to in vivo cellular environment where cells can adopt a 3D body shape by attaching to the 3D space, cells in 2D adhere within a single plane and develop an apical-basal polarization that might not be representative for the in vivo case (Caliari and Burdick, 2016). Therefore, 3D culture models of neuronal cells have developed in recent years. Different biomaterials have been used as scaffolds: carbohydrates (alginate, dextran or hyaluronic acid), synthetic hydrogels (poly(ethyleneglycol) (PEG), fibrous matrices of peptide amphiphiles (PAs) nanofibers, or electrospun membranes from degradable polymers, i.e., PLLA, poly(L-lactide-co-glycolide) (PLGA) or silk. These materials are also coated with adhesive proteins, or modified with adhesive bioactive peptide for supporting cell attachment (Cooke et al., 2010). Embryonic stem cell-derived motor neurons (ESMN) encapsulated in PEG hydrogel showed outgrowth of neurites only when the gel was functionalized with KGRGDS sequence (McKinnon et al., 2014). Mouse embryonic stem cells (ESCs) encapsulated into 3D alginate beads modified with FN or hyaluronic acid supported adhesion and differentiation of ESCs into neuronal lineage (Bozza et al., 2014). PEG hydrogel functionalized with IKVAV derived peptide sequence (CCRRIKVAVWLC) supported adhesion and proliferation of hNSCs (Li X. et al., 2014). RGD functionalized hyaluronic acid based 3D hydrogel enhanced 2 folds adhesion and neurite outgrowth of hippocampal NPCs (Figure 2C) (Tarus et al., 2016). Hydrogels formed by nanofibers of peptide amphiphiles (PAs) containing the repeating RADA sequence and different peptidomimetica (RGD for cell adhesion, PFSSTKT for signal transduction and SKPPGTSS for apoptosis inhibition) allowed attachment, survival and proliferation of NSCs into NPCs, neurons, astrocytes and oligodendrocytes during 5 months of cell culture (Koutsopoulos and Zhang, 2013; Zweckberger et al., 2016). The enhanced cell survival on hydrogels of PAs modified with SKPPGTSS (69%), RGD (58%), and PFSSTKT (56%) was significantly higher than on matrigel (37%) or collagen-1 (25%) gels (Koutsopoulos and Zhang, 2013). PA hydrogels containing RGD and IKVAV motif showed enhanced adhesion and proliferation of embedded NSCs in comparison to unmodified fibers (Sun et al., 2016). Spider silk based 3D scaffold has also been used for neural cell culture due to biocompatibility, tunable surface charge and mechanical properties. Primary cortical neurons showed growth, extension and higher expression of neural cell adhesion molecule (NCAM) on recombinant major ampullate spidroin 1 (MaSp1) spider silk. The modification of MaSp1 with GRGGL adhesive sequence (N-cadherin binding site) lead to a significant improvement in neuronal growth (An et al., 2015). The Self-assembling peptides (SAPs) nanofiber scaffolds from K2(QL)6K2(QL6)-IKVAV and RADA16-I-BMHP-1 were tested for spinal cord injury (SCI) model. These peptides increased the stem cell viability and facilitated differentiation into neurons (Zweckberger et al., 2016). A hybrid nanofiber scaffold based on PLGA and RADA16 and modified with bone marrow homing peptide-1 (BMHP-1) supported adhesion and proliferation of rat Schwann cells for application in peripheral nervous system (PNS) repair (Nune et al., 2016).
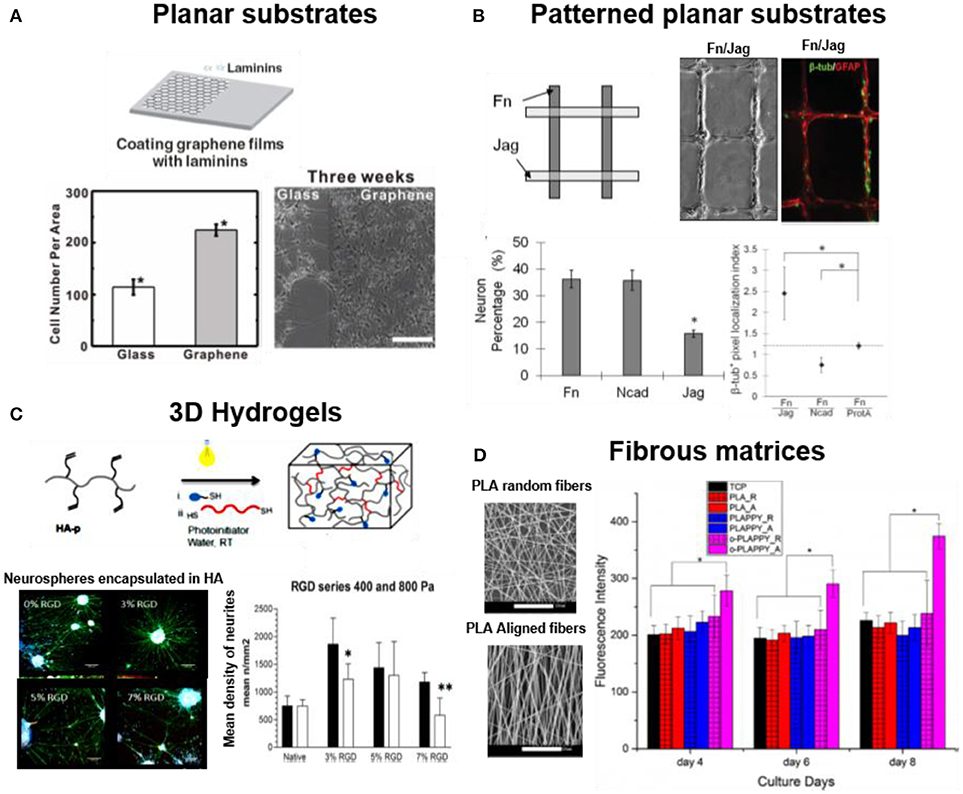
Figure 2. Dimensionality in neuronal cell cultures. Examples of 2D and 3D substrates supporting attachment of neuronal cells (A) hNSCs cultured on laminin coated glass and on graphene film (Park et al., 2011) Copyright 2011, John Wiley and Sons; (B) NSCs cultured on micro-contact printed patterns of FN, Jag or N-cadherin adhesive proteins on PDMS films. FN support higher neuronal cell adhesion while combination of FN/Jag support neuronal differentiation (Wang et al., 2014) Copyright 2014, John Wiley and Sons; (C) Neural cultures in 3D gels of soft (Young's Modulus = 0.4 kPa) photopolymerized hyaluronic acid functionalized with RGD. The soft hydrogel facilitated neurite extension from encapsulated neurospheres during 17 days of culture (Tarus et al., 2016) Copyright 2016, American Chemical Society; (D) Neural cultures on 3D fibrous matrices of aligned (-A) and random PLA fibers (-R), modified with polypyrrole (PLAPPY) and poly-ornithine (o-PLAPPY) (Tian et al., 2016) Copyright 2016, Elsevier.
Topological and topographical features at micro- and nanoscale influence the behavior of neurons in multiple ways, not yet well understood (Micholt et al., 2013; Kulangara et al., 2014; Yang et al., 2014; Nagamine et al., 2015). Line micropatterns with 1–10 μm width and several mm length are the preferred geometrical designs to meet the characteristic elongated morphology of neurons. Either adhesive protein micropatterns on planar substrates (Figure 2) or microchannels have been used for this purpose. Different examples demonstrate positive effect of topographical features in supporting neuronal adhesion. hNSCs showed a two folds enhanced adhesion to LN coated graphene films vs. glass, presumably due to the surface roughness (Park et al., 2011). A micro-grated PDMS substrate coated with PO/LN were designed with dimensions of different heights (0.35–4 μm), width (2 μm), and spacing (2 μm). Higher cell adhesion, alignment (10–50 %), and neuronal differentiation (~10–20%) of murine NPCs was reported in deeper (2 and 4 μm) PDMS channels vs. shallow channels (Chua et al., 2014). Electrospun PLGA nanofibers with smooth and nanorough surfaces (~100–400 nm) were used to culture A-172 cell line derived from human brain. The PLGA fibers with rough surface enhanced the adhesion and up to 50% increase in viability of cells (Zamani et al., 2013). A scaffold of aligned electrospun PLLA nanofibers coated with PO enhanced adhesion and proliferation of PC-12 cells by two-fold in comparison to randomly oriented PLLA-PO fibers (Figure 2D) (Tian et al., 2016). In some cases microtopographies are used to define the direction for neurons to attach and grow. Alginate hydrogels containing microchannels functionalized with RGD supported adhesion and differentiation of bone marrow stromal cells (Lee et al., 2015). Electrospun PLLA fibers coated with graphene oxide (GO) supported adhesion of Schwann cells and PC12. The surface roughness was introduced by coating of GO nanosheets (Zhang et al., 2016).
Biomaterials that Support Proliferation of Neuronal Cells
Low cell proliferation ratio is a major barrier in the clinical success of cell therapies (Karow et al., 2012; Ortega et al., 2013). Similar to adhesive behavior, proliferation varies with material parameters such as coating chemistry, mechanics, dimensionality or morphology.
Functionalization of biodegradable polyesters with hydrophilic and charged functional groups has a positive effect in proliferation ratios. Hydrophilic O2 plasma treated PCL fiber meshes enhanced by ~2 folds the viability and proliferation of mouse ESCs after 3 days of cell culture (Abbasi et al., 2014). Schwann cells showed doubled proliferation ratio on PCL surfaces treated with hexamethylenediamine than on non-functionalized ones during 5 days of cell culture (Luca et al., 2014). Electrospun PLLA-co-PCL/silk fibers loaded with vitamin B5 supported 20% higher proliferation of Schwann cells due to increased surface hydrophilicity (Bhutto et al., 2016).
Several reports demonstrate the positive effect of surface anchored adhesive proteins and peptidomimetics in proliferation. Matrigel functionalized PCL nanofibers enhanced ~2.5 times proliferation of nerve precursor cells during 4 days of cell culture compared to bare PCL (Hiraoka et al., 2009; Ghasemi-Mobarakeh et al., 2010). Laminin peptides from α1 (LP3) or γ1 (LP) chains improved cell survival in in vivo collagen implants. Implanted NSCs encapsulated in the functionalized collagen at striatum of healthy rats showed enhanced cell viability (Nakaji-Hirabayashi et al., 2013). GRGDS modified gellan gum used to culture neural stem progenitor cells (NSPCs) showed 3-fold increase in proliferation than unmodified gellan gum (Silva et al., 2012). Collagen hydrogels functionalized with peptide sequence PPFLMLLKGSTR from LN α3 chain enhanced the viability and proliferation (~4 folds) of neurosphere isolated from striatum of embryonic rat. Schwann cells cultured on core-shell electrospun PLLA-co-PCL nanofibers (316 ± 110 nm) coated with LN showed a 78% enhanced proliferation after 7 days of culture (Kijenska et al., 2014). Hydrogels formed by PA nanofibers functionalized with IKVAV and RGD promoted ~1 fold higher proliferation ratios of Schwann cells after 7–14 days of cell culture. The RGD-PA hydrogels were slightly (~20%) more effective in supporting cell proliferation and maintaining cell viability than IKVAV during 21 days of culture (Li A. et al., 2014). IKVAV functionalized poly(ester carbonate) fibers enhanced cell proliferation (20%) and neurite outgrowth (~5 folds) of PC12 cells compared to unmodified fibers (Xing et al., 2014).
Improvement in cell proliferation was also reported on natural matrices modified with adhesive peptides in combination with NGFs. The fibroblast growth factor-2 (FGF2) embedded in a collagen sponge enhanced the viability and proliferation of NSCs (Ma et al., 2014). Slow release of NGFs (10 ng/mL) from collagen gel enhanced the viability and decreased apoptosis of PC12 cell during 4 days of cell culture more effectively than NGFs directly added to medium (Bhang et al., 2009).
It is now well accepted that the mechanical properties of the natural cellular microenvironment influence the behavior of embedded cells. In a similar manner, the mechanical properties of the culture substrate in 2D or 3D impact cellular processes (Laura et al., 2008; Norman and Aranda-Espinoza, 2010; Hanein et al., 2011). In general culture conditions tend to match the properties of the natural environment. In the case of brain tissue, which belongs to the softest in the body, biomaterials with stiffness 0.1–20 kPa are preferred. For the retina tissue, the stiffness with 1–20 kPa is the preferred mechanical designs for biomaterials. Several reports show how the stiffness of the biomaterial influences proliferation of neuronal cells. Soft P(AAm) hydrogels (2 kPa) functionalized with PL/IKVAV enhance proliferation (11–24%) of NSCs during 4 days of culture vs. glass or stiff gel (20 kPa) (Figure 3A) (Farrukh et al., 2017a). A soft N-carboxyethyl chitosan/oxidized sodium alginate hydrogel with Young's Modulus between 0.1 and 1 kPa was demonstrated to support proliferation and differentiation of NSCs. Enhanced cell proliferation (~1 fold) and a 38% increase in neuronal differentiation was observed in the hydrogel with Young's Modulus 0.5 kPa. This hydrogel is also injectable and, therefore, it could be a suitable carrier for NSCs based regeneration (Wei et al., 2016). A study of NSCs proliferation in materials with different stiffness was reported using thermo-responsive polyurethane hydrogels with varying compositional ranges with Young's Modulus between 0.68 and 2.4 kPa. NSCs on 0.68 kPa gels proliferated 30% faster than cells on 2.4 kPa hydrogels (Figure 3B). Low stiffness facilitated NSCs survival and growth (Hsieh et al., 2015). Electrospun blends of silk fibroin (SF) and poly(L-lactic acid-co-ε-caprolactone) (PLLA-co-PCL) with Young's Modulus between 13 and 120 MPa supported adhesion, proliferation and preferential differentiation of retinal progenitor cells (RPCs) into retinal neurons. The cells show highest proliferation on 105 MPa SF:PLCL (1:1) substrates (Zhang et al., 2015).
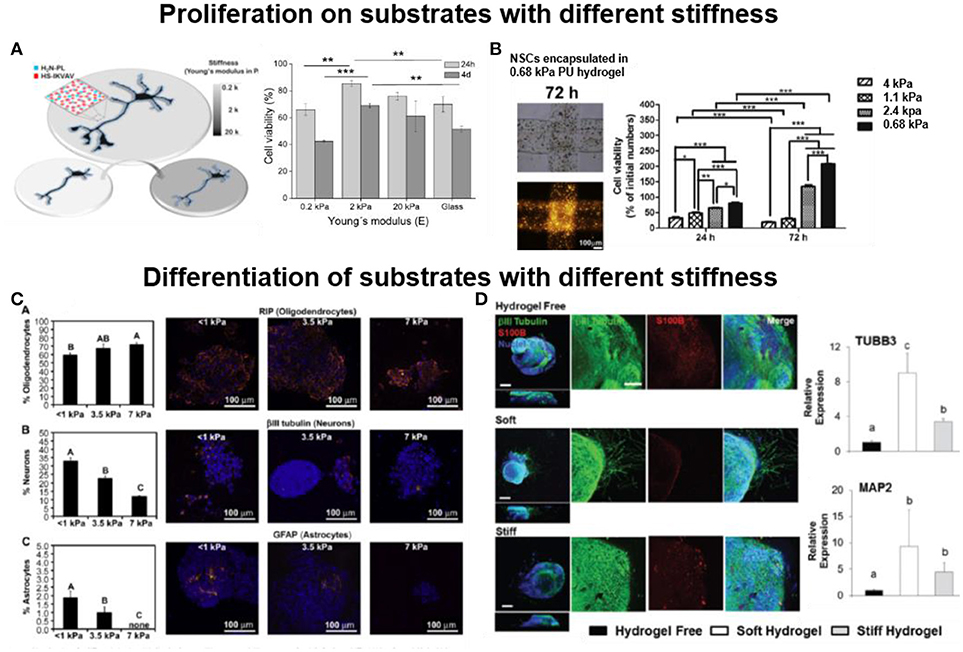
Figure 3. Influence of the stiffness of the biomaterial in cell proliferation and differentiation (A) Soft (2 kPa) 2D polyacrylamide hydrogels functionalized with PL and IKVAV support proliferation of NSCs during 4 days of culture (Farrukh et al., 2017a) Copyright 2017, The Authors; (B) Soft (0.68 kPa) 3D polyurethane hydrogel encapsulating NSCs enhance proliferation of encapsulated cells (Hsieh et al., 2015) Copyright 2015, Elsevier; (C) Soft (< 1 kPa) 2D methacrylamide chitosan hydrogel on glass, preferentially support neuronal differentiation of NSPCs during 8 days of culture (Leipzig and Shoichet, 2009) Copyright 2009, Elsevier; (D) Soft (0.5 kPa) 3D hyaluronic acid hydrogels trigger neurite extension from hiPSC-NPC spheroids, while stiff (1.4 kPa) hydrogel failed to support neurite extension, after 28 days of culture (Wu et al., 2017) Copyright 2017, Royal Society of Chemistry.
Topography and alignment also influence neuronal cell proliferation. Electrospun gelatin nanofiber meshes with randomly oriented nanofibers showed enhanced adhesion (20%) and proliferation of Schwann (RT4-D6P2T) (30%) and sensory neuron-like (50B11) (40%) cell lines, while aligned fibers enhanced the differentiation (Gnavi et al., 2015). Similarly stimuli responsive biomaterials also influence cell proliferation. Electrically conducting aligned PLLA and polypyrrole electrospun nanofibers coated with PO support proliferation (40%) and differentiation of PC-12 cells while on electric stimulation it improves the neurite out growth (Tian et al., 2016). Table 1 presents an overview of biomaterials reported to support cell proliferation.
Biomaterial Designs to Support Differentiation of Stem Cells Into Neuronal Cell Lineages
The success of nerve regeneration therapies is based on optimized differentiation of stem cells into different nerve cell lineage. The undifferentiated multipotent NSCs can differentiate into unipotent neurons, astrocytes, and oligodendrocytes lineage. The following section describes biomaterials to guide differentiation of stem cells into neural cells.
Several adhesive proteins and peptidomimetics have been reported to support neuronal differentiation on different biomaterials. Micropatterned FN/N-cadherin on PDMS substrates allows formation of cell-cell and cell-matrix contacts and stimulate differentiation of NSCs into neural linage (Wang et al., 2014). LN coated graphene films preferential enhance neuronal differentiation of hNSCs during 2–4 weeks culture (Park et al., 2011). Bioactive IKVAV peptide functionalized phospholipid bilayers supported differentiation of embryonic NSCs into neuronal lineage over the glial phenotype (Thid et al., 2008). YIGSR modified aligned PLLA-DIBO nanofibers prepared by metal-free click chemistry promoted neurogenic differentiation of mouse ESCs (Callahan et al., 2013). Gelatin coated PCL electrospun fibers in combination with retinoic acid were reported to trigger differentiation of embryonic stem cells (ESCs) into neural progenitors (Xie et al., 2009). Hybrid scaffolds such as silk-carbon nanotube scaffold coated with PO promoted ~1 fold increase in neuronal differentiation of human ESCs compared to bare silk fibers (Chen et al., 2012). Similarly, silk fibroin films decorated with integrin-binding LN peptide motifs (YIGSR and GYIGSR) triggered differentiation of human Mesenchymal stem cells (MSCs) into neurogenic cells (Manchineella et al., 2016). Growth factors such as ciliary neurotrophic factor (CNTF) pattern printed on P(AAm) hydrogels promoted astrogenic differentiation of MSCs at the printed areas, while cells remained undifferentiated on fibroblast growth factor-2 (FGF2) printed area (Ilkhanizadeh et al., 2007). MSCs cultured on gold surfaces modified with FN, RGD (cyclic and linear form), or KRDGVC ligands developed into different phenotypes. Neurogenesis was observed at low surface concentration of linear RGD ligand, and myogenesis when cultured on high concentrations of linear RGD. The rest of adhesive ligands promoted osteogenesis (Kilian and Mrksich, 2012).
Biofunctionalization of biomaterials for 3D cultures with adhesive ligands also influences neural differentiation. Aligned electrospun cyclodextrin nanofibers conjugated with admantane-IKVAV increased neuronal differentiation (~1 fold) and oriented neurite extension (Hamsici et al., 2017). IKVAV modified hyaluronic acid–PEG hydrogel supported neural differentiation, neurite outgrowth and growth of long axons (Xing et al., 2017). Recombinant 3D spider silk (4RepCT) matrices enhance differentiation of NSCs isolated from the cerebral cortices into neurons (Lewicka et al., 2012). Electrospun PLC fibers coated with GO result in PCL-GO hybrid nanofibers. NSCs undergo differentiation into neuronal lineage at low GO concentration, while into oligodendrocyte lineage at high GO concentration. The authors predict that differentiation is based on interaction of cells with material, influenced by high elasticity and flexibility of GO (Shah et al., 2014). Soft 3D hydrogels formed by self-assembling of RADA16 sequence containing RGD support differentiation of adult mouse NSCs into neurons (Gelain et al., 2006). RADA16 based SAPs functionalized with RGD or LN derived BMHP-1 and BMHP-2 were used for 3D cell culture of adult mouse NSCs. The RADA16-RGD promoted cell differentiation while RADA16-BMHP supported proliferation (Cunha et al., 2011). DNA nanotubes functionalized with RGD also supported differentiation of NSCs into neurons (Stephanopoulos et al., 2014). NSCs cultured on 3D porous graphene foam (3D-GFs) functionalized with LN supported cell differentiation into neurons and astrocytes (Nasir et al., 2013). Super porous 2-hydroxyethyl methacrylate with 2-aminoethyl methacrylate scaffolds modified with IKVAV support cell adhesion and the differentiation of human fetal NSCs into neurons (Kubinová et al., 2010). IKVAV grafted 3D silk fibroin-based hydrogel promote differentiation of progenitor cells into neuronal cells (Sun et al., 2017). Alginate hydrogels biochemically conjugated with Fc-tagged recombinant N-cadherin (N-Cad-Fc) protein promoted a ~80% increased neurogenic differentiation of neural cortical cells of rat embryo (E18) compared to unmodified hydrogel (Lee et al., 2016). IKVAV modified self-assembled 3D PAs nanofibers, preferentially promotes neuronal cell growth over glial cells (Silva et al., 2004). Rat embryonic NPCs embedded in 3D graphene oxide (GO) scaffold coated with PL developed interconnected synapsis and differentiated into neurons (~62%) and astrocytes (~41%) during 14 days of cell culture (Serrano et al., 2014). The differentiation of neural precursor cells in 3D collagen gels modified with PO/LN, growth factor-reduced matrigel (gfrMG) or PuraMatrix® gels depicted enhanced (~2 folds) neurogenic differentiation in gfrMG modified matrices (Uemura et al., 2010). IKVAV functionalized 3D collagen hydrogels enhanced (~5 folds) differentiation of dorsal root ganglions (DRGs) into neuronal phenotype in comparison to unmodified collagen matrix (Hosseinkhani et al., 2013). Hybrid 3D matrices formed by collagen and PAs nanofibers modified with IKVAV were reported to support survival and dendritic growth of purkinje neurons (PC) (Sur et al., 2014). High PA-IKVAV to collagen ratio (1:0.45) promoted dendritic growth (~4 folds) and axonal guidance of PC neurons (Sur et al., 2014). Gradient of IKVAV on photochemically modified PCL fibers supports neuronal differentiation and expression of β-III-tubulin in PC12 cells, and neurite growth in the direction of increasing peptide concentration (Kim et al., 2015).
The stiffness of the biomaterial also plays an important role in defining cell phenotype. MSCs cultured on soft P(AAm) gels (i.e., Young's Modulus of 0.1- 1 kPa, mimicking the stiffness of brain tissue) developed into neurogenic cells, while the culture conditions rendered myocytes or osteogenic phenotypes when harder gels were used for culture (8–17 or 25–40 kPa respectively).(Engler et al., 2006) These findings have been supported by other reports as well (Wen et al., 2014). ESCs differentiation was studied on covalently crosslinked gelatin gels with Young's Modulus ranging from 2 to 35 kPa. Soft 2 kPa substrate preferentially supported differentiation of ESCs into mature neurons, while a 83% decrease in neuronal differentiation was reported on gelatin of higher (35 kPa) stiffness (Ali et al., 2015). Neuronal differentiation of mouse embryonic NPCs on P(AAm) hydrogels (0.2–20 kPa) covalently functionalized with IKVAV and PL showed a 6 folds enhancement of NPCs neuronal maturation on 2 kPa gels vs. IKVAV/PL coated glass, while the 20 kPa hydrogel enhanced (30% increase) neuronal differentiation of adult neural stem cells (aNSCs) (Farrukh et al., 2017a). Methacrylamide chitosan (MAC) hydrogels (1–10 kPA) functionalized with LN showed the highest neuronal differentiation of NSPCs at < 1 kPa (30%), low density of astrocytes (2%) between 1 and 3.5 kPa, and oligodendrocyte differentiation (70%) on stiff 7 kPa hydrogel (Figure 3C) (Leipzig and Shoichet, 2009).
3D cultures of hyaluronic acid hydrogels with tunable stiffness (1.5–7 kPa) coated with PL showed differentiation of NPCs into neurons at low stiffness (1.5 kPa) and into astrocytes at higher stiffness (7 kPa) (Seidlits et al., 2010). mNSCs encapsulated in soft elastin-like proteins (ELP) hydrogel modified with RGD (G′ = 0.36 kPa) showed enhanced viability ~97% and differentiation into neurons (Madl et al., 2016). ELPs without RGD sequence or scramble RDG sequence failed to support cell survival or differentiation. RGD functionalized 3D hyaluronic acid hydrogel with different stiffness (G′ 400 and 800 Pa) is reported for culture of hippocampal NPCs. Neurite extend through the hydrogel with enhanced neurite outgrowth and branching on soft hydrogel (G′ 400 Pa) (Tarus et al., 2016). Hydrogel films of 3-hydroxybutyrate and 3-hydroxyhexanoate copolymers promoted neuronal differentiation of NSCs during 2D cell culture. The same polymers used as 3D matrices supported attachment, synaptic outgrowth and synaptogenesis (Xu et al., 2010). Chitosan 2D films promoted astrocytic differentiation of NSCs, while chitosan porous scaffolds and chitosan multimicrotubule conduits supported neuronal differentiation (Wang et al., 2010a). Methacrylated hyaluronic acid (0.5–1.5 kPa) 3D hydrogels promoted differentiation of human induced pluripotent stem (hiPSCs) derived NPCs and Down syndrome patient-specific hiPSCs derived NPCs spheroids into neurons (Figure 3D). Soft (0.5 kPa) hydrogels enhanced neuronal phenotype (~1 fold), suppressed (~40%) astrocytic differentiation and triggered neurite outgrowth (Wu et al., 2017). RGD functionalized 3D ELP based polymeric hydrogels (0.5–2.1 kPa) enhanced DRGs neuronal viability and promote neurite outgrowth from DRGs explant. Soft 0.5 kPa ELPs-RGD promoted neurite extension up to ~1,800 μm from DRS during 7 days of cell culture, in contrast to ~500 μm neurite extension on 2.1 kPa hydrogel (Lampe et al., 2013). Gelatin-hydroxyphenylpropionic acid hydrogels with tunable stiffness (G′ 0.28–0.84 kPa) modulated differentiation of hMSCs. Low stiffness (0.28 kPa) hydrogels promoted neurogenesis, while high stiffness (0.84 kPa) increased cell proliferation (Wang et al., 2010b). Thixotropic 3D PEG-silica hydrogel with 7, 25, and 75 Pa stiffness functionalized with RGD promoted neuronal differentiation of MSCs only above 75 Pa. Very soft hydrogels failed to support cell viability, proliferation and differentiation of cells (Pek et al., 2010).
Several reports demonstrate the effect of geometry and topography of substrates on cell differentiation. Rat hippocampus-derived adult NSCs on laminin-coated electrospun polyethersulfone fiber with different fiber diameter (~ 250–1,500 nm) showed a 40% increase in oligodendrocyte differentiate on small diameter mesh (280 nm), while a 20% increase in neuronal differentiation was observed on meshes with diameter 750 nm. Authors attribute this effect to random spreading of cells on densely packed small fiber mesh vs. aligned extension of cell along single fiber of large diameter (Christopherson et al., 2009). PCL loop mesh, bimodal, and biaxially aligned electrospun scaffold (fiber diameter ~40–85 μm) promoted neuronal differentiation and guided the neurite outgrowth of human iPSCs along the fiber, as depicted in Figure 4A. Biaxial aligned scaffolds promoted the highest viability (95%) and neurite extension along the fibers (Mohtaram et al., 2015). Micro-patterned PDMS with PL and LN stripes directed differentiation and guidance of adult human stem cells. Microstripes significantly wider than the cell soma (3 μm) promoted neural differentiation, while stripes narrower than 10 μm hindered differentiation (Figure 4B) (Béduer et al., 2012). LN functionalized P(AAm) hydrogels (0.6 kPa) with un-patterned or with circular (50 μm) geometrical patterns, trigger preferential differentiation of MSCs into neurogenic cells (90%) on un-patterned substrate while adipogenic cells (60%) on circular geometry (Lee et al., 2013).
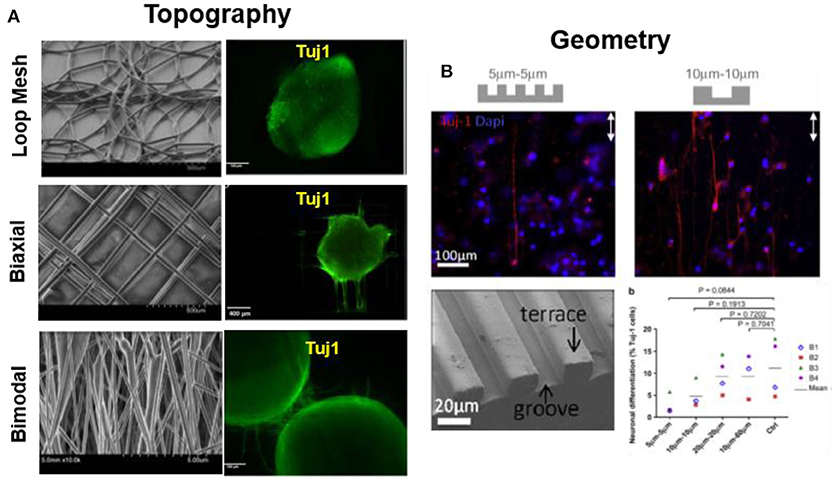
Figure 4. Substrate topography modulates cell differentiation. (A) PCL loop mesh, bimodal and biaxially aligned electrospun scaffold (fiber diameter ~40–85 μm) influence on differentiation of iPSCs. Biaxially aligned fibers preferentially promoted the highest neurite extension as labeled by Tuj1 immunostaining (Mohtaram et al., 2015) Copyright 2015, John Wiley and Sons; (B) Micro-patterned PDMS with PL and LN stripes directed differentiation and guidance of adult hNSCs. Microstripes >10 μm promote neuronal differentiation, while < 10 μm hinder differentiation, during 7 days of culture (B1-B4 represents individuals with 4 different biopsies) (Béduer et al., 2012) Copyright 2012, Elsevier.
Biomaterials Supporting Neuronal Migration
Regenerative strategies for neural tissue involve the recruitment and instruction of endogenous neural stem cells or Schwann cells by using scaffolds containing relevant features of the migratory environment in brain tissue and assist cells to organize and relocate at appropriate positions. Similar to neural attachment, neural migration is influenced by biochemical (adhesive ligands, growth factors), mechanical factors and topographical of the extracellular matrix, and also by adhesive contacts to neighboring cells. Examples of reported migratory responses of neural cells to these material parameters are detailed below.
The type and concentration of adhesive ligand on a biomaterial influences migration of neural cells by stabilizing the attachment of the growth cone of neurites. LN, for example, has been demonstrated to stimulate and guide migration of olfactory epithelial neurons in vitro (Calof and Lander, 1991). Depending on the cell type, different responses to the same adhesive coating can be expected. For example, aNSCs and astrocytes migrated on PL surfaces and became less migratory on PL/LN mixtures, while neuron preferred to spread and not move on PL (Joo et al., 2015a,b). A recent article describes morphological features on the migration of neuronal cells on different protein coatings FN, LN, LN mimetic peptides, reelin etc. Using micropatterned substrates with contrasting regions with different proteins, the role of specific adhesive cues in triggering, guide or stop migration of Early postmitotic cortical neurons on a biomaterial surface was explored (Zhao et al., 2017). In particular the role of adhesion for terminal somal translocation, i.e., the specific migratory behavior of cortical neurons when they position in the cortex layers, was studied. Somal translocation could be efficiently triggered when the growth cone of a neurite spread and stabilized on an area of stronger adhesive interactions, for example with a higher concentration of adhesive molecules (Figure 5A). In vivo, LN coated scaffolds promote migration of neuroblasts to injured brain tissue, contributing to neuronal regeneration after stroke in mice (Figure 5B) (Fujioka et al., 2017).
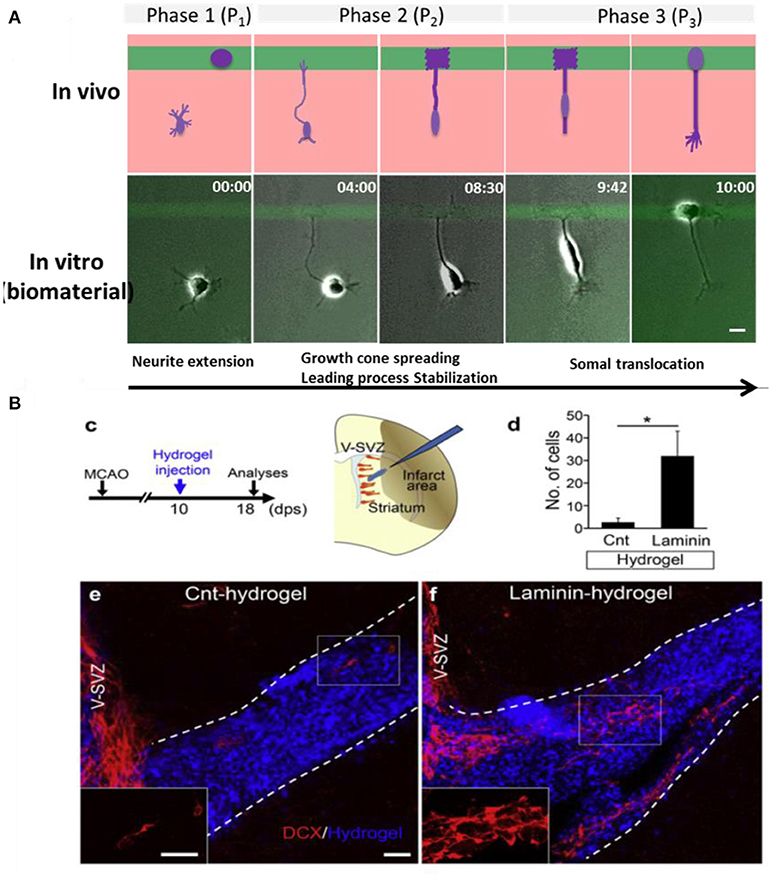
Figure 5. Neuronal migration on biomaterials. (A) Hydrogel coated glass slides modified with mircopatterens of PL/IKVAV to trigger neuronal migration and somal translocation of cortical neurons in vitro (Zhao et al., 2017) Copyright 2017, Elsevier; (B) Migration of neuroblasts along a LN coated hydrogels toward the injured area in vivo (Fujioka et al., 2017) Copyright 2017, Elsevier.
Gradients of soluble neurotrophic factors and neurotransmitters influence neuronal migration (Li Jeon et al., 2002). These molecules can be added to cell cultures, or secreted by other co-cultured cells like astrocytes (Mason et al., 2001). Glial and neural migration through hydrogels was demonstrated to be enhanced through delivery of soluble growth factors such as nerve growth factor (NGF) from fibrin in in vitro cultures (Wood and Sakiyama-Elbert, 2008). In vivo, the delivery of stimulating molecules to the CNS represents a clinical challenge because the blood–brain barrier limits the diffusion of molecules into the brain by traditional oral or intravenous routes. Injectable hydrogels have the capacity to overcome the challenges associated with drug delivery to the CNS (Pakulska et al., 2012). Intraventricular sequential delivery of epidermal growth factor (EGF) and erythropoietin (EPO) into a stroke injured rat brain showed enhanced migration of endogenous NSPCs to the injury site, resulting in neurogenesis and improved functional recovery (Kolb et al., 2007).
During growth and migration cells sense and can be guided by the variation of mechanical properties of their microenvironment. The relevance of mechanical signaling in different contexts of cell function is a current vivid area of research, also related to neural tissue. Stiffness gradients have been reported in CNS tissue (Franze, 2011, 2013; Wrobel and Sundararaghavan, 2013). LN-coated P(AAm) hydrogel with high stiffness (20 kPa) greatly promoted the migration of Schwann cells progenitors from embryonic DRGs compared to low stiffness hydrogel (1 kPa)(Rosso et al., 2017).
During development, migration of neuronal cells often occurs in a directional way. Neurons attach and migrate along fibers of the ECM or along glial cell tracts (Malatesta et al., 2008). The sensitivity of neurons to topographical features has been exploited in vitro and in vivo (Hoffman-Kim et al., 2010; Gumera et al., 2011). Oligodendrocytes (Webb et al., 1995) and neurons (Gomez et al., 2007), have been shown to migrate along grooved topographies. The average migration speed of cells was higher on microgrooved substrates than on flat surfaces (Nikkhah et al., 2012). In 3D environments, DRGs cells exhibited unidirectional migration into micro-channels of the PEGylated fibrinogen hydrogel (Sarig-Nadir et al., 2009).
Neuronal migration has been also modulated via cell-cell contacts, specifically involving glial cells in cocultures. The membrane proteins connexin 46 and 23 expressed by radial glia (Valiente and Marín, 2010) and the cell adhesive proteins L1-CAM and neural cell adhesion molecule (NCAM) (Schmid and Maness, 2008) seem to play a relevant role in neuronal migration.
Biomaterials for Directional Neurite Extension
In order to achieve successful regeneration of nerve tissue, sprouting axons from the proximal stump of one neuron need to grow and establish a new connection with the distal stump of the next neuron (Shin et al., 2003). Following injury, the remaining functional neurons will try to grow processes and reestablish connections with neighboring partners, but they often meet an impenetrable scar tissue composed of myelin, cellular debris, and other cells (astrocytes, oligodendrocytes, microglia) at the injury site. The scar tissue blocks existing neurons from reaching their synaptic target and hinder the regeneration process (Schmidt and Leach, 2003). Guidance in neurite growth plays a vital role in nerve repair. Many approaches to support nerve regeneration, therefore, have focused on the development of biomaterials that provide guidance cues for directional neurite growth.
Topographical Cues for Guiding Neurite Extension
The topography of the neuronal microenvironment, including fibrillar ECM proteins and elongated glial cells, plays a major role for the directional growth of neurites. In the biomaterials field nerve guidance channels, surface topographies and 3D fibrillar meshworks have been used as supportive scaffolds for directional neural regeneration.
Nerve Guidance Channels (NGCs) for Peripheral Nerve Regeneration
Nerve guidance channels (NGCs) are tubular constructs with a hollow lumen through which the neuron axon should grow. This geometry has several advantages for spatial guidance of peripheral nerve regeneration: protection of the regenerating nerve against compression by the surrounding tissue, isolation of the regenerating axons from surrounding tissue, and longitudinal directional guidance of the regenerating neurites toward target tissue. Hollow nerve conduits have been widely used in research and clinical applications. Porous and not porous NGCs providing longitudinally oriented grooves in their lumen surface, (Göpferich, 1996) and eventually functionalized with cell adhesive ligands (e.g., LN-derived peptides YIGSR and IKVAV (Chiono et al., 2009) or controlled released growth factor (neurotrophic factors (Pfister et al., 2007) promote directional axon growth in vivo in small animals test. However, in some cases dispersion of the regenerating axons through the comparatively large lumen of the NGCs leads to inappropriate target reinnervation or polyinnervation of different targets by the axonal branches of the same neuron. Single hollow lumen NGCs are thus only recommended for small lesions (< 30 mm) in the sensory nerves (Weber et al., 2000). In vitro neuronal, Schwann and DRGs culture were used to test the NGCs and in vivo a thy-1-YFP-H mouse common fibular nerve injury model or a nerve gap in the rat sciatic nerve were normally used. Generally, typical NGCs dimensions for experimental use in small animals are inner diameters of 1–2 mm and lengths of several millimeters, depending on the experimental gap.
Considerable effort has been focused on the development of more effective NGCs, in which a microstructured lumen of the NGC provides higher directionality. Structured lumens including multichannels, porous matrices or oriented fibrous conduits have been proposed (Figure 6). Multichannel NGCs mimic the natural compartment structure of nerves (He et al., 2009; Chiono and Tonda-Turo, 2015). They reduce axon dispersion, offer higher surface area for functionalization, cell adhesion and migration as compared to single lumen NGCs. The disadvantages of the multichannel NGC design reduced permeability and mechanical flexibility. In fact, multichannel NGCs did not lead to significant functional improvement in the repair of a 1-cm nerve gap in the rat sciatic nerve compared to single lumen nerve tubes (de Ruiter et al., 2008). NGCs might also incorporate fillers to form an internal porous or aligned 3D matrix. Fillers may include longitudinally aligned fibers (Matsumoto et al., 2000; Wang et al., 2005), porous sponges (Tonda-Turo et al., 2011) or gels (Ceballos et al., 1999; Nakayama et al., 2007). Fillers can also be functionalized with specific peptides/proteins or growth factors, as described in recent reviews (Pfister et al., 2007; Gu et al., 2011). Filling of the lumen of silicon NGCs with longitudinally oriented polyamide filaments lead to improved nerve regeneration by bridging a 15-mm sciatic nerve gap in rats (Lundborg et al., 1997). The ability of tubular channels mininally supplemented with aligned nanofiber-based thin-films to promote regeneration across a 14 mm tibial nerve gap was studied(Clements et al., 2009). They evaluated two different channels: a 1-film guidance channel–containing a single continuous thin-film of aligned fibers, and a 3-film channel. Interestingly, they found that the 1-film channels supported enhanced regeneration compared to the 3-film channels, because the two additional thin-film reduced permeability. Recently, the hollow chitosan nerve guides (CNGs) enhanced by introduction of a longitudinal chitosan film to reconstruct critical length 15 mm sciatic nerve defects in rats(Meyer et al., 2016). Compare to simple hollow CNGs, the CNGs with the introduced chitosan film significantly improved nerve regeneration, almost reached the regeneration outcome after autologous nerve grafting.
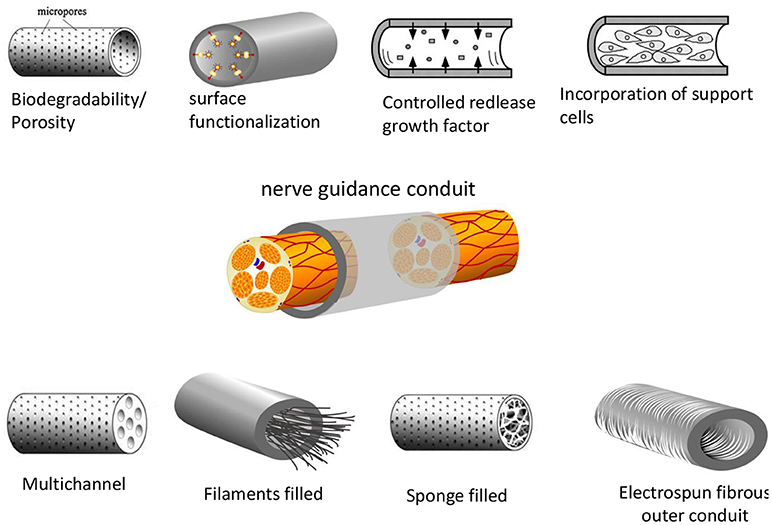
Figure 6. Different designs of nerve guidance conduits (NGCs). The basic design parameters include: a biodegradable and porous channel wall, a biofunctionalized wall surface including adhesive and growth factors, intraluminal structures to mimic the structure of nerve fascicles, intraluminal guidance structures (filaments, sponge or hydrogel-like) or wall microstructures to support cell migration and axonal growth. Support cells can also be incorporated to the NGCs (Daly et al., 2012) Copyright 2012, The Royal Society.
An alternative strategy to guide neurites growth within the luminal cavity of the NGC involves the use of electrospun tube walls. The use of tubes with walls consisting of oriented fibers has a number of advantages over the filled lumen strategy. (i) The materials are highly flexible and porous, well adapted for use within biological systems; (ii) nano- and micro-scale fibers have a high surface area-to-volume ratio increasing the area available for protein absorption, neural cells migration and regeneration of axons; (iii) fibers that can be preferentially aligned resulting in increased promotion of guided axonal growth (Daly et al., 2012).
Scaffolds for Guided Central Nerve Regeneration
Although regeneration of the mammalian CNS was thought to be impossible, studies have shown that axonal growth after spinal cord injury can occur when neurons are provided with the suitable substrata that support directional growth (Fawcett, 1998, 2002). Natural, ECM-derived biomaterials and also synthetic polymers processed in different ways to generate microtopographies have been used as matrices for supporting spinal nerve regeneration.
The relationship between microscale topography and neuronal development has been recently investigated in vitro in a high-throughput screening assay (Li et al., 2015). Primary neurons were presented to patterned substrates with a large library of topographical features including isotropic (e.g., dots, grids, squares) and anisotropic pattern designs (e.g., gratings) with lateral width between 5 and 15 μm and 1 μm depth. Anisotropic topographies enhanced axonal and in some cases dendritic extension vs. isotropic ones. However, dendritic branching occurred preferentially on planar substrates. The depth of the topographical features also influences the growth of processes. Murine NPCs sensed the depth of micro-gratings and neurite elongation, alignment and neuronal differentiation increased with grating depth (Chua et al., 2014).
In vivo studies using poly(2-hydroxyethyl methacrylate-co-methylmethacrylate) hydrogel channels demonstrated improved tissue regeneration of transected rat spinal cords (Tsai et al., 2004). The hydrogel guidance channels were designed to match the dimensions and modulus of the rat spinal cord; the outside diameter of the channels was approximately 4.2 mm, the inside diameter was 3.6 mm, giving a wall thickness of 0.3 mm and the length was 6 mm. By inserting the transected cord stumps into the hydrogel nerve guidance channels, alignment of the cord stumps occurs, and cells were able to migrate along them. Axonal regeneration was enabled, and no significant scar formation appears.
Guided Neurite Extension on 3D Fibrillar Meshworks
Fibrillar 3D matrices can serve as substrates for neuronal growth. The fibrils provide spatial guidance to the extension of processes, while retaining an open matrix structure to be repopulated by the growing cells (Lietz et al., 2006; Schnell et al., 2007). This is of particular interest in the development of biomaterial-based scaffolds intended to promote the repair of highly organized nerve tissues, such as the retina or white matter tracts of the spinal cord.
Protein based and synthetic polymer fibers have been used to form fibrillar matrices and guide axonal growth. Fibers can be processed by different technologies, like electrospinning, bioprinting or self-assembly. Among these methods, electrospinning offers an uncomplicated and low-cost method for processing and applicable to different kinds of materials. Electrospun membranes with randomly or aligned fibers can be produced, and neuronal growth along the fibers has been demonstrated (Sell et al., 2007). NPCs and DRGs cells grew preferentially along aligned PLLA electrospun scaffolds with fiber diameters between 150 to 3,000 nm independently of the adhesive coating (Yang et al., 2005; Corey et al., 2007). NSCs elongated and outgrew neurites along aligned fiber scaffolds without adhesive coating (Figure 7A) (Yang et al., 2005). Authors could not establish a significant effect of the fiber diameter (between 300 and 1,500 nm) on the cell orientation. NSCs differentiation rate was found to be higher on PLLA nanofibers (diameter 300 nm) than that of micro fibers (diameter 1,500 nm), independently of alignment. Aligned nanofibers significantly improved neurite outgrowth compared to not aligned ones. On thicker fibers and fibers coated with adhesive factors, however, different tendencies were observed. Fibers of 35 μm coated with PL and LN promoted directional neurite outgrowth and promoted greater oriented process growth than large-caliber fibers (500 μm) (Smeal et al., 2005; Smeal and Tresco, 2008). Many studies have demonstrated that the aligned nanofibers, pattern nanofibers (half random and half aligned) and also cross-patterned nanofiber can guide the neurites to extend along the nanostructure. However, the contact cues provided by the nanofibers can be far more complicated than just guiding the neurites to extend along them. Xie et al. demonstrated that the neurites could not only project along the nanofibers, but also be directed to grow along a direction perpendicular to the aligned nanofibers. The DRGs neurites grew perpendicularly to the alignment direction of electrospun PCL fibers (Figure 7B) (Xie et al., 2014). The growing direction of neurite on fibers was dependent on the adhesive interaction between neurites and nanofibers and on the dimensions and separation between fibers. A strong interaction leads to parallel growth of neurites along the fibers (e.g., low density fiber and fiber with LN coating), while a weak interaction (i.e., fibers without adhesive proteins) lead to perpendicular growth while high density mesh works lead also to perpendicular growth.
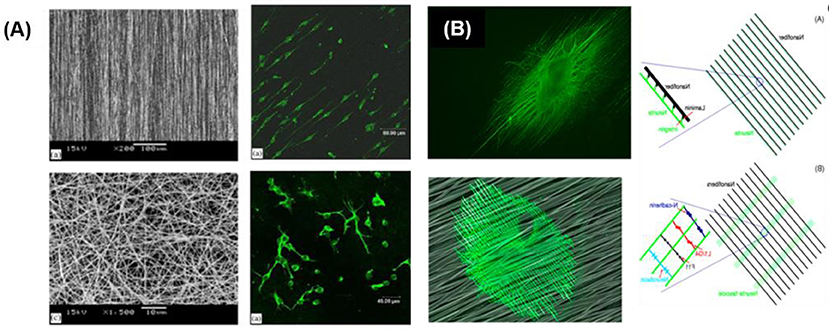
Figure 7. Guided neurite extension on fibrillar networks (A) Neuron cells cultured on random and aligned fibers (Yang et al., 2005) Copyright 2005, Elsevier; (B) Neurites oriented along the nanofibers previously coated with laminin and perpendicularly to the nanofibers without adhesive coating (Xie et al., 2014) Copyright 2014, American Chemical Society.
In vivo, aligned oriented fibers elicit regeneration, while randomly distributed fibers do not, demonstrating how topographical cues can influence endogenous nerve repair mechanisms in the absence of exogenous growth promoting proteins (Kim et al., 2008). Using electrospinning method, poly(acrylonitrile-co-methylacrylate) (PAN-MA) fibrillar constructs (19 mm long and 1.5 mm inner diameter) were produced. Axons regenerated across a 17 mm nerve gap, re-innervated muscles, and reformed neuromuscular junctions. Electrophysiological and behavioral analyses revealed that aligned but not randomly oriented constructs facilitated both sensory and motor nerve regeneration, improving significantly functional outcomes.
Fibers have been also integrated within hydrogel materials to provide hybrid three-dimensional construct for neuronal guidance within a growth promoting environment (Newman et al., 2006; Novikova et al., 2008). Studies showed that magnetic collagen fibers in collagen gels, aligned using magnetic fields, provide an improved template for neurite extension compared to randomly oriented collagen fibers (Ceballos et al., 1999; Dubey et al., 1999). Recently, magnetoresponsive PEG based microgel are reported by incorporation of iron oxide nanoparticles for directional growth of DRGs (Rose et al., 2017). Natural protein based hydrogels also provide adhesive factors to support attachment and neurite grown. Yao et al. developed a hierarchically aligned fibrillar fibrin hydrogel (AFG) with low rigidity and aligned topography to mimics both the soft and oriented features of nerve tissue. They found that the AFG exhibit co-effects on promoting the neurogenic differentiation of human umbilical cord mesenchymal stem cells (hUMSCs) in comparison to random fibrin hydrogel (RFG) and tissue culture plate (TCP). Also, AFG induces DRGs neurons to rapidly project numerous long neurite outgrowths longitudinally along the AFG fibers (Yao et al., 2016).
Recently, Johnson et al. have developed a novel 3D printing approach for manufacturing of a custom nerve repair technology which is personalized to anatomical geometries, and augmented with physical (microgrooves) and biochemical cues (multicomponent diffusive biomolecular gradients) to promote the regeneration of multiple nerve pathways (Johnson et al., 2015). The custom scaffolds are prepared via a 3D printing using 3D models, which are reverse engineered from patient anatomies by 3D scanning. The bifurcating pathways (sensory and motor path) are augmented with microgrooves and path-specific biochemical cues for the regeneration of complex mixed nerve injuries (Figure 8). This 3D printed scaffold provides axonal guidance in vitro and achieved successful regeneration of bifurcated injuries across a 10 mm complex nerve gap in rats in vivo.
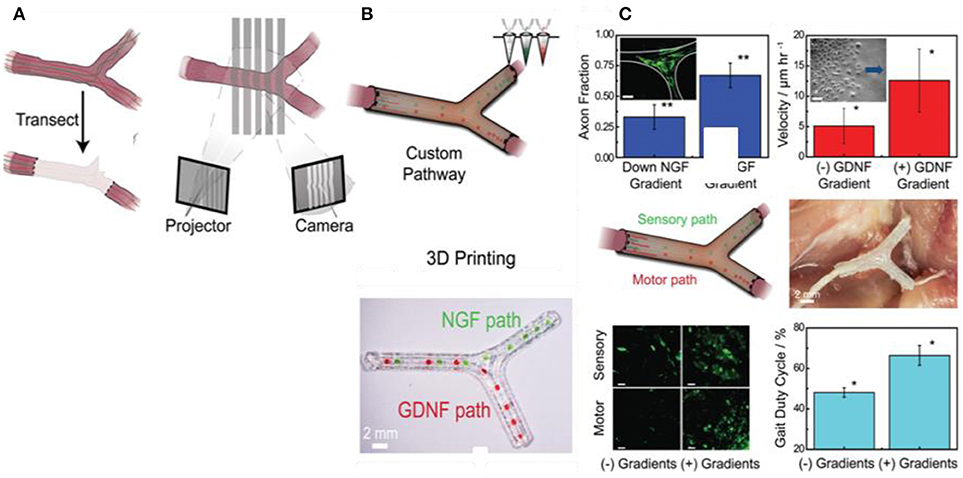
Figure 8. Guided neurite extension in 3D printed scaffold; (A) Nerve regeneration pathways enabled by 3D scanning and printing, (B) The 3D printing process provided the ability to introduce advantageous physical and biochemical cues in the form of microgrooves and multicomponent diffusive biomolecular gradients, (C) In vitro and in vivo characterization of regeneration with 3D printed nerve pathways (Johnson et al., 2015), Copyright 2015, John Wiley and Sons.
Synthetic hydrogels formed by self-assembling PAs show also a nanofibrilar structure that has been used in nerve regeneration. Encapsulated NPCs were observed to differentiate into neurons with extensive neurite outgrowth within nanofibrillar hydrogels (Silva et al., 2004).
Guided Neurite Growth by Rigidity Patterns
The rigidity of the biomaterial contributes to the oriented growth of neurites in spiral ganglion neurons (SGN) on micropatterns. Alignment was significantly enhanced when the material stiffness increased from 650 to 2,000 MPa (Tuft et al., 2014). Increasing substrate stiffness of a LN-coated P(AAm) hydrogel also promoted directional neurite outgrowth from embryonic DRGs (Rosso et al., 2017). The neurite in low stiffness substrate (1 kPa) show relax and less aligned morphology, whereas the neurite display stretch, more aligned morphology in high stiffness (20 kPa). Interestingly, the opposite observation was made in 3D cultures. A hyaluronic acid (HA) hydrogel with tunable Young's Modulus between 400 and 800 Pa was used to culture hippocampal neural progenitor cells (HNPCs) (Tarus et al., 2016). Neurites of HNPCs grew into the soft HA hydrogel at increased outgrowth and density. The growth of neurites (in quantity and length) from DRGs was also promoted in softer (0.5 kPa) elastin-like hydrogels (Lampe et al., 2013). Authors hypothesize that on 2D environments the stiffer substrates provide more stable anchoring to facilitate the outgrowth of neurite. In contrast, stiffer 3D matrices (i.e., higher crosslinking degree) hinder the outgrowth of neurites due to the small pore sizes.
Neural Growth on Patterns of Cell Adhesive Ligands
Patterns of adhesive ligands (full proteins or peptidomimetics) on non-adhesive backgrounds (typically PEG) can be used to selectively promote neuronal attachment and guided outgrowth on the adhesive regions of the pattern (Zhang et al., 2005; Straley et al., 2010; Joo et al., 2015a). Recent studies demonstrate that responsive biomaterials can be used to in situ guide axonal growth. In vivo, poly(vinyl chloride) (PVC) channels filled with different adhesive matrices (a YIGSR peptide containing agarose gel, a plain gel, and PBS solution) have been applied to fill a 4 mm segment of dissected dorsal root. A significant increase of myelinated axons was shown in the peptide modified agarose gel (Borkenhagen et al., 1998).
In addition to promoting cell growth, the presentation of neurotrophic factors in a gradient distribution within scaffold has also been studied for guidance of regenerating neurons. Several in vitro studies have demonstrated that neuronal cells are guided by immobilized gradients of nerve growth factors or neurotrophic factors on scaffolds (Moore et al., 2006; Dodla and Bellamkonda, 2008). The presence of LN and NGF gradients in agarose scaffolds has also shown better functional recovery of long peripheral nerve gaps than uniform concentration scaffolds (Dodla and Bellamkonda, 2008).
Directed Growth on “Living Scaffolds”
During neural morphogenesis and development, directed axon growth and cell migration typically occurs along pathways formed by other cells. This concept has long been appreciated in developmental neurobiology as crucial to the proper formation of the nervous system, including necessary axonal connectivity and localization of cellular constituents. This idea has also been embraced by the tissue regeneration community and lead to the concept of “living scaffolds” for regeneration. These are tissue engineered constructs containing supporting guiding material and cells from the neural environment, typically glial cells and astrocytes (Figure 9). These follow the haptotactic cues of the scaffold and arrange in oriented dispositions. These cells secrete neural growth factor and combine haptotactic and chemotactig signals to neuronal cells to grow along them (Struzyna et al., 2014).
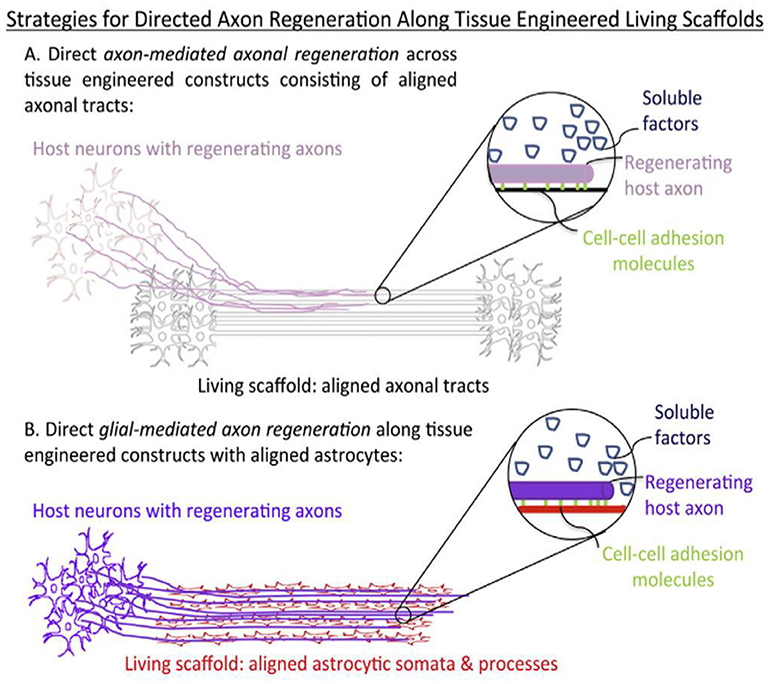
Figure 9. Structural and soluble cues directing axonal outgrowth along “living scaffolds” (Struzyna et al., 2014) Copyright 2014, Elsevier.
In vitro, higher order structures can be formed by first culturing and aligning support cells on microgrooves, followed by seeding of neurons (Nikkhah et al., 2012). Micropatterned PLLA substrates containing grooves selectively coated with LN were used to culture rat Schwann cells to support neurites outgrowth (Miller et al., 2001). Neurons cultured on those substrates displayed accelerated outgrowth of nerve fibers and 98% alignment of neurites along the microgrooves. In a different study, micropatterned Schwann cells controlled by micropatterned LN stipes on glass substrates were used to direct neuronal regeneration (Thompson and Buettner, 2004; Schmalenberg and Uhrich, 2005).
Winter et al. have developed a living scaffold that structurally mimicked the glial tube. It consisted of aligned astrocytes that guided the migration of NPCs and facilitated directed axonal regeneration for CNS repair. The networks of longitudinally aligned astrocytes on patterned hydrogels, supported seeded neurons to extend neurites along the aligned astrocytes bundles (Winter et al., 2016). In a different approach, collagen sheets supported alignment of astrocytes in the presence of transforming growth factor (East et al., 2010). The collagen sheets were then rolled to create cylindrical constructs. Dissociated DRGs neurons and astrocytes were seeded together on the scaffolds. Neurites preferentially grew along the aligned astrocytes.
In vivo, living scaffolds consisting of neurons and stretch-grown axonal tracts were grown to 10 mm in length, encapsulated in collagenous matrices, and transplanted to repair equally sized lateral hemisection spinal cord lesions in rats for spinal cord repair (Iwata et al., 2006). At 1 month post-surgery, the constructs had integrated with the host by extending axons into the spinal cord. Similar constructs containing “stretch-grown” axonal tracts were also used for peripheral nerve repair (East et al., 2010).
Biomaterials Supporting Neural Growth in-vivo
Central nervous system (CNS) injuries emerge from accidents or trauma affecting brain and spinal cord or by neurodegenerative disorders such as Parkinson's or Alzheimer's disease (Daly et al., 2012). Peripheral nervous system (PNS) disorders occurred through cut or injury to nerve cord, effecting autonomic motor and sensory functions resulting in impairment of body performance (Kabu et al., 2015). The common strategies to repair CNS injuries involves grafting of stem cells at injured site, while PNS system repair is frequently based on autograft or hollow nerve guidance conduits. However, after implantation the grafted cells fails to survive, remain undifferentiated or chiefly differentiate into to glial cell forming glial scar and fail to develop oriented nerves. In addition, the rejection of implant due to inflammation and infection at surgery site also affect success of transplant. Biomaterials for neural regeneration are designed to resemble the properties of the natural cellular niche (stiffness, topography), accompanied with tunable release of growth factors and availability of ECM bioactive motifs. This section presents a brief overview of new advances in biomaterial based implants for nervous system regeneration.
Inert biocompatible scaffolds functionalized with bioactive sequences in combination with addition or immobilization of nerve growth factors have been tested for regeneration therapies. Injectable 3D IKVAV containing SAPs (RADA16-IKVAV) hydrogel (G' 300 Pa) was reported for recovery of cerebral neocortex injury in rat brain surgery model. In-situ self-assembly of RADA16-IKVAV hydrogel fill the injury gap, enhanced cell survival and reduced the glial astrocytes differentiation in comparison to bare RADA16 during 6 weeks after post-implantation (Cheng et al., 2013). MAC scaffold functionalized with growth factors and LN modulated the differentiation of subcutaneously implanted NPCs into different lineages. MAC was functionalized with interferon-γ (IFN-γ) for neurons, platelet derived growth factor-AA (PDGF-AA) for oligodendrocytes, or Bone morphogenetic protein-2 (BMP-2) for astrocytes differentiation. Cells differentiation was more effective when the growth factors were conjugated with the scaffold and not added freely to the medium. Differentiation into neuron was significant and rosette like neurons were reported after 28 days of implantation (Li H. et al., 2014). A 3D scaffold of poly(desaminotyrosyl tyrosine ethyl ester carbonate) electrospun fibers with 1.25–3.23 μm diameters was implanted at mouse brain striatum to enhance the cell viability and neuronal differentiation at implantation site. Human induced neuronal cells (h-iN), dispersed in fiber suspension were injected at the site of injury forming a gel in-situ. h-iN inside the hydrogel showed ~38-fold enhanced in vivo cell viability and 3.5-folds improvement in neurite outgrowth in comparison to isolated h-iN (Carlson et al., 2016). Cell viability and outgrowth of spiral ganglion neurites has reported to be enhanced by coupling of IKVAV peptide (~100%) on PuraMatrix® hydrogel in comparison to unmodified hydrogel (~40%) (Frick et al., 2017). IKVAV containing PAs nanofibers employed in in vivo mouse spinal cord injury model enhanced cell viability (~2 folds) at the site of injury and promoted development of motor neurons (Tysseling-Mattiace et al., 2008; Cui et al., 2010; Sun et al., 2017). Alginate-based capillary hydrogels seeded with brain-derived neurotrophic factor (BDNF) expressing bone marrow stromal cells (BMSCs) guided axon extension on lesion site. A 3–4 folds increase in the axon length along the rostro-caudal direction, extending through the whole implant in rat spinal cord was achieved (Figure 10A) (Günther et al., 2015).
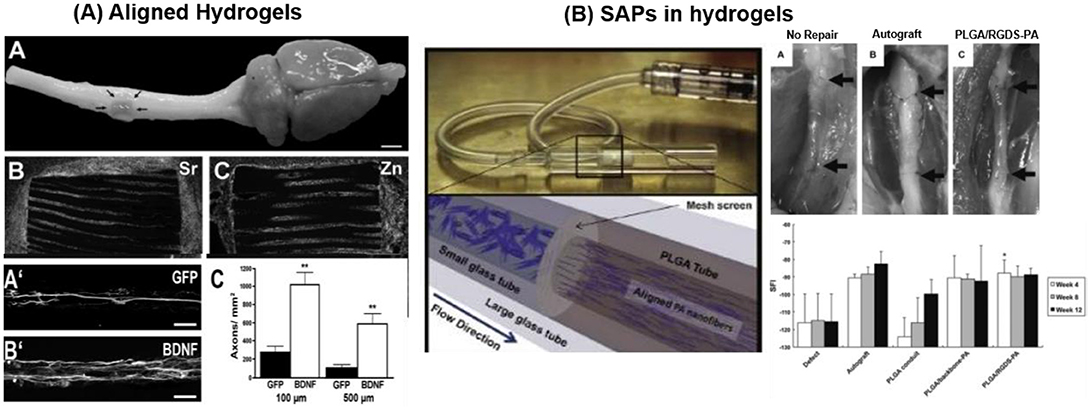
Figure 10. Biomaterials applied for regeneration therapies (A) Alginate hydrogel scaffolds encapsulating BMSC (expressing GFP or BDNF) to promote directed linear axonal regeneration in the injured rat spinal cord model. Cell-filled alginate scaffold implanted at injured area with Sr2+ and Zn2+ ions staining demonstrates numerous cells within the channels. BMSC expressing BDNF cell preferentially promote axonal growth (Günther et al., 2015) Copyright 2015, Elsevier; (B) PLGA based peripheral nerve construct filled with aligned PAs (PAs-RGD and PAs-IKVAV) for recovery of peripheral nerve injury. PAs solution, filled inside PLGA tubes was crosslinked by CaCl2. PAs-RGD filled PLGA construct provide faster recovery than bare PAs and comparable with autograft (Li A. et al., 2014) Copyright 2014, Elsevier.
PLGA conduits filled with aligned PAs (palmitoyl-VVAAEENH2) with and without bioactive RGD or IKVAV epitope were reported to repair rat sciatic nerve injury. Agarose hydrogel loaded with concentration gradient of LN and NGF, promote sciatic nerve repair covering the gap of 20 mm (Dodla and Bellamkonda, 2008). PLGA implant containing bioactive (IKVAV or RGD) PAs grafted at injury site of peripheral nerve critical sized defect model enhanced Schwann cells (~20–40% increase) and axonal growth (~20% increase) during 21 days of implant in comparison to bare PLGA-PAs (Figure 10B). Bioactive PLGA-PAs support recovery of motor and sensory activity after 12 weeks of implantation comparable to autograft (positive control) (Li A. et al., 2014). Commercially available inert PuraMatrix® hydrogel functionalized with IKVAV is applied for cochlear implants (CI). Table 2 entailed biomaterials relevant for neural tissue engineering.
Critical Overview
Strategies for brain repair heavily depend on our ability to temporally reconstruct the natural cellular microenvironment of neural cells. Biomaterials play a fundamental role in this context, as they provide the mechanical support for cells to attach and migrate to the injury site, as well as fundamental signals for differentiation. The increasing evidence that (neural) cells sense and specifically respond to biochemical and physical material parameters like stiffness or morphology opens enormous possibilities for material-supported cell therapies for brain repair. However, reported work up to now, as described in this article, is mostly phenomenological and limits attempts to extract generic material properties-cellular response relationships out of our analysis. The still phenomenological character of most publications does not allow scientifically grounded statements that could lead biomaterials design for nervous tissue regeneration.
Break-through approaches in the field will depend on several factors. From the materials side, the analysis and quantification of the material properties to which cells are exposed is a fundamental requirement. The density of protein or peptide of an adhesive coating depends on the chemistry of the surface and the coating strategy. Same incubation conditions lead to different surface densities on different materials, and this will influence the biological response. No comparison between biological readouts from different articles is possible if there is no quantitative information about the surface composition with which the neuronal cell interacts. The stiffness of a material is typically analyzed as a macroscopic parameter, whereas the cell senses stiffness at a molecular lengthscale. Fibrous or continuous matrices can appear very different to a neuron from a mechanical perspective. Moreover, the Young's Modulus of a material describes only part of the mechanical response, and not necessarily the one a cell might feel long-term. Viscous components might play a role, as demonstrated for other cell types. All these factors have to be properly described in order to make meaningful interpretation of cell responses to biomaterials, and to extract useful information for advanced materials design.
Novel strategies for brain repair will also depend on the ability of biomaterials developers to assimilate and translate increasing knowledge from cell and matrix biology of neural tissue into artificial models. The regenerative biomaterials community is traditionally dominated by material scientists cooperating with surgeons in best case, and it has little interaction with neurobiology or neuronal development community. All these fields have a lot to share with each other, though the languages and experimental methods are very different. Approximation between the different communities is starting and will profoundly impact development in biomaterials for brain tissue repair, as it is impacting in other tissue types. We face a challenging and exciting era.
Experimental work with neuronal cells is challenging. Neurons are difficult to culture and the access to primary cells is more complicated than in other tissue types. The analysis of the existing literature evidences that biomaterials development for brain repair lies behind other tissue types. However, knowledge transfer will occur and will accelerate development in the coming years. There is a long way to go until break-through approaches in brain repair will translate into revolutionary therapies, but there is hope to get there in the next decade.
Author Contributions
All authors listed have made a substantial, direct and intellectual contribution to the work, and approved it for publication.
Funding
SZ acknowledges funding from China Scholarship Council. AdC acknowledges funding from Deutsche Forschungsgemeinschaft (SFB1027).
Conflict of Interest Statement
The authors declare that the research was conducted in the absence of any commercial or financial relationships that could be construed as a potential conflict of interest.
Abbreviations
3D-GFs, 3D porous graphene foam; AFG, Aligned fibrillar fibrin hydrogel; aNSCs, Adult neural stem cells; BDNF, Brain-derived neurotrophic factor; BMHP, Bone marrow homing peptide; BMP, Bone morphogenetic protein; BMSCs, Bone marrow stromal cells; CNGs, Chitosan nerve guides; CNS, Central nervous system; CO, Collagen; DRGs, Dorsal root ganglion; ECM, Extracellular matrix; EGF, Epidermal growth factor; ELP, Elastin-like proteins; EPO, Erythropoietin; ESMN, Embryonic stem cell-derived motor neurons; FGF-2, Fibroblast growth factor 2; FN, Fibronectin; GDNF, Glial cell line-derived neurotrophic factor; GO, Graphene oxide; h-iN, Human induced neuronal cells; hiPSCs, human induced pluripotent stem cells; HNPCs, Hippocampal neural progenitor cells; hNSCs, Human neural stem cells; hUMSCs, human umbilical cord mesenchymal stem cells; LN, Laminin; MAC, Methacrylamide chitosans; MaSp1, Major ampullate spidroin 1; mNSCs, Mouse neural stem cells; MSCs, Mesenchymal stem cells; NCAM, Neural cell adhesion molecule; NF, Neurotrophic factors; NGCs, Nerve guidance channels; NGF, Nerve growth factor; NPCs, Neural progenitor cells; NSCs, Neural stem cells; NSPCs, Neural stem progenitor cells; P(AAm), Poly(Acrylamide); PAN-MA, Poly(Acrylonitrile-co-Methylacrylate); PAs, Peptide amphiphiles; PCL, Poly(ε-Caprolactone); PDMS, Poly(Dimethylsiloxane); PEG, Poly(Ethyleneglycol); PEI, Poly(Ethyleneimine); PL, Poly(Lysine); PLGA, Poly(L-lactide-co-glycolide); PLLA, Poly(L-lactic acid); pNE, Poly(Norepinephrine); PNS, Peripheral nervous system; PO, Poly(Ornithine); PPI, Poly(Propyleneimine); PVC, Poly(Vinyl chloride); RFG, Random fibrin hydrogel; SAPs, Self-assembling peptides; SF, Silk fibroin; SGN, Spiral ganglion neurons; TCP, Tissue culture plate.
References
Abbasi, N., Soudi, S., Hayati-Roodbari, N., Dodel, M., and Soleimani, M. (2014). The effects of plasma treated electrospun nanofibrous poly (ε-caprolactone) scaffolds with different orientations on mouse embryonic stem cell proliferation. Cell J. 16, 245–254. Available online at: http://celljournal.org/journal/article/abstract/275
Akizawa, Y., Morita, Y., Hsu, Y., Yamaoka, T., and Nakamachi, E. (2016). “Development of IKVAV Modified PLLA Guide Tube Having Unidirectional Fibers on Inner Surface to Enhance Axonal Extension,”in: ASME 2016 International Mechanical Engineering Congress and Exposition (American Society of Mechanical Engineers), V003T004A073-V003T004A073.
Ali, S., Wall, I. B., Mason, C., Pelling, A. E., and Veraitch, F. S. (2015). The effect of Young's modulus on the neuronal differentiation of mouse embryonic stem cells. Acta Biomater. 25, 253–267. doi: 10.1016/j.actbio.2015.07.008
An, B., Tang-Schomer, M. D., Huang, W., He, J., Jones, J. A., Lewis, R. V., et al. (2015). Physical and biological regulation of neuron regenerative growth and network formation on recombinant dragline silks. Biomaterials 48, 137–146. doi: 10.1016/j.biomaterials.2015.01.044
Ananthanarayanan, B., Little, L., Schaffer, D. V., Healy, K. E., and Tirrell, M. (2010). Neural stem cell adhesion and proliferation on phospholipid bilayers functionalized with RGD peptides. Biomaterials 31, 8706–8715. doi: 10.1016/j.biomaterials.2010.07.104
Béduer, A., Vieu, C., Arnauduc, F., Sol, J.-C., Loubinoux, I., and Vaysse, L. (2012). Engineering of adult human neural stem cells differentiation through surface micropatterning. Biomaterials 33, 504–514. doi: 10.1016/j.biomaterials.2011.09.073
Belkin, A. M., and Stepp, M. A. (2000). Integrins as receptors for laminins. Micro. Res. Tech. 51, 280–301. doi: 10.1002/1097-0029(20001101)51:3<280::AID-JEMT7>3.0.CO;2-O
Berns, E. J., Sur, S., Pan, L., Goldberger, J. E., Suresh, S., Zhang, S., et al. (2014). Aligned neurite outgrowth and directed cell migration in self-assembled monodomain gels. Biomaterials 35, 185–195. doi: 10.1016/j.biomaterials.2013.09.077
Bhang, S. H., Lee, T. J., Lim, J. M., Lim, J. S., Han, A. M., Choi, C. Y., et al. (2009). The effect of the controlled release of nerve growth factor from collagen gel on the efficiency of neural cell culture. Biomaterials 30, 126–132. doi: 10.1016/j.biomaterials.2008.09.021
Bhutto, M. A., Wu, T., Sun, B., Hany, E. H., Al-Deyab, S. S., and Mo, X. (2016). Fabrication and characterization of vitamin B5 loaded poly (l-lactide-co-caprolactone)/silk fiber aligned electrospun nanofibers for schwann cell proliferation. Coll. Surf. B Biointerf. 144, 108–117. doi: 10.1016/j.colsurfb.2016.04.013
Borkenhagen, M., Clémence, J. F., Sigrist, H., and Aebischer, P. (1998). Three-dimensional extracellular matrix engineering in the nervous system. J. Biomed. Mater. Res. A 40, 392–400.
Bozza, A., Coates, E. E., Incitti, T., Ferlin, K. M., Messina, A., Menna, E., et al. (2014). Neural differentiation of pluripotent cells in 3D alginate-based cultures. Biomaterials 35, 4636–4645. doi: 10.1016/j.biomaterials.2014.02.039
Caliari, S. R., and Burdick, J. A. (2016). A practical guide to hydrogels for cell culture. Nat. Methods 13, 405–414. doi: 10.1038/nmeth.3839
Callahan, L. A. S., Xie, S., Barker, I. A., Zheng, J., Reneker, D. H., Dove, A. P., et al. (2013). Directed differentiation and neurite extension of mouse embryonic stem cell on aligned poly (lactide) nanofibers functionalized with YIGSR peptide. Biomaterials 34, 9089–9095. doi: 10.1016/j.biomaterials.2013.08.028
Calof, A. L., and Lander, A. D. (1991). Relationship between neuronal migration and cell-substratum adhesion: laminin and merosin promote olfactory neuronal migration but are anti-adhesive. J. Cell Biol. 115, 779–794. doi: 10.1083/jcb.115.3.779
Carlson, A. L., Bennett, N. K., Francis, N. L., Halikere, A., Clarke, S., Moore, J. C., et al. (2016). Generation and transplantation of reprogrammed human neurons in the brain using 3D microtopographic scaffolds. Nat. Commun. 7:10862. doi: 10.1038/ncomms10862
Casarosa, S., Bozzi, Y., and Conti, L. (2014). Neural stem cells: ready for therapeutic applications? Mol. Cell. Ther. 2:31. doi: 10.1186/2052-8426-2-31
Ceballos, D., Navarro, X., Dubey, N., Wendelschafer-Crabb, G., Kennedy, W. R., and Tranquillo, R. T. (1999). Magnetically aligned collagen gel filling a collagen nerve guide improves peripheral nerve regeneration. Exp. Neurol. 158, 290–300. doi: 10.1006/exnr.1999.7111
Chen, C.-S., Soni, S., Le, C., Biasca, M., Farr, E., Chen, E. Y., et al. (2012). Human stem cell neuronal differentiation on silk-carbon nanotube composite. Nanoscale Res. Lett. 7:126. doi: 10.1186/1556-276X-7-126
Cheng, T. Y., Chen, M. H., Chang, W. H., Huang, M. Y., and Wang, T. W. (2013). Neural stem cells encapsulated in a functionalized self-assembling peptide hydrogel for brain tissue engineering. Biomaterials 34, 2005–2016. doi: 10.1016/j.biomaterials.2012.11.043
Chiono, V., and Tonda-Turo, C. (2015). Trends in the design of nerve guidance channels in peripheral nerve tissue engineering. Prog. Neurobiol. 131, 87–104. doi: 10.1016/j.pneurobio.2015.06.001
Chiono, V., Tonda-Turo, C., and Ciardelli, G. (2009). “Chapter 9: Artificial scaffolds for peripheral nerve reconstruction,” in International Review of Neurobiology, eds S. Geuna, P. Tos, and B. Battiston (Academic Press), 173–198.
Christopherson, G. T., Song, H., and Mao, H.-Q. (2009). The influence of fiber diameter of electrospun substrates on neural stem cell differentiation and proliferation. Biomaterials 30, 556–564. doi: 10.1016/j.biomaterials.2008.10.004
Chua, J. S., Chng, C.-P., Moe, A. A. K., Tann, J. Y., Goh, E. L., Chiam, K.-H., et al. (2014). Extending neurites sense the depth of the underlying topography during neuronal differentiation and contact guidance. Biomaterials 35, 7750–7761. doi: 10.1016/j.biomaterials.2014.06.008
Clements, I. P., Kim, Y.-,t., English, A. W., Lu, X., Chung, A., and Bellamkonda, R. V. (2009). Thin-film enhanced nerve guidance channels for peripheral nerve repair. Biomaterials 30, 3834–3846. doi: 10.1016/j.biomaterials.2009.04.022
Cooke, M., Vulic, K., and Shoichet, M. (2010). Design of biomaterials to enhance stem cell survival when transplanted into the damaged central nervous system. Soft Matter 6, 4988–4998. doi: 10.1039/c0sm00448k
Corey, J. M., Lin, D. Y., Mycek, K. B., Chen, Q., Samuel, S., Feldman, E. L., et al. (2007). Aligned electrospun nanofibers specify the direction of dorsal root ganglia neurite growth. J. Biomed. Mater. Res. A 83, 636–645. doi: 10.1002/jbm.a.31285
Cui, H., Webber, M. J., and Stupp, S. I. (2010). Self-assembly of peptide amphiphiles: from molecules to nanostructures to biomaterials. Peptide Sci. 94, 1–18. doi: 10.1002/bip.21328
Cunha, C., Panseri, S., Villa, O., Silva, D., and Gelain, F. (2011). 3D culture of adult mouse neural stem cells within functionalized self-assembling peptide scaffolds. Int. J. Nanomed. 6:943. doi: 10.2147/IJN.S17292
Daly, W., Yao, L., Zeugolis, D., Windebank, A., and Pandit, A. (2012). A biomaterials approach to peripheral nerve regeneration: bridging the peripheral nerve gap and enhancing functional recovery. J. R. Soc. Interf. 9, 202–221. doi: 10.1098/rsif.2011.0438
de Ruiter, G. C., Spinner, R. J., Malessy, M. J. A., Moore, M. J., Sorenson, E. J., Currier, B. L., et al. (2008). Accuracy of motor axon regeneration across autograft, single-lumen, and multichannel poly(lactic-co-glycolic acid) nerve tubes. Neurosurgery 63, 144–153. doi: 10.1227/01.NEU.0000335081.47352.78
Dodla, M. C., and Bellamkonda, R. V. (2008). Differences between the effect of anisotropic and isotropic laminin and nerve growth factor presenting scaffolds on nerve regeneration across long peripheral nerve gaps. Biomaterials 29, 33–46. doi: 10.1016/j.biomaterials.2007.08.045
Dubey, N., Letourneau, P., and Tranquillo, R. (1999). Guided neurite elongation and Schwann cell invasion into magnetically aligned collagen in simulated peripheral nerve regeneration. Exp. Neurol. 158, 338–350. doi: 10.1006/exnr.1999.7095
East, E., de Oliveira, D. B., Golding, J. P., and Phillips, J. B. (2010). Alignment of astrocytes increases neuronal growth in three-dimensional collagen gels and is maintained following plastic compression to form a spinal cord repair conduit. Tissue Eng. A 16, 3173–3184. doi: 10.1089/ten.tea.2010.0017
Engler, A. J., Sen, S., Sweeney, H. L., and Discher, D. E. (2006). Matrix elasticity directs stem cell lineage specification. Cell 126, 677–689. doi: 10.1016/j.cell.2006.06.044
Farrukh, A., Ortega, F., Fan, W., Marichal, N., Paez, J. I., Berninger, B., et al. (2017a). Bifunctional hydrogels containing the laminin motif IKVAV promote neurogenesis. Stem Cell Rep. 9, 1432–1440 doi: 10.1016/j.stemcr.2017.09.002
Farrukh, A., Paez, J. I., Salierno, M., Fan, W., Berninger, B., and del Campo, A. (2017b). Bifunctional poly (acrylamide) hydrogels through orthogonal coupling chemistries. Biomacromolecules 18, 906–913. doi: 10.1021/acs.biomac.6b01784
Fawcett, J. (2002). Repair of spinal cord injuries: where are we, where are we going? Spinal Cord 40:615. doi: 10.1038/sj.sc.3101328
Fawcett, J. W. (1998). Spinal cord repair: from experimental models to human application. Spinal Cord 36, 811–817. doi: 10.1038/sj.sc.3100769
Franze, K. (2011). Atomic force microscopy and its contribution to understanding the development of the nervous system. Curr. Opin. Genet. Dev. 21, 530–537. doi: 10.1016/j.gde.2011.07.001
Franze, K. (2013). The mechanical control of nervous system development. Development 140, 3069–3077. doi: 10.1242/dev.079145
Freitas, V. M., Vilas-Boas, V. F., Pimenta, D. C., Loureiro, V., Juliano, M. A., Carvalho, M. R., et al. (2007). SIKVAV, a laminin α1-derived peptide, interacts with integrins and increases protease activity of a human salivary gland adenoid cystic carcinoma cell line through the ERK 1/2 signaling pathway. Am. J. Pathol. 171, 124–138. doi: 10.2353/ajpath.2007.051264
Frick, C., Müller, M., Wank, U., Tropitzsch, A., Kramer, B., Senn, P., et al. (2017). Biofunctionalized peptide-based hydrogels provide permissive scaffolds to attract neurite outgrowth from spiral ganglion neurons. Coll. Surf. B Biointerf. 149, 105–114. doi: 10.1016/j.colsurfb.2016.10.003
Frith, J. E., Mills, R. J., Hudson, J. E., and Cooper-White, J. J. (2012). Tailored integrin–extracellular matrix interactions to direct human mesenchymal stem cell differentiation. Stem Cells Dev. 21, 2442–2456. doi: 10.1089/scd.2011.0615
Fujioka, T., Kaneko, N., Ajioka, I., Nakaguchi, K., Omata, T., Ohba, H., et al. (2017). β1 integrin signaling promotes neuronal migration along vascular scaffolds in the post-stroke brain. EBioMed. 16(Suppl. C), 195–203. doi: 10.1016/j.ebiom.2017.01.005
Gage, F. H., and Temple, S. (2013). Neural stem cells: generating and regenerating the brain. Neuron 80, 588–601. doi: 10.1016/j.neuron.2013.10.037
Gelain, F., Bottai, D., Vescovi, A., and Zhang, S. (2006). Designer self-assembling peptide nanofiber scaffolds for adult mouse neural stem cell 3-dimensional cultures. PLoS ONE 1:e119. doi: 10.1371/journal.pone.0000119
Ghasemi-Mobarakeh, L., Prabhakaran, M. P., Morshed, M., Nasr-Esfahani, M. H., and Ramakrishna, S. (2010). Bio-functionalized PCL nanofibrous scaffolds for nerve tissue engineering. Mater. Sci. Eng. C 30, 1129–1136. doi: 10.1016/j.msec.2010.06.004
Gnavi, S., Fornasari, B. E., Tonda-Turo, C., Laurano, R., Zanetti, M., Ciardelli, G., et al. (2015). The effect of electrospun gelatin fibers alignment on Schwann cell and axon behavior and organization in the perspective of artificial nerve design. Int. J. Mol. Sci. 16, 12925–12942. doi: 10.3390/ijms160612925
Gomez, N., Chen, S., and Schmidt, C. E. (2007). Polarization of hippocampal neurons with competitive surface stimuli: contact guidance cues are preferred over chemical ligands. J. R. Soc. Interf. 4, 223–233. doi: 10.1098/rsif.2006.0171
Göpferich, A. (1996). Mechanisms of polymer degradation and erosion. Biomaterials 17, 103–114. doi: 10.1016/0142-9612(96)85755-3
Gu, X. S., Ding, F., Yang, Y. M., and Liu, J. (2011). Construction of tissue engineered nerve grafts and their application in peripheral nerve regeneration. Prog. Neurobiol. 93, 204–230. doi: 10.1016/j.pneurobio.2010.11.002
Guarnieri, D., Battista, S., Borzacchiello, A., Mayol, L., De Rosa, E., Keene, D., et al. (2007). Effects of fibronectin and laminin on structural, mechanical and transport properties of 3D collageneous network. J. Mater. Sci. Mater. Med. 18, 245–253. doi: 10.1007/s10856-006-0686-5
Gumera, C., Rauck, B., and Wang, Y. (2011). Materials for central nervous system regeneration: bioactive cues. J. Mater. Chem. 21, 7033–7051. doi: 10.1039/c0jm04335d
Günther, M. I., Weidner, N., Müller, R., and Blesch, A. (2015). Cell-seeded alginate hydrogel scaffolds promote directed linear axonal regeneration in the injured rat spinal cord. Acta Biomater. 27, 140–150. doi: 10.1016/j.actbio.2015.09.001
Hamsici, S., Cinar, G., Celebioglu, A., Uyar, T., Tekinay, A. B., and Guler, M. O. (2017). Bioactive peptide functionalized aligned cyclodextrin nanofibers for neurite outgrowth. J. Mater. Chem. B 5, 517–524. doi: 10.1039/C6TB02441F
Hanein, Y., Tadmor, O., Anava, S., and Ayali, A. (2011). Neuronal soma migration is determined by neurite tension. Neuroscience 172, 572–579. doi: 10.1016/j.neuroscience.2010.10.022
He, L., Zhang, Y., Zeng, C., Ngiam, M., Liao, S., Quan, D., et al. (2009). Manufacture of PLGA multiple-channel conduits with precise hierarchical pore architectures and in vitro/vivo evaluation for spinal cord injury. Tissue Eng. C Methods 15, 243–255. doi: 10.1089/ten.tec.2008.0255
Hiraoka, M., Kato, K., Nakaji-Hirabayashi, T., and Iwata, H. (2009). Enhanced survival of neural cells embedded in hydrogels composed of collagen and laminin-derived cell adhesive peptide. Bioconjug. Chem. 20, 976–983. doi: 10.1021/bc9000068
Ho, M., Yu, D., Davidsion, M. C., and Silva, G. A. (2006). Comparison of standard surface chemistries for culturing mesenchymal stem cells prior to neural differentiation. Biomaterials 27, 4333–4339. doi: 10.1016/j.biomaterials.2006.03.037
Hoffman-Kim, D., Mitchel, J. A., and Bellamkonda, R. V. (2010). Topography, cell response, and nerve regeneration. Annu. Rev. Biomed. Eng. 12, 203–231. doi: 10.1146/annurev-bioeng-070909-105351
Hosseinkhani, H., Hiraoka, Y., Li, C.-H., Chen, Y.-R., Yu, D.-S., Hong, P.-D., et al. (2013). Engineering three-dimensional collagen-IKVAV matrix to mimic neural microenvironment. ACS Chem. Neurosci. 4, 1229–1235. doi: 10.1021/cn400075h
Hsieh, F.-Y., Lin, H.-H., and Hsu, S.-,h. (2015). 3D bioprinting of neural stem cell-laden thermoresponsive biodegradable polyurethane hydrogel and potential in central nervous system repair. Biomaterials 71, 48–57. doi: 10.1016/j.biomaterials.2015.08.028
Ilkhanizadeh, S., Teixeira, A. I., and Hermanson, O. (2007). Inkjet printing of macromolecules on hydrogels to steer neural stem cell differentiation. Biomaterials 28, 3936–3943. doi: 10.1016/j.biomaterials.2007.05.018
Iwata, A., Browne, K. D., Pfister, B. J., Gruner, J. A., and Smith, D. H. (2006). Long-term survival and outgrowth of mechanically engineered nervous tissue constructs implanted into spinal cord lesions. Tissue Eng. 12, 101–110. doi: 10.1089/ten.2006.12.101
Johnson, B. N., Lancaster, K. Z., Zhen, G., He, J., Gupta, M. K., Kong, Y. L., et al. (2015). 3D printed anatomical nerve regeneration pathways. Adv. Funct. Mater. 25, 6205–6217. doi: 10.1002/adfm.201501760
Joo, S., Kang, K., and Nam, Y. (2015a). In vitro neurite guidance effects induced by polylysine pinstripe micropatterns with polylysine background. J. Biomed. Mater. Res. A 103, 2731–2739. doi: 10.1002/jbm.a.35405
Joo, S., Kim, J. Y., Lee, E., Hong, N., Sun, W., and Nam, Y. (2015b). Effects of ECM protein micropatterns on the migration and differentiation of adult neural stem cells. Sci. Rep. 5:13043. doi: 10.1038/srep13043
Kabu, S., Gao, Y., Kwon, B. K., and Labhasetwar, V. (2015). Drug delivery, cell-based therapies, and tissue engineering approaches for spinal cord injury. J. Control. Release 219, 141–154. doi: 10.1016/j.jconrel.2015.08.060
Kang, K., Choi, I. S., and Nam, Y. (2011). A biofunctionalization scheme for neural interfaces using polydopamine polymer. Biomaterials 32, 6374–6380. doi: 10.1016/j.biomaterials.2011.05.028
Karow, M., Sánchez, R., Schichor, C., Masserdotti, G., Ortega, F., Heinrich, C., et al. (2012). Reprogramming of pericyte-derived cells of the adult human brain into induced neuronal cells. Cell Stem Cell 11, 471–476. doi: 10.1016/j.stem.2012.07.007
Kharkar, P. M., Kiick, K. L., and Kloxin, A. M. (2013). Designing degradable hydrogels for orthogonal control of cell microenvironments. Chem. Soc. Rev. 42, 7335–7372. doi: 10.1039/C3CS60040H
Kijenska, E., Prabhakaran, M. P., Swieszkowski, W., Kurzydlowski, K. J., and Ramakrishna, S. (2014). Interaction of Schwann cells with laminin encapsulated PLCL core–shell nanofibers for nerve tissue engineering. Eur. Polym. J. 50, 30–38. doi: 10.1016/j.eurpolymj.2013.10.021
Kilian, K. A., and Mrksich, M. (2012). Directing stem cell fate by controlling the affinity and density of ligand–receptor interactions at the biomaterials interface. Angew. Chem. Int. Ed. 51, 4891–4895. doi: 10.1002/anie.201108746
Kim, H., Zahir, T., Tator, C. H., and Shoichet, M. S. (2011). Effects of dibutyryl cyclic-AMP on survival and neuronal differentiation of neural stem/progenitor cells transplanted into spinal cord injured rats. PLoS ONE 6:e21744. doi: 10.1371/journal.pone.0021744
Kim, S.-E., Harker, E. C., De Leon, A. C., Advincula, R. C., and Pokorski, J. K. (2015). Coextruded, aligned, and gradient-modified poly (ε-caprolactone) fibers as platforms for neural growth. Biomacromolecules 16, 860–867. doi: 10.1021/bm501767x
Kim, Y.-,t., Haftel, V. K., Kumar, S., and Bellamkonda, R. V. (2008). The role of aligned polymer fiber-based constructs in the bridging of long peripheral nerve gaps. Biomaterials 29, 3117–3127. doi: 10.1016/j.biomaterials.2008.03.042
Koh, H., Yong, T., Chan, C., and Ramakrishna, S. (2008). Enhancement of neurite outgrowth using nano-structured scaffolds coupled with laminin. Biomaterials 29, 3574–3582. doi: 10.1016/j.biomaterials.2008.05.014
Kolb, B., Morshead, C., Gonzalez, C., Kim, M., Gregg, C., Shingo, T., et al. (2007). Growth factor-stimulated generation of new cortical tissue and functional recovery after stroke damage to the motor cortex of rats. J. Cereb. Blood Flow Metabol. 27, 983–997. doi: 10.1038/sj.jcbfm.9600402
Koutsopoulos, S., and Zhang, S. (2013). Long-term three-dimensional neural tissue cultures in functionalized self-assembling peptide hydrogels, matrigel and collagen I. Acta Biomater. 9, 5162–5169. doi: 10.1016/j.actbio.2012.09.010
Kubinová, Š., Horák, D., Kozubenko, N., Vaněček, V., Proks, V., Price, J., et al. (2010). The use of superporous Ac-CGGASIKVAVS-OH-modified PHEMA scaffolds to promote cell adhesion and the differentiation of human fetal neural precursors. Biomaterials 31, 5966–5975. doi: 10.1016/j.biomaterials.2010.04.040
Kulangara, K., Adler, A. F., Wang, H., Chellappan, M., Hammett, E., Yasuda, R., et al. (2014). The effect of substrate topography on direct reprogramming of fibroblasts to induced neurons. Biomaterials 35, 5327–5336. doi: 10.1016/j.biomaterials.2014.03.034
Lampe, K. J., Antaris, A. L., and Heilshorn, S. C. (2013). Design of three-dimensional engineered protein hydrogels for tailored control of neurite growth. Acta Biomater. 9, 5590–5599. doi: 10.1016/j.actbio.2012.10.033
Laura, M., Leipzig, N. D., and Shoichet, M. S. (2008). Promoting neuron adhesion and growth. Mater. Today 11, 36–43. doi: 10.1016/S1369-7021(08)70088-9
Lee, J., Abdeen, A. A., Zhang, D., and Kilian, K. A. (2013). Directing stem cell fate on hydrogel substrates by controlling cell geometry, matrix mechanics and adhesion ligand composition. Biomaterials 34, 8140–8148. doi: 10.1016/j.biomaterials.2013.07.074
Lee, M. K., Qin, E. C., Rich, M., Lee, K. Y., Kim, D. H., Chung, H. J., et al. (2016). Three dimensional conjugation of recombinant N-cadherin to a hydrogel for in vitro anisotropic neural growth. J. Mater. Chem. B 4, 6803–6811. doi: 10.1039/C6TB01814A
Lee, M. K., Rich, M. H., Lee, J., and Kong, H. (2015). A bio-inspired, microchanneled hydrogel with controlled spacing of cell adhesion ligands regulates 3D spatial organization of cells and tissue. Biomaterials 58, 26–34. doi: 10.1016/j.biomaterials.2015.04.014
Lee, W., Frank, C. W., and Park, J. (2014). Directed axonal outgrowth using a propagating gradient of IGF-1. Adv. Mater. 26, 4936–4940. doi: 10.1002/adma.201305995
Lei, W. L., Xing, S. G., Deng, C. Y., Ju, X. C., Jiang, X. Y., and Luo, Z. G. (2012). Laminin/β1 integrin signal triggers axon formation by promoting microtubule assembly and stabilization. Cell Res. 22, 954–972. doi: 10.1038/cr.2012.40
Leipzig, N. D., and Shoichet, M. S. (2009). The effect of substrate stiffness on adult neural stem cell behavior. Biomaterials 30, 6867–6878. doi: 10.1016/j.biomaterials.2009.09.002
Lewicka, M., Hermanson, O., and Rising, A. U. (2012). Recombinant spider silk matrices for neural stem cell cultures. Biomaterials 33, 7712–7717. doi: 10.1016/j.biomaterials.2012.07.021
Li Jeon, L, Baskaran, H., Dertinger, S. K., Whitesides, G. M., Van De Water, L., and Toner, M. (2002). Neutrophil chemotaxis in linear and complex gradients of interleukin-8 formed in a microfabricated device. Nat. Biotechnol. 20, 826–830. doi: 10.1038/nbt712
Li, A., Hokugo, A., Yalom, A., Berns, E. J., Stephanopoulos, N., McClendon, M. T., et al. (2014). A bioengineered peripheral nerve construct using aligned peptide amphiphile nanofibers. Biomaterials 35, 8780–8790. doi: 10.1016/j.biomaterials.2014.06.049
Li, H., Koenig, A. M., Sloan, P., and Leipzig, N. D. (2014). In vivo assessment of guided neural stem cell differentiation in growth factor immobilized chitosan-based hydrogel scaffolds. Biomaterials 35, 9049–9057. doi: 10.1016/j.biomaterials.2014.07.038
Li, W., Tang, Q. Y., Jadhav, A. D., Narang, A., Qian, W. X., Shi, P., et al. (2015). Large-scale topographical screen for investigation of physical neural-guidance cues. Sci. Rep. 5:8664. doi: 10.1038/srep08644
Li, X., Liu, X., Josey, B., Chou, C. J., Tan, Y., Zhang, N., et al. (2014). Short laminin peptide for improved neural stem cell growth. Stem Cells Transl. Med. 3, 662–670. doi: 10.5966/sctm.2013-0015
Lietz, M., Dreesmann, L., Hoss, M., Oberhoffner, S., and Schlosshauer, B. (2006). Neuro tissue engineering of glial nerve guides and the impact of different cell types. Biomaterials 27, 1425–1436. doi: 10.1016/j.biomaterials.2005.08.007
Liu, B., Ma, J., Xu, Q., and Cui, F. (2006). Regulation of charged groups and laminin patterns for selective neuronal adhesion. Coll. Surf. B Biointerf. 53, 175–178. doi: 10.1016/j.colsurfb.2006.08.018
Liu, K., Tedeschi, A., Park, K. K., and He, Z. (2011). Neuronal intrinsic mechanisms of axon regeneration. Annu. Rev. Neurosci. 34, 131–152. doi: 10.1146/annurev-neuro-061010-113723
Lu, Y. P., Yang, C. H., Yeh, J. A., Ho, F. H., Ou, Y. C., Chen, C. H., et al. (2014). Guidance of neural regeneration on the biomimetic nanostructured matrix. Int. J. Pharm. 463, 177–183. doi: 10.1016/j.ijpharm.2013.08.006
Luca, A. C., Terenghi, G., and Downes, S. (2014). Chemical surface modification of poly-ε-caprolactone improves Schwann cell proliferation for peripheral nerve repair. J. Tissue Eng. Regen. Med. 8, 153–163. doi: 10.1002/term.1509
Lundborg, G., Dahlin, L., Dohi, D., Kanje, M., and Terada, N. (1997). A new type of “bioartificial” nerve graft for bridging extended defects in nerves. J. Hand Surg. Br Eur. Vol. 22, 299–303.
Lunn, J. S., Sakowski, S. A., Hur, J., and Feldman, E. L. (2011). Stem cell technology for neurodegenerative diseases. Ann. Neurol. 70, 353–361. doi: 10.1002/ana.22487
Ma, F., Xiao, Z., Chen, B., Hou, X., Han, J., Zhao, Y., et al. (2014). Accelerating proliferation of neural stem/progenitor cells in collagen sponges immobilized with engineered basic fibroblast growth factor for nervous system tissue engineering. Biomacromolecules 15, 1062–1068. doi: 10.1021/bm500062n
Madl, C. M., Katz, L. M., and Heilshorn, S. C. (2016). Bio-orthogonally crosslinked, engineered protein hydrogels with tunable mechanics and biochemistry for cell encapsulation. Adv. Funct. Mater. 26, 3612–3620. doi: 10.1002/adfm.201505329
Malatesta, P., Appolloni, I., and Calzolari, F. (2008). Radial glia and neural stem cells. Cell Tissue Res. 331, 165–178. doi: 10.1007/s00441-007-0481-8
Mammadov, B., Sever, M., Guler, M. O., and Tekinay, A. B. (2013). Neural differentiation on synthetic scaffold materials. Biomater. Sci. 1, 1119–1137. doi: 10.1039/c3bm60150a
Manchineella, S., Thrivikraman, G., Basu, B., and Govindaraju, T. (2016). Surface-functionalized silk fibroin films as a platform to guide neuron-like differentiation of human mesenchymal stem cells. ACS Appl. Mater. Interf. 8, 22849–22859. doi: 10.1021/acsami.6b06403
Mason, H. A., Ito, S., and Corfas, G. (2001). Extracellular signals that regulate the tangential migration of olfactory bulb neuronal precursors: inducers, inhibitors, and repellents. J. Neurosci. 21, 7654–7663. doi: 10.1523/JNEUROSCI.21-19-07654.2001
Matsumoto, K., Ohnishi, K., Kiyotani, T., Sekine, T., Ueda, H., Nakamura, T., et al. (2000). Peripheral nerve regeneration across an 80-mm gap bridged by a polyglycolic acid (PGA)-collagen tube filled with laminin-coated collagen fibers: a histological and electrophysiological evaluation of regenerated nerves. Brain Res. 868, 315–328. doi: 10.1016/S0006-8993(00)02207-1
McKinnon, D., Domaille, D., Brown, T., Kyburz, K., Kiyotake, E., Cha, J., et al. (2014). Measuring cellular forces using bis-aliphatic hydrazone crosslinked stress-relaxing hydrogels. Soft Matter 10, 9230–9236. doi: 10.1039/C4SM01365D
Meyer, C., Stenberg, L., Gonzalez-Perez, F., Wrobel, S., Ronchi, G., Udina, E., et al. (2016). Chitosan-film enhanced chitosan nerve guides for long-distance regeneration of peripheral nerves. Biomaterials 76, 33–51. doi: 10.1016/j.biomaterials.2015.10.040
Micholt, L., Gärtner, A., Prodanov, D., Braeken, D., Dotti, C. G., and Bartic, C. (2013). Substrate topography determines neuronal polarization and growth in vitro. PLoS ONE 8:e66170. doi: 10.1371/journal.pone.0066170
Miller, C., Jeftinija, S., and Mallapragada, S. (2001). Micropatterned Schwann cell–seeded biodegradable polymer substrates significantly enhance neurite alignment and outgrowth. Tissue Eng. 7, 705–715. doi: 10.1089/107632701753337663
Mizuno, A., Matsuda, K., and Shuto, S. (2017). Peptides to peptidomimetics: a strategy based on the structural features of cyclopropane. Chemistry 23, 14394–14409. doi: 10.1002/chem.201702119
Mochizuki, M., Philp, D., Hozumi, K., Suzuki, N., Yamada, Y., Kleinman, H. K., et al. (2007). Angiogenic activitiy of syndecan-binding laminin peptide AG73 (RKRLQVQLSIRT). Arch. Biochem. Biophys. 459, 249–255. doi: 10.1016/j.abb.2006.12.026
Mohtaram, N. K., Ko, J., King, C., Sun, L., Muller, N., Jun, M. B. G., et al. (2015). Electrospun biomaterial scaffolds with varied topographies for neuronal differentiation of human-induced pluripotent stem cells. J. Biomed. Mater. Res. A 103, 2591–2601. doi: 10.1002/jbm.a.35392
Moore, K., Macsween, M., and Shoichet, M. (2006). Immobilized concentration gradients of neurotrophic factors guide neurite outgrowth of primary neurons in macroporous scaffolds. Tissue Eng. 12, 267–278. doi: 10.1089/ten.2006.12.267
Mothe, A. J., Tam, R. Y., Zahir, T., Tator, C. H., and Shoichet, M. S. (2013). Repair of the injured spinal cord by transplantation of neural stem cells in a hyaluronan-based hydrogel. Biomaterials 34, 3775–3783. doi: 10.1016/j.biomaterials.2013.02.002
Nagamine, K., Hirata, T., Okamoto, K., Abe, Y., Kaji, H., and Nishizawa, M. (2015). Portable micropatterns of neuronal cells supported by thin hydrogel films. ACS Biomater. Sci. Eng. 1, 329–334. doi: 10.1021/acsbiomaterials.5b00020
Nakaji-Hirabayashi, T., Kato, K., and Iwata, H. (2013). In vivo study on the survival of neural stem cells transplanted into the rat brain with a collagen hydrogel that incorporates laminin-derived polypeptides. Bioconjug. Chem. 24, 1798–1804. doi: 10.1021/bc400005m
Nakayama, K., Takakuda, K., Koyama, Y., Itoh, S., Wang, W., Mukai, T., et al. (2007). Enhancement of peripheral nerve regeneration using bioabsorbable polymer tubes packed with fibrin gel. Artificial Organs 31, 500–508. doi: 10.1111/j.1525-1594.2007.00418.x
Nasir, S., Ramirez, P., Ali, M., Ahmed, I., Fruk, L., Mafe, S., et al. (2013). Nernst-Planck model of photo-triggered, p H–tunable ionic transport through nanopores functionalized with “caged” lysine chains. J. Chem. Phys. 138:034709. doi: 10.1063/1.4775811
Newman, K., McLaughlin, C., Carlsson, D., Li, F., Liu, Y., and Griffith, M. (2006). Bioactive hydrogel-filament scaffolds for nerve repair and regeneration. Int. J. Artif. Organs 29, 1082–1091. doi: 10.1177/039139880602901109
Nikkhah, M., Edalat, F., Manoucheri, S., and Khademhosseini, A. (2012). Engineering microscale topographies to control the cell-substrate interface. Biomaterials 33, 5230–5246. doi: 10.1016/j.biomaterials.2012.03.079
Norman, L. L., and Aranda-Espinoza, H. (2010). Cortical neuron outgrowth is insensitive to substrate stiffness. Cell. Mol. Bioeng. 3, 398–414. doi: 10.1007/s12195-010-0137-8
Novikova, L. N., Pettersson, J., Brohlin, M., Wiberg, M., and Novikov, L. N. (2008). Biodegradable poly-β-hydroxybutyrate scaffold seeded with Schwann cells to promote spinal cord repair. Biomaterials 29, 1198–1206. doi: 10.1016/j.biomaterials.2007.11.033
Nune, M., Krishnan, U. M., and Sethuraman, S. (2016). PLGA nanofibers blended with designer self-assembling peptides for peripheral neural regeneration. Mater. Sci. Eng. C 62, 329–337. doi: 10.1016/j.msec.2016.01.057
Ortega, F., Gascon, S., Masserdotti, G., Deshpande, A., Simon, C., Fischer, J., et al. (2013). Oligodendrogliogenic and neurogenic adult subependymal zone neural stem cells constitute distinct lineages and exhibit differential responsiveness to Wnt signalling. Nat. Cell Biol. 15:602. doi: 10.1038/ncb2736
Pakulska, M. M., Ballios, B. G., and Shoichet, M. S. (2012). Injectable hydrogels for central nervous system therapy. Biomed. Mater. 7:024101. doi: 10.1088/1748-6041/7/2/024101
Park, M., Shin, M., Kim, E., Lee, S., Park, K. I., Lee, H., et al. (2014). The promotion of human neural stem cells adhesion using bioinspired poly (norepinephrine) nanoscale coating. J. Nanomater. 2014:2. doi: 10.1155/2014/793052
Park, S. Y., Park, J., Sim, S. H., Sung, M. G., Kim, K. S., Hong, B. H., et al. (2011). Enhanced differentiation of human neural stem cells into neurons on graphene. Adv. Mater. 23:H263–7. doi: 10.1002/adma.201101503
Pek, Y. S., Wan, A. C., and Ying, J. Y. (2010). The effect of matrix stiffness on mesenchymal stem cell differentiation in a 3D thixotropic gel. Biomaterials 31, 385–391. doi: 10.1016/j.biomaterials.2009.09.057
Pfister, L. A., Papaloïzos, M., Merkle, H. P., and Gander, B. (2007). Nerve conduits and growth factor delivery in peripheral nerve repair. J. Peripher. Nerv. Syst. 12, 65–82. doi: 10.1111/j.1529-8027.2007.00125.x
Roach, P., Parker, T., Gadegaard, N., and Alexander, M. (2010). Surface strategies for control of neuronal cell adhesion: a review. Surf. Sci. Rep. 65, 145–173. doi: 10.1016/j.surfrep.2010.07.001
Rose, J. C., Cámara-Torres, M., Rahimi, K., Köhler, J., Möller, M., and De Laporte, L. (2017). Nerve cells decide to orient inside an injectable hydrogel with minimal structural guidance. Nano Lett. 17, 3782–3791. doi: 10.1021/acs.nanolett.7b01123
Rosso, G., Young, P., and Shahin, V. (2017). Mechanosensitivity of embryonic neurites promotes their directional extension and schwann cells progenitors migration. Cell Physiol. Biochem. 44, 1263–1270. doi: 10.1159/000485485
Sarig-Nadir, O., Livnat, N., Zajdman, R., Shoham, S., and Seliktar, D. (2009). Laser photoablation of guidance microchannels into hydrogels directs cell growth in three dimensions. Biophys. J. 96, 4743–4752. doi: 10.1016/j.bpj.2009.03.019
Schmalenberg, K. E., and Uhrich, K. E. (2005). Micropatterned polymer substrates control alignment of proliferating Schwann cells to direct neuronal regeneration. Biomaterials 26, 1423–1430. doi: 10.1016/j.biomaterials.2004.04.046
Schmid, R. S., and Maness, P. F. (2008). L1 and NCAM adhesion molecules as signaling coreceptors in neuronal migration and process outgrowth. Curr. Opin. Neurobiol. 18, 245–250. doi: 10.1016/j.conb.2008.07.015
Schmidt, C. E., and Leach, J. B. (2003). Neural tissue engineering: Strategies for repair and regeneration. Ann. Rev. Biomed. Eng. 5, 293–347. doi: 10.1146/annurev.bioeng.5.011303.120731
Schnell, E., Klinkhammer, K., Balzer, S., Brook, G., Klee, D., Dalton, P., et al. (2007). Guidance of glial cell migration and axonal growth on electrospun nanofibers of poly-ε-caprolactone and a collagen/poly-ε-caprolactone blend. Biomaterials 28, 3012–3025. doi: 10.1016/j.biomaterials.2007.03.009
Seidlits, S. K., Khaing, Z. Z., Petersen, R. R., Nickels, J. D., Vanscoy, J. E., Shear, J. B., et al. (2010). The effects of hyaluronic acid hydrogels with tunable mechanical properties on neural progenitor cell differentiation. Biomaterials 31, 3930–3940. doi: 10.1016/j.biomaterials.2010.01.125
Sell, S., Barnes, C., Smith, M., McClure, M., Madurantakam, P., Grant, J., et al. (2007). Extracellular matrix regenerated: tissue engineering via electrospun biomimetic nanofibers. Polym. Int. 56, 1349–1360. doi: 10.1002/pi.2344
Serrano, M., Patiño, J., García-Rama, C., Ferrer, M., Fierro, J., Tamayo, A., et al. (2014). 3D free-standing porous scaffolds made of graphene oxide as substrates for neural cell growth. J. Mater. Chem. B 2, 5698–5706. doi: 10.1039/C4TB00652F
Shah, S., Yin, P. T., Uehara, T. M., Chueng, S. T. D., Yang, L., and Lee, K. B. (2014). Guiding stem cell differentiation into oligodendrocytes using graphene-nanofiber hybrid scaffolds. Adv. Mater. 26, 3673–3680. doi: 10.1002/adma.201400523
Shin, H., Jo, S., and Mikos, A. G. (2003). Biomimetic materials for tissue engineering. Biomaterials 24, 4353–4364. doi: 10.1016/S0142-9612(03)00339-9
Silva, G. A., Czeisler, C., Niece, K. L., Beniash, E., Harrington, D. A., Kessler, J. A., et al. (2004). Selective differentiation of neural progenitor cells by high-epitope density nanofibers. Science 303, 1352–1355. doi: 10.1126/science.1093783
Silva, N. A., Cooke, M. J., Tam, R. Y., Sousa, N., Salgado, A. J., Reis, R. L., et al. (2012). The effects of peptide modified gellan gum and olfactory ensheathing glia cells on neural stem/progenitor cell fate. Biomaterials 33, 6345–6354. doi: 10.1016/j.biomaterials.2012.05.050
Smeal, R. M., Rabbitt, R., Biran, R., and Tresco, P. A. (2005). Substrate curvature influences the direction of nerve outgrowth. Ann. Biomed. Eng. 33, 376–382. doi: 10.1007/s10439-005-1740-z
Smeal, R. M., and Tresco, P. A. (2008). The influence of substrate curvature on neurite outgrowth is cell type dependent. Exp. Neurol. 213, 281–292. doi: 10.1016/j.expneurol.2008.05.026
Stephanopoulos, N., Freeman, R., North, H. A., Sur, S., Jeong, S. J., Tantakitti, F., et al. (2014). Bioactive DNA-peptide nanotubes enhance the differentiation of neural stem cells into neurons. Nano Lett. 15, 603–609. doi: 10.1021/nl504079q
Straley, K. S., Foo, C. W., and Heilshorn, S. C. (2010). Biomaterial design strategies for the treatment of spinal cord injuries. J. Neurotrauma 27, 1–19. doi: 10.1089/neu.2009.0948
Struzyna, L. A., Katiyar, K., and Cullen, D. K. (2014). Living scaffolds for neuroregeneration. Curr. Opin. Solid State Mater. Sci. 18, 308–318. doi: 10.1016/j.cossms.2014.07.004
Sun, W., Incitti, T., Migliaresi, C., Quattrone, A., Casarosa, S., and Motta, A. (2017). Viability and neuronal differentiation of neural stem cells encapsulated in silk fibroin hydrogel functionalized with an IKVAV peptide. J. Tissue Eng. Regener. Med. 11, 1532–1541. doi: 10.1002/term.2053
Sun, Y., Li, W., Wu, X., Zhang, N., Zhang, Y., Ouyang, S., et al. (2016). Functional self-assembling peptide nanofiber hydrogels designed for nerve degeneration. ACS Appl. Mater. Interf. 8, 2348–2359. doi: 10.1021/acsami.5b11473
Sur, S., Guler, M. O., Webber, M. J., Pashuck, E. T., Ito, M., Stupp, S. I., et al. (2014). Synergistic regulation of cerebellar Purkinje neuron development by laminin epitopes and collagen on an artificial hybrid matrix construct. Biomater. Sci. 2, 903–914. doi: 10.1039/C3BM60228A
Tarus, D., Hamard, L., Caraguel, F., Wion, D., Szarpak-Jankowska, A., van der Sanden, B., et al. (2016). Design of hyaluronic acid hydrogels to promote neurite outgrowth in three dimensions. ACS Appl. Mater. Interf. 8, 25051–25059. doi: 10.1021/acsami.6b06446
Taylor, A. C., González, C. H., Miller, B. S., Edgington, R. J., Ferretti, P., and Jackman, R. B. (2017). Surface functionalisation of nanodiamonds for human neural stem cell adhesion and proliferation. Sci. Rep. 7:7307. doi: 10.1038/s41598-017-07361-y
Thid, D., Bally, M., Holm, K., Chessari, S., Tosatti, S., Textor, M., et al. (2007). Issues of ligand accessibility and mobility in initial cell attachment. Langmuir 23, 11693–11704. doi: 10.1021/la701159u
Thid, D., Holm, K., Eriksson, P. S., Ekeroth, J., Kasemo, B., and Gold, J. (2008). Supported phospholipid bilayers as a platform for neural progenitor cell culture. J. Biomed. Mater. Res. A 84, 940–953. doi: 10.1002/jbm.a.31358
Thompson, D. M., and Buettner, H. M. (2004). Oriented Schwann cell monolayers for directed neurite outgrowth. Ann. Biomed. Eng. 32, 1121–1131. doi: 10.1114/B:ABME.0000036648.68804.e7
Tian, L., Prabhakaran, M. P., Hu, J., Chen, M., Besenbacher, F., and Ramakrishna, S. (2016). Synergistic effect of topography, surface chemistry and conductivity of the electrospun nanofibrous scaffold on cellular response of PC12 cells. Coll. Surf. B Biointerf. 145, 420–429. doi: 10.1016/j.colsurfb.2016.05.032
Tonda-Turo, C., Audisio, C., Gnavi, S., Chiono, V., Gentile, P., Raimondo, S., et al. (2011). Porous poly (ε-caprolactone) nerve guide filled with porous gelatin matrix for nerve tissue engineering. Adv. Eng. Mater. 13, B151–B164. doi: 10.1002/adem.201080099
Tsai, E. C., Dalton, P. D., Shoichet, M. S., and Tator, C. H. (2004). Synthetic hydrogel guidance channels facilitate regeneration of adult rat brainstem motor axons after complete spinal cord transection. J. Neurotrauma 21, 789–804. doi: 10.1089/0897715041269687
Tuft, B. W., Zhang, L., Xu, L., Hangartner, A., Leigh, B., Hansen, M. R., et al. (2014). Material stiffness effects on neurite alignment to photopolymerized micropatterns. Biomacromolecules 15, 3717–3727. doi: 10.1021/bm501019s
Tysseling-Mattiace, V. M., Sahni, V., Niece, K. L., Birch, D., Czeisler, C., Fehlings, M. G., et al. (2008). Self-assembling nanofibers inhibit glial scar formation and promote axon elongation after spinal cord injury. J. Neurosci. 28, 3814–3823. doi: 10.1523/JNEUROSCI.0143-08.2008
Uemura, M., Refaat, M. M., Shinoyama, M., Hayashi, H., Hashimoto, N., and Takahashi, J. (2010). Matrigel supports survival and neuronal differentiation of grafted embryonic stem cell-derived neural precursor cells. J. Neurosci. Res. 88, 542–551. doi: 10.1002/jnr.22223
Valiente, M., and Marín, O. (2010). Neuronal migration mechanisms in development and disease. Curr. Opin. Neurobiol. 20, 68–78. doi: 10.1016/j.conb.2009.12.003
Wang, G., Ao, Q., Gong, K., Wang, A., Zheng, L., Gong, Y., et al. (2010a). The effect of topology of chitosan biomaterials on the differentiation and proliferation of neural stem cells. Acta Biomater. 6, 3630–3639. doi: 10.1016/j.actbio.2010.03.039
Wang, L. S., Chung, J. E., Chan, P. P. Y., and Kurisawa, M. (2010b). Injectable biodegradable hydrogels with tunable mechanical properties for the stimulation of neurogenesic differentiation of human mesenchymal stem cells in 3D culture. Biomaterials 31, 1148–1157. doi: 10.1016/j.biomaterials.2009.10.042
Wang, X., Hu, W., Cao, Y., Yao, J., Wu, J., and Gu, X. (2005). Dog sciatic nerve regeneration across a 30-mm defect bridged by a chitosan/PGA artificial nerve graft. Brain 128, 1897–1910. doi: 10.1093/brain/awh517
Wang, Y., Xu, Z., Kam, L. C., and Shi, P. (2014). Site-specific differentiation of neural stem cell regulated by micropatterned multicomponent interfaces. Adv. Healthcare Mater. 3, 214–220. doi: 10.1002/adhm.201300082
Webb, A., Clark, P., Skepper, J., Compston, A., and Wood, A. (1995). Guidance of oligodendrocytes and their progenitors by substratum topography. J. Cell Sci. 108, 2747–2760.
Weber, R. A., Breidenbach, W. C., Brown, R. E., Jabaley, M. E., and Mass, D. P. (2000). A randomized prospective study of polyglycolic acid conduits for digital nerve reconstruction in humans. Plastic Reconstruc. Surg. 106, 1036–1045. doi: 10.1097/00006534-200010000-00013
Wei, Z., Zhao, J., Chen, Y. M., Zhang, P., and Zhang, Q. (2016). Self-healing polysaccharide-based hydrogels as injectable carriers for neural stem cells. Sci. Rep. 6:37841. doi: 10.1038/srep37841
Wen, J. H., Vincent, L. G., Fuhrmann, A., Choi, Y. S., Hribar, K. C., Taylor-Weiner, H., et al. (2014). Interplay of matrix stiffness and protein tethering in stem cell differentiation. Nat. Mater. 13, 979–987. doi: 10.1038/nmat4051
Wilkinson, A. E., Kobelt, L. J., and Leipzig, N. D. (2014). Immobilized ECM molecules and the effects of concentration and surface type on the control of NSC differentiation. J. Biomed. Mater. Res. A 102, 3419–3428. doi: 10.1002/jbm.a.35001
Winter, C. C., Katiyar, K. S., Hernandez, N. S., Song, Y. J., Struzyna, L. A., Harris, J. P., et al. (2016). Transplantable living scaffolds comprised of micro-tissue engineered aligned astrocyte networks to facilitate central nervous system regeneration. Acta Biomater. 38, 44–58. doi: 10.1016/j.actbio.2016.04.021
Wood, M. D., and Sakiyama-Elbert, S. E. (2008). Release rate controls biological activity of nerve growth factor released from fibrin matrices containing affinity-based delivery systems. J. Biomed. Mater. Res. A 84, 300–312. doi: 10.1002/jbm.a.31269
Wrobel, M. R., and Sundararaghavan, H. G. (2013). Directed migration in neural tissue engineering. Tissue Eng. B Rev. 20, 93–105. doi: 10.1089/ten.teb.2013.0233
Wu, S., Xu, R., Duan, B., and Jiang, P. (2017). Three-dimensional hyaluronic acid hydrogel-based models for in vitro human iPSC-derived NPC culture and differentiation. J. Mater. Chem. B. 5, 3870–3878. doi: 10.1039/C7TB00721C
Xie, J., Liu, W., MacEwan, M. R., Bridgman, P. C., and Xia, Y. (2014). Neurite outgrowth on electrospun nanofibers with uniaxial alignment: the effects of fiber density, surface coating, and supporting substrate. ACS nano 8, 1878–1885. doi: 10.1021/nn406363j
Xie, J., Willerth, S. M., Li, X., Macewan, M. R., Rader, A., Sakiyama-Elbert, S. E., et al. (2009). The differentiation of embryonic stem cells seeded on electrospun nanofibers into neural lineages. Biomaterials 30, 354–362. doi: 10.1016/j.biomaterials.2008.09.046
Xing, D., Ma, L., and Gao, C. (2014). Synthesis of functionalized poly (ester carbonate) with laminin-derived peptide for promoting neurite outgrowth of PC12 cells. Macromol. Biosci. 14, 1429–1436. doi: 10.1002/mabi.201400186
Xing, D., Ma, L., and Gao, C. (2017). A bioactive hyaluronic acid–based hydrogel cross-linked by Diels–Alder reaction for promoting neurite outgrowth of PC12 cells. J. Bioact. Compat. Polym. 32:0883911516684654. doi: 10.1177/0883911516684654
Xu, X.-Y., Li, X.-T., Peng, S.-W., Xiao, J.-F., Liu, C., Fang, G., et al. (2010). The behaviour of neural stem cells on polyhydroxyalkanoate nanofiber scaffolds. Biomaterials 31, 3967–3975. doi: 10.1016/j.biomaterials.2010.01.132
Yamada, K. M. (1989). “Fibronectin domains and receptors,” in Fibronectin, ed D. Mosher (New York, NY: Academic Press), 47–121.
Yamada, Y., Hozumi, K., Aso, A., Hotta, A., Toma, K., Katagiri, F., et al. (2012). Laminin active peptide/agarose matrices as multifunctional biomaterials for tissue engineering. Biomaterials 33, 4118–4125. doi: 10.1016/j.biomaterials.2012.02.044
Yang, F., Murugan, R., Wang, S., and Ramakrishna, S. (2005). Electrospinning of nano/micro scale poly(l-lactic acid) aligned fibers and their potential in neural tissue engineering. Biomaterials 26, 2603–2610. doi: 10.1016/j.biomaterials.2004.06.051
Yang, K., Jung, H., Lee, H.-R., Lee, J. S., Kim, S. R., Song, K. Y., et al. (2014). Multiscale, hierarchically patterned topography for directing human neural stem cells into functional neurons. ACS Nano 8, 7809–7822. doi: 10.1021/nn501182f
Yang, K., Lee, J. S., Kim, J., Lee, Y. B., Shin, H., Um, S. H., et al. (2012). Polydopamine-mediated surface modification of scaffold materials for human neural stem cell engineering. Biomaterials 33, 6952–6964. doi: 10.1016/j.biomaterials.2012.06.067
Yao, S. L., Liu, X., Yu, S. K., Wang, X. M., Zhang, S. M., Wu, Q., et al. (2016). Co-effects of matrix low elasticity and aligned topography on stem cell neurogenic differentiation and rapid neurite outgrowth. Nanoscale 8, 10252–10265. doi: 10.1039/C6NR01169A
Zamani, F., Amani-Tehran, M., Latifi, M., and Shokrgozar, M. A. (2013). The influence of surface nanoroughness of electrospun PLGA nanofibrous scaffold on nerve cell adhesion and proliferation. J. Mater. Sci. Mater. Med. 24, 1551–1560. doi: 10.1007/s10856-013-4905-6
Zhang, D., Ni, N., Chen, J., Yao, Q., Shen, B., Zhang, Y., et al. (2015). Electrospun SF/PLCL nanofibrous membrane: a potential scaffold for retinal progenitor cell proliferation and differentiation. Sci. Rep. 5:14326. doi: 10.1038/srep14326
Zhang, K., Zheng, H., Liang, S., and Gao, C. (2016). Aligned PLLA nanofibrous scaffolds coated with graphene oxide for promoting neural cell growth. Acta Biomater. 37, 131–142. doi: 10.1016/j.actbio.2016.04.008
Zhang, Z., Yoo, R., Wells, M., Beebe, T. P., Biran, R., and Tresco, P. (2005). Neurite outgrowth on well-characterized surfaces: preparation and characterization of chemically and spatially controlled fibronectin and RGD substrates with good bioactivity. Biomaterials 26, 47–61. doi: 10.1016/j.biomaterials.2004.02.004
Zhao, S., Fan, W., Guo, X., Xue, L., Berninger, B., Salierno, M. J., et al. (2017). Microenvironments to study migration and somal translocation in cortical neurons. Biomaterials 156, 238–247. doi: 10.1016/j.biomaterials.2017.11.042
Zweckberger, K., Ahuja, C. S., Liu, Y., Wang, J., and Fehlings, M. G. (2016). Self-assembling peptides optimize the post-traumatic milieu and synergistically enhance the effects of neural stem cell therapy after cervical spinal cord injury. Acta Biomater. 42, 77–89. doi: 10.1016/j.actbio.2016.06.016
Keywords: biomaterials, neuroregeneration, axon growth, neurite guidance, nerve repair
Citation: Farrukh A, Zhao S and del Campo A (2018) Microenvironments Designed to Support Growth and Function of Neuronal Cells. Front. Mater. 5:62. doi: 10.3389/fmats.2018.00062
Received: 22 February 2018; Accepted: 05 October 2018;
Published: 02 November 2018.
Edited by:
Sara Pedron, University of Illinois at Urbana-Champaign, United StatesReviewed by:
Kirsten Haastert-Talini, Hannover Medical School, GermanyBin Duan, University of Nebraska Medical Center, United States
Copyright © 2018 Farrukh, Zhao and del Campo. This is an open-access article distributed under the terms of the Creative Commons Attribution License (CC BY). The use, distribution or reproduction in other forums is permitted, provided the original author(s) and the copyright owner(s) are credited and that the original publication in this journal is cited, in accordance with accepted academic practice. No use, distribution or reproduction is permitted which does not comply with these terms.
*Correspondence: Aránzazu del Campo, aranzazu.delcampo@leibniz-inm.de