- 1Key Laboratory of Quality and Safety Risk Assessment for Aquatic Products on Storage and Preservation (Shanghai), China Ministry of Agriculture, College of Food Science and Technology, Shanghai Ocean University, Shanghai, China
- 2Archaea Centre, Department of Biology, University of Copenhagen, Copenhagen, Denmark
Vibrio parahaemolyticus is a seafoodborne pathogen that can cause severe gastroenteritis and septicemia diseases in humans and even death. The emergence of multidrug-resistant V. parahaemolyticus leads to difficulties and rising costs of medical treatment. The bacterium of environmental origins containing no major virulence genes (tdh and trh) has been reported to be associated with infectious diarrhea disease as well. Identification of risk factors in V. parahaemolyticus is imperative for assuming food safety. In this study, we obtained secretomic and proteomic profiles of V. parahaemolyticus isolated from 12 species of commonly consumed aquatic products and identified candidate protein spots by using two-dimensional gel electrophoresis and liquid chromatography tandem mass spectrometry techniques. A total of 11 common and 28 differential extracellular proteins were found from distinct secretomic profiles, including eight virulence-associated proteins: outer membrane channel TolC, maltoporin, elongation factor Tu, enolase, transaldolase, flagellin C, polar flagellin B/D, and superoxide dismutase, as well as five antimicrobial and/or heavy metal resistance-associated ABC transporter proteins. Comparison of proteomic profiles derived from the 12 V. parahaemolyticus isolates also revealed five intracellular virulence-related proteins, including aldehyde-alcohol dehydrogenase, outer membrane protein A, alkyl hydroperoxide reductase C, phosphoenolpyruvate-protein phosphotransferase, and phosphoglycerate kinase. Additionally, our data indicated that aquatic product matrices significantly altered proteomic profiles of the V. parahaemolyticus isolates with a number of differentially expressed proteins identified. The results in this study meet the increasing need for novel diagnosis candidates of the leading seafoodborne pathogen worldwide.
Introduction
Vibrio parahaemolyticus is a gram-negative bacterium that thrives in marine, estuarine, and aquaculture environments worldwide (Ghenem et al., 2017). The bacterium is frequently detected from seafood such as shellfish, shrimp, and fish. Consumption of raw or inadequately cooked seafood, primarily oysters, can cause acute gastroenteritis in humans (Ghenem et al., 2017). Vibrio parahaemolyticus was initially identified in an outbreak of infectious diarrhea disease in 1950 in Osaka, Japan, caused by contaminated semidried juvenile sardines, which sickened 272 and killed 20 people (Fujino et al., 1965). Henceforth, outbreaks and prevalence of the infectious disease are reported in many countries in the world (Ghenem et al., 2017; Baker-Austin et al., 2018). In the United States, there were approximately 35,000 cases of acute gastroenteritis infected by V. parahaemolyticus per year from 2000 to 2008 (Baker-Austin et al., 2018). Data from foodborne disease outbreak reporting system in China showed that 42.3% of biohazard cases reported were attributed to V. parahaemolyticus from 2011 to 2016 (Liu et al., 2018). Vibrio parahaemolyticus has been the leading cause of infectious diarrhea disease, especially among adults in coastal regions in China (Yang C. et al., 2019). Recently, V. parahaemolyticus was reported to be associated with acute hepatopancreatic necrosis disease, a newly emerging shrimp disease, which severely damaged the global shrimp industry (Li et al., 2017). The global spread of V. parahaemolyticus underscores the need for a better understanding of virulence traits of the bacterium.
Most pathogenic V. parahaemolyticus strains have two major virulence genes (tdh and trh) encoding thermostable direct hemolysin (TDH) and TDH-related hemolysin. The former is a heat-resistant and pore-forming toxin composed of 156 amino acids, whereas the latter contains 189 amino acids and shares 54.8–68.8% identify in nucleotide level with the TDH (Zhang and Orth, 2013). Both these toxins have hemolytic activity, enterotoxin activity, cardiotoxicity, and cytotoxicity (Li et al., 2019). Nevertheless, approximately 90–99% of V. parahaemolyticus isolates of environmental origins were detected negative for the toxic factors (Raszl et al., 2016; Park et al., 2018). Previous studies have revealed some other cytotoxic factors to human gastrointestinal cells secreted by type III and type VI secretion systems (T3SS and T6SS) in V. parahaemolyticus (Makino et al., 2003). Two types of T3SSs (T3SS1 and T3SS2) were identified, of which T3SS1 located in chromosome I is necessary for the bacterial survival in the environment (Makino et al., 2003; De Nisco et al., 2017). Recent studies indicated that T3SS1 appeared to inject effectors, for example, VopQ, VopR, VopS, and VPA0450, directly into human gastrointestinal cells, which lead to the induction of rapid autophagy followed by cell rounding, eventually cell lysis (Osorio, 2018). The T3SS2 located on chromosome II plays a critical role in the enteropathogenicity of the bacterium (Makino et al., 2003). The effectors of T3SS2 can cause enterotoxicity by destroying the cell cytoskeleton, for example, VopC, VopL, VopV, and VopO, or manipulating cell signaling transduction, for example, VopA, VopT, VopZ, and VPA1380 (De Souza Santos et al., 2017; Osorio, 2018; Luo et al., 2019). Additionally, T6SS is a complex secretory device capable of secreting effectors into host mammalian cells as well as target bacterial cells (Li et al., 2019). It consists of a series of components including structural proteins, translocators, secreted proteins, and some other proteins with auxiliary function, for example, DotU, IcmF, ClpV, Hcp, VgrG, and PAAR (Salomon et al., 2014). Similar to T3SS, V. parahaemolyticus encodes two T6SSs (T6SS1 and T6SS2) located on chromosomes I and II, respectively. T6SS1 is active under warm marine-like conditions (3% NaCl, 30°C), whereas T6SS2 is active under low salinity and low temperature (1% NaCl, 23°C) (Salomon et al., 2013). Recent studies have indicated that T6SSs are tightly regulated in pathogenic bacteria, for example, Vibrio cholerae, Pseudomonas aeruginosa, and V. parahaemolyticus, and induced by external conditions and cues such as quorum sensing, salinity, temperature, mucin, chitin, surface sensing, and cell membrane damage (Ben-Yaakov and Salomon, 2019).
There is growing evidence to support that V. parahaemolyticus isolates lacking the virulence factors can cause infectious diarrhea disease in humans (Castillo et al., 2018). For example, Thongjun et al. (2013) reported that 9–10% of clinical isolates recovered from diarrhea patients were identified as non-toxigenic V. parahaemolyticus in South Thailand between 2001 and 2010. On the other hand, non-toxigenic V. parahaemolyticus isolates of environmental origins may possess additional pathogenicity mechanisms. The emergence of multidrug-resistant (MDR) V. parahaemolyticus increases the difficulty and cost of clinical treatment (Elmahdi et al., 2016). For instance, Hu and Chen (2016) reported that 74.5% of the V. parahaemolyticus strains (n = 208) isolated from 10 species of commonly consumed aquatic products in Shanghai, China, were resistant to more than three antimicrobial agents. Yang et al. (2017) isolated 98 V. parahaemolyticus strains from 504 seafood samples in 11 provinces of China and found that 68.38% of the isolates showed MDR phenotypes. Recently, Lopatek et al. (2018) reported that 55.8% of the V. parahaemolyticus isolates (n = 104) recovered from 595 samples collected from Denmark, France, Germany, Italy, the Netherlands, Norway, Poland, Spain, Sri Lanka, and Turkey had resistance to ampicillin and streptomycin and one isolate resistant to ampicillin, streptomycin, and ciprofloxacin. Therefore, identification of virulence and resistance-associated factors in V. parahaemolyticus is imperative for food safety systems, particularly in developing countries.
The combination of two-dimensional gel electrophoresis (2D-GE) and liquid chromatography tandem mass spectrometry (LC-MS/MS) techniques is widely used in current proteomics researches (Kim and Cho, 2019). The 2D-GE couples isoelectric focusing (IEF) in the first dimension and sodium dodecyl sulfate–polyacrylamide gel electrophoresis (SDS-PAGE) in the second dimension, which separate proteins according to isoelectric points and molecular masses, respectively. Several hundreds of individual protein abundances separated by 2D-GE can be quantified in the cell population or sample tissues. The LC-MS/MS is also a powerful tool that can efficiently separate and identify constituents in protein mixtures with high sensitivity and specificity (Aslam et al., 2017). For instance, based on the LC-MS/MS technique, Zhong et al. (2019) compared V. parahaemolyticus proteomes between the resuscitation state and viable but non-culturable state and found a total of 429 differentially expressed proteins mainly involved in cellular process, establishment of localization, and metabolic process. Pang et al. (2020) analyzed 102 acetylation modified proteins of Vibrio alginolyticus HY9901 by LC-MS/MS and identified five virulence factors, including HemL, FabB, FabD, FabF-3, and PhoR.
The People’s Republic of China is the world’s largest producer, consumer, and exporter of aquatic products, including fish, crustaceans, and shellfish (Hu and Chen, 2016). Data from China Fishery Statistical Yearbook (2018) showed that shellfish production (14,371,304 tons) is an important part of maricultural production in China, and the main species include Ostrea gigas thunberg (known as oyster) (34.0%), Ruditapes philippinarum, Mactra veneriformis, and Paphia undulate (known as clam) (29.1%), Placopecten magellanicus (known as scallop) (14.0%), Perna viridis (known as mussel) (6.5%), and Solen strictus (known as razor clam) (6.0%). Additionally, Aristichthys nobilis (known as bighead carp) and Ctenopharyngodon idellus (known as grass carp) are commonly consumed freshwater fish in China, accounting for 18.4% (5,345,641 tons) and 10.7% (3,097,952 tons) of the total freshwater aquaculture production (29,052,930 tons), respectively. The production of Litopenaeus vannamei (known as white-leg shrimp) was 1,672,287 tons, which was the most predominant among the crustaceans in 2017 (Zhang et al., 2018). Oratosquilla oratoria (known as mantis shrimp) is one of the most economically important species of Pacific shrimp, and its output was 219,087 tons in 2017 (Zhang et al., 2018). Continuous monitoring of food contaminants and identification of risk factors are crucial to safeguard the food supply chain (Chen and Alali, 2018). In our prior researches, V. parahaemolyticus contamination in 10 species of aquatic products was surveyed (He et al., 2016; Hu and Chen, 2016). Secretomic profiles of seven V. parahaemolyticus strains of two clinical and five aquatic product origins (Haliotis asinina, Moerella iridescens, Metapenaeus ensis, Exopalaemon carinicauda, and L. vannamei) were compared, and six virulence-associated proteins involved in bacterial pathogenicity were identified (He et al., 2015). Recently, a number of V. parahaemolyticus isolates from 25 species of commonly consumed shellfish, crustacean, and fish samples were isolated and characterized (Su et al., unpublished). Among these, 12 V. parahaemolyticus isolates recovered from eight shellfish (M. veneriformis, O. gigas thunberg, P. undulate, P. viridis, P. magellanicus, R. philippinarum, Sinonovacula constricta, and S. strictus), two crustacean (L. vannamei and O. oratoria), and two fish (A. nobilis and C. idellus) had multiple resistance to antimicrobial agents and heavy metals. The objectives of this study were (1) to characterize genetic diversity of the V. parahaemolyticus isolates recovered from 12 species of aquatic products; (2) to take advantage of 2D-GE and LC-MS/MS technologies to determine secretomic and proteomic profiles of the 12 V. parahaemolyticus isolates and to identify common and/or differential extracellular and intracellular proteins by comparative secretomic and proteomic analysis of the isolates from three kinds of aquatic products; and (3) to study implications of various aquatic product matrices on proteomics of the V. parahaemolyticus isolates. The secretomes and proteomes between the V. parahaemolyticus isolates and pathogenic reference strains were compared as well. To our knowledge, very little of such information in V. parahaemolyticus recovered from all the species (except L. vannamei) was available to date. The results in this study support the increasing need for novel sites and targets for food safety control of V. parahaemolyticus contamination in aquatic products.
Materials and Methods
V. parahaemolyticus Strains and Culture Conditions
Vibrio parahaemolyticus strains used in this study are listed in Table 1, and their genotypes and phenotypes were characterized by Su et al. (under review). Vibrio parahaemolyticus strains were individually inoculated from −80°C stock in our laboratory in Shanghai Ocean University and incubated in tryptic soy broth (TSB) medium (pH 8.5, 3.0% NaCl; Beijing Land Bridge Technology Co., Ltd., Beijing, China) at 37°C. Bacterial cell cultures grown at mid-logarithmic phase with OD600nm values ranging from 0.5 to 0.6 without shaking were collected for extracellular protein extraction, whereas those at late-logarithmic phase with OD600nm values ranging from 0.8 to 1.0 with shaking at 180 revolutions/min (rpm) were used for intracellular protein extraction (He et al., 2015). Vibrio parahaemolyticus ATCC33847 (tdh+ trh–) and ATCC17802 (tdh– trh+) were used as standard strains (Table 1).
Enterobacterial Repetitive Intergenic Consensus—Polymerase Chain Reaction Assay
Bacterial genomic DNA was extracted by a thermal lysis method as previously described (Xu et al., 2019). The Enterobacterial Repetitive Intergenic Consensus (ERIC) primers ERIC-F (5′-ATGTAAGCTCCTGGGGATTCAC-3′) and ERIC-R (5′-AAGTAAGTGACTGGGGTGAGCG-3′) (Rivera et al., 1995) were synthesized by Sangon Biotech Co., Ltd., Shanghai, China. A 20 μL of ERIC–polymerase chain reaction (PCR) reaction solution contained 6 μL sterile DNase/RNase-free deionized H2O (Tiangen Biotech Co., Ltd., Beijing, China), 1 μL of each primer (0.25 mM), 10 μL 2 × Taq PCR master mix (Novoprotein Scientific lnc., Shanghai, China), and 2 μL DNA template. The ERIC-PCRs was performed using Mastercycler® pro PCR thermal cycler (Eppendorf Corporate, Hamburg, Germany) under the following conditions: denaturation at 95°C for 8 min, 32 cycles of 95°C for 30 s, 52°C for 1 min, and 65°C for 8 min and a final extension of 65°C for 16 min. The amplified products were analyzed by electrophoresis with 1.0% (wt/vol) agarose gels [Biowest Agarose, Spain (Origin), distributed by Shanghai Fushen Bio-Technology Co., LTD., Shanghai, China] and imaged using UVP EC3 Imaging system (UVP LLC, Upland, CA, United States). Fingerprint patterns were analyzed using BioNumerics 7.6 software, and the clustering was deduced based on the unweighted pair group with arithmetic average (UPGMA) algorithm (Xu et al., 2019).
Extraction of Extracellular and Intracellular Proteins of V. parahaemolyticus Isolates
Extracellular proteins of V. parahaemolyticus isolates were extracted according to the method described previously (He et al., 2015) with minor modifications. Briefly, growth curves of V. parahaemolyticus isolates incubated in the TSB medium (pH 8.5, 3% NaCl) at 37°C were determined using Bioscreen Automatic Growth Curve Analyzer (BioTek Instruments, Inc., Winooski, VT, United States). A 20 μL of Bacterial Protease Inhibitors Complex (Sangon) was added to each 100 mL of bacterial cell culture at midlogarithmic phase, which was then centrifuged at 6,000 g for 10 min at 4°C. The supernatant was filtered through 0.22-μm-pore-size membrane filters (Millipore, Bedford, MA, United States) to remove residual bacterial cells. The filtrate was precipitated by adding ice-cold trichloracetic acid (Sinopharm Chemical Reagent Co., Ltd., Shanghai, China) to a final concentration of 10% (vol/vol) and then incubated on ice for 5 h. The extracellular proteins were collected by centrifugation at 12,000 rpm for 30 min at 4°C. The resulting protein pellet was washed with ice-cold acetone (Sinopharm) for three times, air-dried and stored at −80°C for further analysis.
For the extraction of intracellular proteins, V. parahaemolyticus isolates were individually incubated in the TSB medium (pH 8.5, 3.0% NaCl) to late-logarithmic phase at 37°C with shaking. Bacterial cell cultures were collected as described above, and intracellular proteins were extracted using the Bacterial Protein Extraction Kit (Sangon) containing protease inhibitors, according to the manufacturer’s instructions. Protein concentration was measured using Bradford Protein Assay Kit (Sangon) with bovine serum albumin as the standard protein.
The 2D-GE Assay
The 2D-GE was performed according to the method described previously (He et al., 2015) with minor modifications. Briefly, extracellular protein pellet was dissolved with 100 μL of lysis buffer [8 M urea (Sangon), 4% (wt/vol) 3-[3-cholamidopropyldimethylammonio-1-propanesulfonate (CHAPS; Sangon), 65 mM dithiothreitol (DTT; Sangon), 0and.2% (vol/vol) Bio-Lyte 3/10 ampholyte (Bio-Rad Laboratories Inc., Hercules, CA, United States)] and then centrifuged at 12,000 rpm for 10 min at room temperature to remove undissolved residues. Isoelectric focusing was performed with the ready immobilized pH gradient gel (IPG) strips (Bio-Rad). Approximately 20 μg of extracellular proteins was diluted with the rehydration buffer [8 M urea, 4% (wt/vol) CHAPS, 65 mM DTT, 0.2% (vol/vol) Bio-Lyte 3/10 ampholyte, and 0.0001% (wt/vol) bromophenol blue (Sangon)] to a final volume of 200 μL per sample. The mixture of each sample was applied to the strips (pH 4–7, 7 cm; Bio-Rad) and passive rehydrated for 16 h at 17°C. After rehydration, IEF was run with a 6-step program: 100 V for 3 h with slow ramping; 250 V for 1 h with slow ramping; 500 V for 1 h with linear ramping; 4,000 V for 3 h with linear ramping; and 4,000 V with rapid ramping until 20,000 V-hour was reached. Following the electrophoresis in the first dimension, the strips were first equilibrated for 15 min in equilibration buffer I [6 M urea, 37.5 mM Tris-HCl (pH 8.8, Sangon), 20% glycerol (Sangon), 2% SDS (Sangon), and 2% DTT] and then washed for another 15 min with equilibration buffer II [6 M urea, 37.5 mM Tris-HCl at pH 8.8, 2% SDS, 20% glycerol, 2.5% (wt/vol) iodoacetamide (Sangon)]. The second-dimension separation was performed by SDS-PAGE. The strips were individually transferred onto 12.5% separation gel using a Mini-PROTEANW electrophoresis cell (Bio-Rad) with a 2-step program: 5 mA for 20 min, and 15 mA for 85 min.
For intracellular proteins, an aliquot of each 400 μL of protein sample was individually applied to the strips (pH 4–7, 17 cm) (Bio-Rad) and passive rehydrated for 17 h at 17°C. After rehydration, IEF was run with a 9-step program: 50, 100, 500, and 1,000 V for 1 h with slow ramping, respectively; 2,000 and 4,000 V for 1 h with linear ramping, respectively; 6,000 and 8,000 V for 1 h with rapid ramping, respectively; and 10,000 V with rapid ramping until 80,000 V-hour was reached. The strips were individually transferred onto 12.5% separation gel using PROTEAN II XL electrophoresis cell (Bio-Rad) with a 2-step program: 5 mA for 5 h and 15 mA for 6 h.
After electrophoresis, the gels were stained using Protein Stains K (Sangon), according to the manufacturer’s instructions. Silver-stained gels were scanned using GenoSens 1800 Series Gel Documentation and Analysis System (Clinx Science Instruments Co. Ltd., Shanghai, China). Protein spot detection, spot matching, and quantitative intensity analysis were performed using PDQuest Advanced-8.0.1 software (Bio-Rad).
LC-MS/MS Analysis
The LC-MS/MS analysis was carried out at HooGen Biotech, Shanghai, China. The visible and discriminative protein spots were individually excised from 2D-GE gels, and gel pieces were dried under vacuum. A 15 μL of digestion solution (25 mM ammonium bicarbonate) containing trypsin (12.5 ng/mL; Promega, Madison, WI, United States) was added to each protein spot sample tube and incubated with gentle shaking at 37°C for 16 h. The resulting peptides were identified by Q Exactive Mass Spectrometer [Thermo Fisher Scientific (TFS), Waltham, MA, United States] coupled with Easy nLC 1200 Chromatography System (TFS). The peptide mixture was loaded onto C18-reversed phase column (15 cm long, 75-μm inner diameter) packed in-house with RP-C18 5 μm resin in buffer A [0.1% formic acid (Sigma-Aldrich, St. Louis, MO, United States) in high-performance liquid chromatography–grade water] and separated with a linear gradient of buffer B [0.1% formic acid in 84% acetonitrile (Sigma-Aldrich, United States)] at a flow rate of 250 nL/min over 60 min. The collected LC-MS/MS data files were converted to Mascot generic format (mgf) and then imported into Mascot version 2.2 server (Matrix Science, London, United Kingdom) for automated peptide identification using UniProt Vibrio parahaemolyticus database (download in September 2019; 89189 sequences). The criteria for peptide matching and protein calls included enzyme: trypsin; max missed cleavage: 2; fixed modifications: carbamidomethyl (C); variable modification: oxidation (M); peptide mass tolerance: ± 20 ppm; fragment MS/MS tolerance: 0.1 Da; Mascot score: ≥20; ion score: >20; and false discovery rate: <0.01 at peptide and protein level. Common protein spots on secretomic profiles were marked with blue circles, whereas differential protein spots on secretomic and proteomic profiles were marked in red numbers. The identified proteins were subjected to Generic Gene Ontology (GO) Term Finder1 for GO analysis (Yang et al., 2015).
Preparation of Aquatic Product Matrix Media
Aquatic product matrices were prepared according to the method described by Chaitiemwong et al. (2010) with minor modifications. Briefly, 12 species of fresh aquatic products were collected in two local fish markets [Guzong road fish market (30°53′11.34″N, 121°55′3.09″E) and Luchaogang seafood market (30°51′34.47″N, 121°51′3.15″E)] in Shanghai and transferred in sterile sealed plastic bags (Nanjing Maojie Microbial Technology Co., Ltd., Nanjing, China) in an ice box (700 × 440 × 390 mm) to the laboratory in Shanghai Ocean University for analysis immediately. A 10 g (wet weight) fresh meat of each sample was aseptically placed in a homogeneous bag (Sangon) with 40 mL sterile water and homogenized for 3 min using a laboratory blender BagMixer (Shanghai Jingxin Industry Development Co., Ltd., Shanghai, China). The mixture was centrifuged at 8,000 g for 20 min, and the supernatant was filtered through 0.22-μm-pore-size membrane filters (Millipore). The filtrate of each aquatic product sample was supplemented into the TSB medium (pH 8.5, 3% NaCl) to produce corresponding aquatic product matrix medium with a final concentration of 1% (vol/vol).
Measurement of Compositions of Aquatic Product Matrices
Total protein content was measured using Bradford Protein Assay Kit (Sangon) according to the manufacturer’s instructions. Crude fat content was determined by the automated Soxhlet method described by Shin et al. (2013) with minor modifications. Briefly, a 5 mL of aquatic product matrix was individually weighed and dried in a Petri dish at 104°C for 3 h. The dried products were wiped with cotton balls moistened with petroleum ether (Shanghai Titan Scientific Co., Ltd., Shanghai, China). Crude fat on cotton balls was extracted by an automated Soxtherm Fat Extraction System (Gerhardt, Bonn, Germany) according to the manufacturer’s instructions. The extracted products remaining in a weighed beaker were dried at 104°C for 30 min and cooled in a dessicator to room temperature. Crude fat in the beakers was weighed and calculated. Carbohydrate (saccharides) content was measured by the phenol–sulfuric acid method described by Albalasmeh et al. (2013) with minor modifications. A 1 mL of each sample was mixed with 1 mL of 5% (wt/wt) aqueous solution of redistilled phenol (Shanghai Macklin Biochemical Co., Ltd., Shanghai, China) in a glass tube (Φ15 mm, 150 mm). A 5 mL of sulfuric acid (95–98%; Shanghai Kuling Fine Chemical Co., Ltd., Shanghai, China) was added into the mixture and then incubated in a boiling water bath for 20 min. After cooling in ice for 2 min and at room temperature for 15 min, the absorption of the reaction mixture at 490 nm was measured using BioTek Synergy 2. Carbohydrate concentration was calculated according to a standard curve prepared with glucose (Sinopharm).
Reverse Transcription–PCR Assay
The reverse transcription (RT)–PCR assay was performed according to the method described by Zhu et al. (2017). Briefly, total RNA was extracted using RNeasy Mini Kit (Qiagen, Hilden, Germany) following the manufacturer’s instructions. The DNA was removed from the samples with the RNase-Free DNase Set (Qiagen). The RT reactions were performed using PrimeScriptTM RT reagent Kit with gDNA Eraser [Takara Biomedical Technology (Beijing) Co., Ltd., Beijing, China]. Relative quantitative PCRs were performed using TB Green® Premix Ex TaqTM II kit (Tli RNaseH Plus, Beijing, China) according to the manufacturer’s instructions. All RT-PCRs were carried out in a 7500 Fast Real-Time PCR System (Applied Biosystems, Foster City, CA, United States) under the following conditions: initial denaturation at 50°C for 2 min and at 95°C for 10 min, followed by 40 cycles of denaturation at 95°C for 15 s and primer annealing at 60°C for 1 min. The 16S RNA was used as the internal reference gene as described previously. The primers (Supplementary Table S1) were designed by Premier 5.0 software2 and synthesized by Sangon (Shanghai, China). In a 20 μL of RT-PCR solution, 1,000, 200, 20, 2, 0.2, and 0.0 ng of cDNA template was individually added for standard curve preparation. The PCR efficiency was 90.0–100.0%, and r2 > 0.980 for the primers. All tests were performed in triplicate in this study.
Results
Genetic Diversity of the V. parahaemolyticus Isolates Recovered From 12 Species of Aquatic Products
The V. parahaemolyticus isolates used in this study were recovered from 12 species of commonly consumed aquatic products, including eight shellfish, two crustaceans, and two fish (Table 1). All the isolates were negative for the toxin genes (tdh and trh), but resistant to three antimicrobial agents, including rifampin (RIF), ampicillin (AMP), and streptomycin (STR). Approximately 75% (9/12) and 42% (5/12) of the V. parahaemolyticus isolates were also resistant to kanamycin (KAN) and tetracycline (TET), respectively. Additionally, different heavy metal tolerance profiles of the V. parahaemolyticus isolates were observed. Remarkably, V. parahaemolyticus CHN-N3-2 isolate recovered from the shellfish P. undulate had tolerance to five heavy metals Cd2+, Cu2+, Hg2+, Pb2+, and Zn2+. The heavy metal tolerance trend of the 12 V. parahaemolyticus isolates was Hg2+ = Zn2+ > Cd2+ > Cu2+ > Cr3+ > Pb2+ (Table 1).
Evolutionary relatedness of the V. parahaemolyticus strains was examined by the ERIC-PCR assay. This analysis revealed that all the tested strains were classified into 14 different ERIC genotypes, and the UPGMA algorithm grouped the ERIC genotypes into five distinct clusters (Clusters A–E) (Figure 1). Vibrio parahaemolyticus ATCC33847 (tdh+ trh–) and ATCC17802 (tdh– trh+) strains of clinical origin fell into Cluster B, whereas the remaining of aquatic product origins fell into Clusters A, C, D, and E. Vibrio parahaemolyticus isolates recovered from three shellfish (O. gigas thunberg, P. undulate, and S. constricta), two crustaceans (L. vannamei and O. oratoria), and two fish (A. nobilis and C. idellus) were grouped into the largest Cluster D with 7 ERIC genotypes, whereas Cluster A contained only the V. parahaemolyticus CHN-N3-2 isolate that had tolerance to 4 antimicrobial agents and 5 heavy metals. The Hg and AMP/KAN/RIF/STR resistance profile was the most predominant among the strains tested (Figure 1). These results demonstrated genetic diversity of the V. parahaemolyticus isolates recovered from the 12 species of commonly consumed aquatic products.
Distinct Secretomic Profiles of the V. parahaemolyticus Isolates
The supernatant of the V. parahaemolyticus cultures at midlogarithmic phases was collected, and extracellular proteins were extracted and analyzed by 2D-GE. This analysis uncovered distinct secretomic profiles of the 12 V. parahaemolyticus isolates of various aquatic product origins (Figure 2). The patterns yielded from three independent 2D-GE gels of each isolate were consistent (figures not shown). Based on the consensus patterns, 11 extracellular protein spots (marked with blue circles, Figure 2) were observed at similar locations on all the 2D-GE patterns derived from the 12 V. parahaemolyticus isolates. Notably, the V. parahaemolyticus CHN-B2-28 isolate recovered from R. philippinarum appeared to secret more extracellular proteins (35) than the other isolates (17–30). Additionally, a total of 28 differential extracellular proteins (marked with different numbers in red, Figure 2) were found from the distinct secretomic profiles. All common protein spots and the remaining differential protein spots among the 12 V. parahaemolyticus isolates were excised from the 2D-GE gels and digested with the trypsin. The resulting peptides were further identified by the LC-MS/MS analysis.
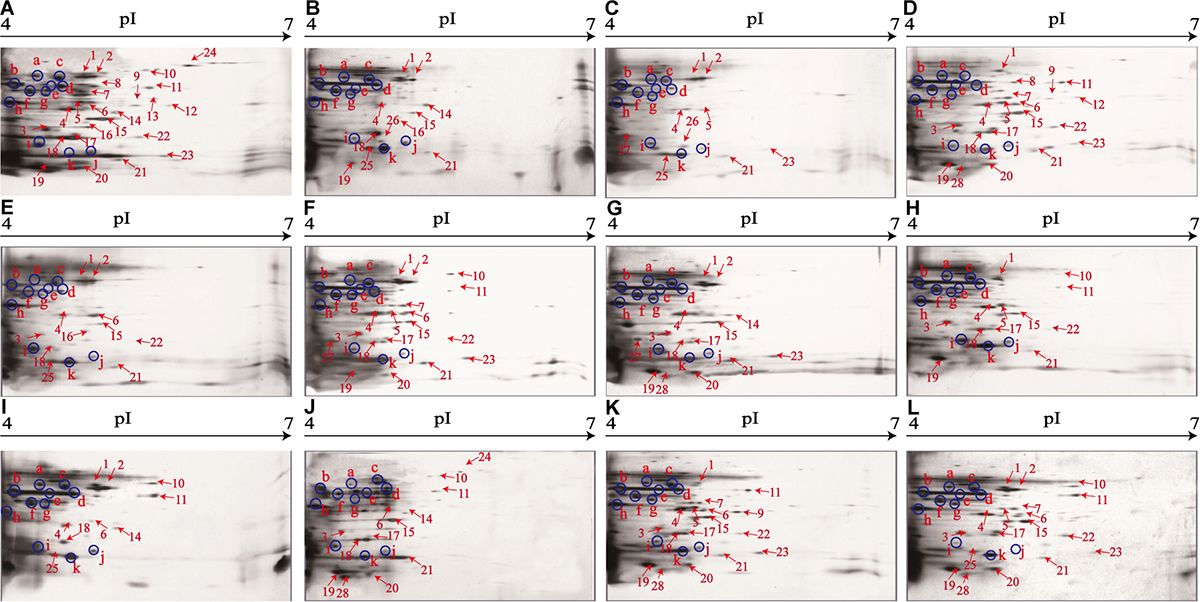
Figure 2. The secretomic profiles of the 12 V. parahaemolyticus isolates by the 2D-GE analysis. (A–L) V. parahaemolyticus CHN-B2-28, CHN-B5-29, CHN-B6-62, CHN-B8-26, CHN-N3-2, CHN-N4-18, CHN-N8-5, CHN-N10-18, CHN-N1-56, CHN-N2-5, CHN-L7-40, and CHN-Q5-1 isolates, respectively. The common protein spots marked with blue circles and the differential protein spots marked with different numbers in red were characterized by the LC-MS/MS analysis. pI, isoelectric point.
Identification of Common and Differential Extracellular Proteins of the V. parahaemolyticus Isolates
In this study, the LC-MS/MS technique was used to identify all common and differential extracellular proteins of the V. parahaemolyticus isolates. Based on the secretomic profiles, 11 common extracellular proteins shared among the 12 isolates were successfully obtained (Table 2). Of these proteins, the majority (n = 7) were cell membrane and periplasm components, including TolC protein (Spot a), maltoporin (Spot b), maltodextrin-binding protein (Spot d), putrescine-binding periplasmic protein (Spot f), gram-negative porin family protein (Spot h), outer membrane protein W (OmpW, Spot j), and basal-body rod modification protein FlgD (Spot i). For the others (n = 4), the Spot c was identified as elongation factor Tu (EF-Tu). Spot e was phosphoglucosamine mutase (PNGM) involved in carbohydrate metabolism and peptidoglycan biosynthetic pathway, whereas Spot g was fructose-bisphosphate aldolase that also acts as a transcriptional regulator in pathogenic Francisella (Ziveri et al., 2017). Additionally, the Spot k matched an uncharacterized protein encoded by the H320_01320 gene of V. parahaemolyticus 49 strain (GenBank: AONA00000000.1) with currently unknown function in public databases (Table 2).
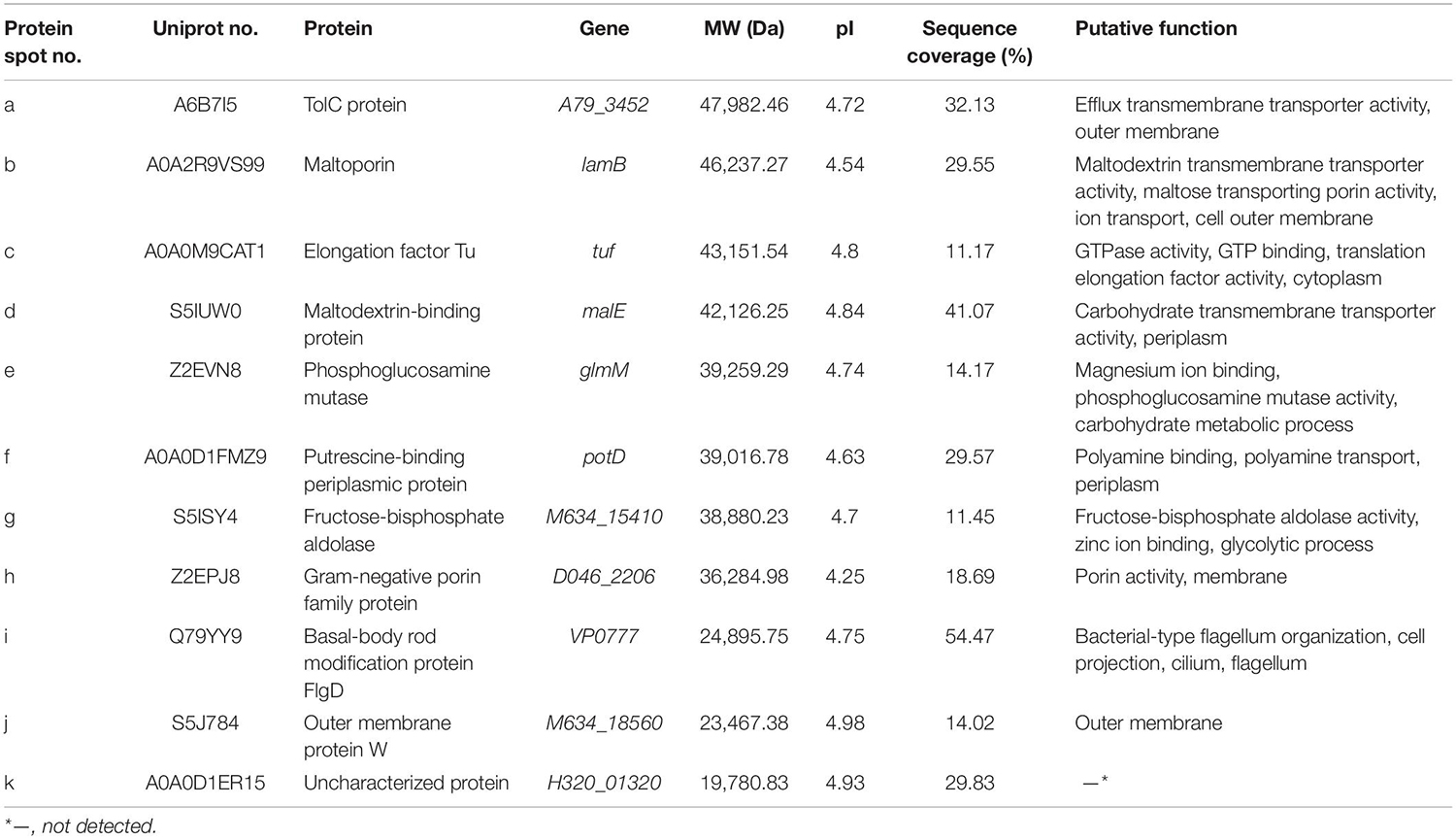
Table 2. Identification of the common protein spots on the secretomic profiles of the 12 V. parahaemolyticus isolates by LC-MS/MS analysis.
A total of 28 differential extracellular proteins were also successfully identified and listed in Table 3. The metabolism-related proteins (n = 7) constituted the largest proportion of the identified proteins, including three glycolysis-related proteins: enolase (Spot S2), phosphofructokinase (PFK) (Spot S6), and glyceraldehyde-3-phosphate dehydrogenase (GAPDH, Spot S13); one carbohydrate metabolism-related protein: transaldolase (Spot S5); one protein catabolism-related protein: ATP-dependent zinc metalloprotease (FtsH, Spot S10); one tricarboxylic acid cycle-related protein: succinate dehydrogenase iron–sulfur subunit (SDISS, Spot S17); and one polyphosphate metabolism-related protein: inorganic pyrophosphatase (PPase, Spot S25). Moreover, five differential extracellular proteins were involved in amino acid and nucleotide biosynthesis. For example, Spots S3, S11, and S22 were identified as 4-hydroxy-tetrahydrodipicolinate synthase (DHDPS), aspartate-semialdehyde dehydrogenase (ASD), and elongation factor Ts (EF-Ts) in amino acid biosynthesis, respectively, whereas Spots S1 and S9 were adenylosuccinate synthetase (AdSS) and ribose-phosphate pyrophosphokinase in nucleotide biosynthesis, respectively. Notably, two bacterial flagellin structural proteins were identified, including flagellin C (Spot S7) and polar flagellin B/D (Spot S8). In addition, Spot S27 matched an uncharacterized protein encoded by the E4P16_05165 gene of V. parahaemolyticus MAVP-R strain (GenBank: CP022553.2).
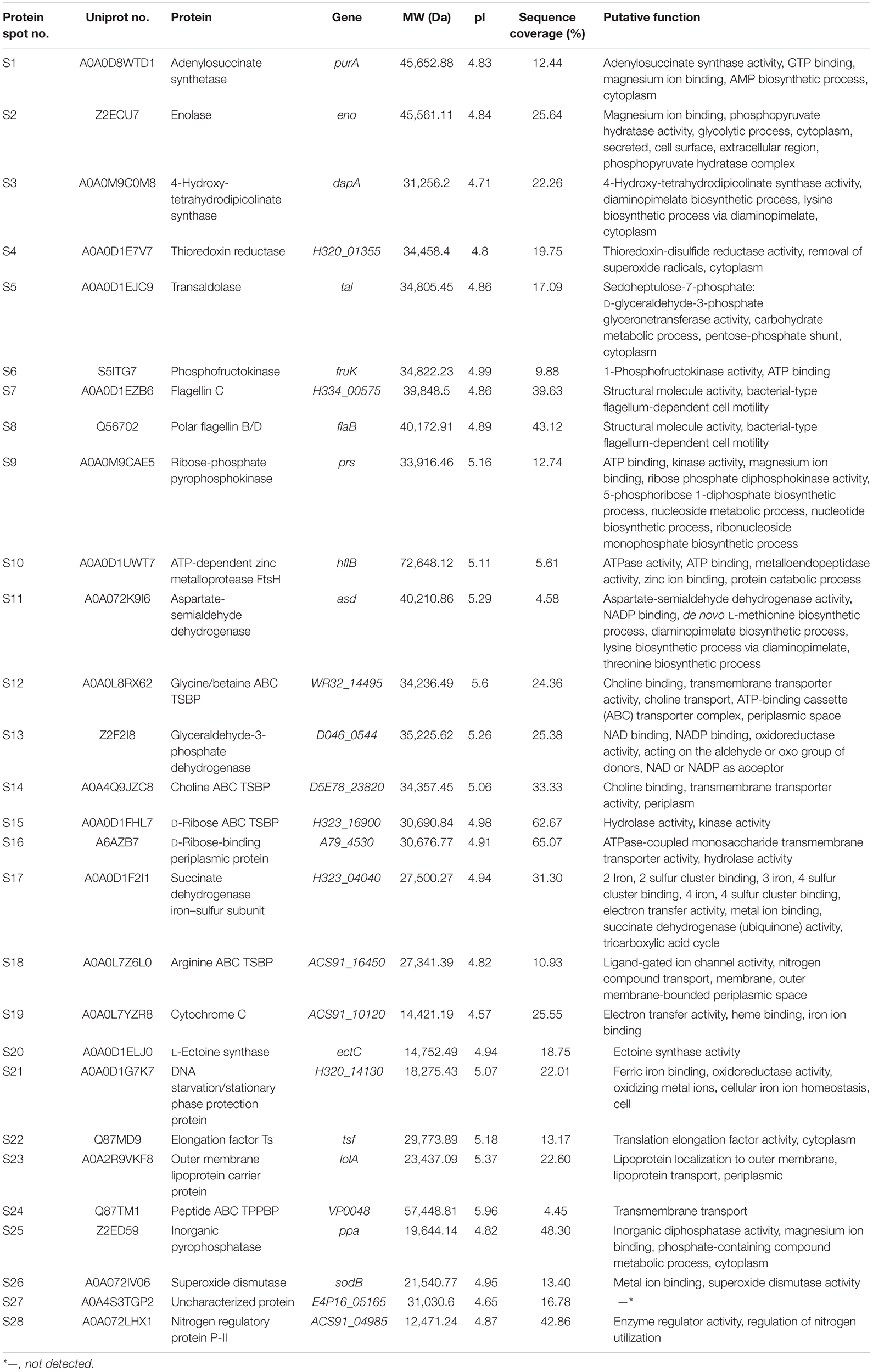
Table 3. Identification of the differential protein spots on the secretomic profiles of the 12 V. parahaemolyticus isolates by LC-MS/MS analysis.
Interestingly, five differential extracellular proteins were identified as ATP-binding cassette (ABC) transporters that constitute one of the largest families of membrane proteins (Theodoulou and Kerr, 2015), including glycine/betaine ABC transporter substrate-binding protein (TSBP) (Spot S12), choline ABC TSBP (Spot S14), D-ribose ABC TSBP (Spot S15), arginine ABC TSBP (Spot S18), and peptide ABC transporter periplasmic peptide-binding protein (TPPBP) (Spot S24). Moreover, the Spot S16 was D-ribose-binding periplasmic protein that has ATPase-coupled monosaccharide transmembrane transporter activity, whereas Spot S23 matched outer membrane lipoprotein carrier protein.
Remarkably, in this study, several identified extracellular proteins of the V. parahaemolyticus isolates have been reported to be involved in bacterial pathogenesis, including Spots a, b, c, S2, S5, S7, S8, and S26. The former three proteins TolC (Spot a), maltoporin (Spot b), and EF-Tu (Spot c) were secreted by all the isolates, and more than half of the isolates also secreted the enolase (Spot S2) and transaldolase (Spot S5). In contrast, only a few isolates secreted the flagellin C (Spot S7), polar flagellin B/D (Spot S8), and superoxide dismutase (Spot S26). For instance, the latter was only observed on the secretomic profiles derived from the CHN-B5-29 and CHN-B6-62 isolates recovered from P. magellanicus and S. constricta, respectively. Among all the V. parahaemolyticus isolates of aquatic product origins, the CHN-B2-28 isolate from R. philippinarum appeared to secret most of the extracellular virulence-associated proteins compared to the other isolates.
Secretomic Comparison of the V. parahaemolyticus Isolates From Three Kinds of Aquatic Products
The secretomic profiles derived from the V. parahaemolyticus isolates of the shellfish, crustaceans, and fish origins were different. For instance, there were 14 common and 14 differential extracellular proteins identified from the secretomic profiles of the V. parahaemolyticus CHN-B2-28, CHN-B5-29, CHN-B6-62, CHN-B8-26, CHN-N3-2, CHN-N4-18, CHN-N8-5, and CHN-N10-18 isolates recovered from the eight species of shellfish (Figures 2A–H). Significantly, there were six extracellular proteins appeared only on the secretomic profile of the shellfish origin, including the polar flagellin B/D (Spot S8), glycine/betaine ABC TSBP (Spot S12), GAPDH (Spot S13), D-ribose-binding periplasmic protein (Spot S16), superoxide dismutase (Spot S26), and an uncharacterized protein (Spot S27) (Table 3).
Comparison of the secretomic profiles of the V. parahaemolyticus isolates from two crustaceans revealed that the V. parahaemolyticus CHN-N2-5 isolate recovered from L. vannamei secreted more abundant proteins than the CHN-N1-56 isolate from O. oratoria (Figures 2I,J). A total of 16 common and 12 differential extracellular proteins on the secretomic profiles of these two isolates were identified. Spots S1, S2, S4, and S25, identified as AdSS, enolase, thioredoxin reductase (TrxR), and PPase, respectively, were secreted only by the CHN-N1-56 isolate, whereas eight proteins were secreted by the CHN-N2-5 isolate, including the DHDPS (Spot S3), D-ribose ABC TSBP (Spot S15), SDISS (Spot S17), cytochrome C (Spot S19), L-ectoine synthase (Spot S20), DNA starvation/stationary phase protection protein (Spot S21), peptide ABC TPPBP (Spot S24), and nitrogen regulatory protein P-II (Spot S28).
The numbers of extracellular proteins secreted by the V. parahaemolyticus CHN-L7-40 and CHN-Q5-1 isolates of the fish origin were similar. A total of 26 common and five differential extracellular proteins on the secretomic profiles of these two isolates were identified. For instance, Spots S9 and S18, identified as ribose-phosphate pyrophosphokinase and arginine ABC TSBP, respectively, were shown only on the secretomic profile of the CHN-L7-40 isolate from A. nobilis (Figure 2K), whereas Spots S2, S10, and S25, identified as enolase, ATP-dependent zinc metalloprotease FtsH, and PPase, respectively, were secreted only by the CHN-Q5-1 isolate from C. idellus (Figure 2L).
Secretomic Comparison of the V. parahaemolyticus Isolates With Pathogenic Reference Strains
The secretomic profiles of the V. parahaemolyticus ATCC33847 and ATCC17802 strains of clinical origin were also obtained for comparative secretomic analysis, although they have been reported in our prior research (He et al., 2015). One interesting observation was that the toxic ATCC33847 and ATCC17802 strains appeared to secret more extracellular proteins (59–62) than the V. parahaemolyticus isolates recovered from the 12 species of aquatic products (17–35), consistent with our prior results (He et al., 2015). Comparative secretomic analysis revealed that approximately 36.4% (4/11) of the common and 42.9% (12/28) of the differential extracellular proteins secreted by the 12 V. parahaemolyticus isolates were observed at similar locations on the secretomic profiles of the ATCC33847 and the ATCC17802 strains (Supplementary Figure S1). For instance, four extracellular proteins secreted by all the V. parahaemolyticus strains of aquatic product and clinical origins included the maltoporin (Spot b), EF-Tu (Spot c), maltodextrin-binding protein (Spot d), and PNGM (Spot e).
Distinct Proteomic Profiles of the V. parahaemolyticus Isolates
The 2D-GE and LC-MS/MS techniques were also used to analyze proteomics of the V. parahaemolyticus isolates recovered from the 12 species of aquatic products, and the obtained proteomic profiles are presented in Figure 3. The patterns yielded from three independent 2D-GE gels of each isolate were consistent (figures not shown). This analysis also revealed distinct proteomic profiles showing various visible differential protein spots (343–312) among the 12 V. parahaemolyticus isolates (Figures 3A–L). The V. parahaemolyticus CHN-N4-18 isolate recovered from P. viridis appeared to express the highest number of intracellular proteins (343) among all the isolates (Figure 3F). In contrast, approximately 312 intracellular proteins were observed from the proteomic profile of the CHN-B8-26 isolate from S. strictus (Figure 3D). Notably, for the crustaceans origin, the CHN-N1-56 isolate from L. vannamei expressed much more intracellular proteins (342, Figure 3I) than the CHN-N2-5 isolate from O. oratoria (314, Figure 3J).
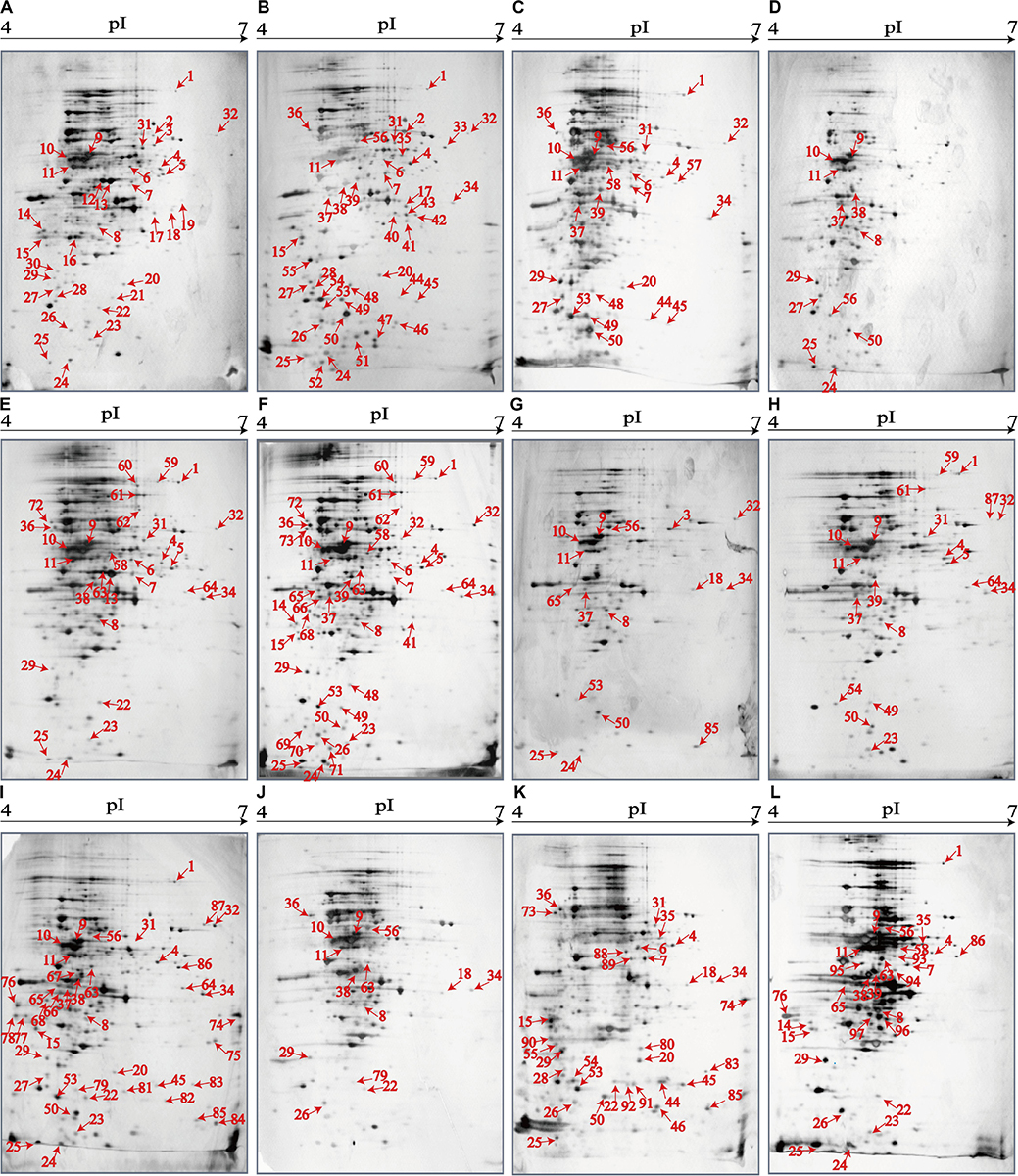
Figure 3. The proteomic profiles of the 12 V. parahaemolyticus isolates by the 2D-GE analysis. (A–L) V. parahaemolyticus CHN-B2-28, CHN-B5-29, CHN-B6-62, CHN-B8-26, CHN-N3-2, CHN-N4-18, CHN-N8-5, CHN-N10-18, CHN-N1-56, CHN-N2-5, CHN-L7-40, and CHN-Q5-1, respectively. The differential intracellular protein spots marked with red numbers were characterized by the LC-MS/MS analysis. pI, isoelectric point.
Identification of Differential Intracellular Proteins of the V. parahaemolyticus Isolates
Differential intracellular proteins of the 12 V. parahaemolyticus isolates were identified by LC-MS/MS analysis and summarized in Table 4. A total of 97 protein sequences were obtained and classified into three major GO categories in the GO database, including the biological process, cellular component, and molecular function (Supplementary Figure S2). Given that multiple biological functions could be assigned for single identified protein, the most abundant GO term was the catalytic activity (72.2%, 70/97), followed by the binding (58.8%, 57/97), cellular process (46.4%, 45/97), and metabolic process (46.4%, 45/97). The opposite patterns were cellular component organization or biogenesis (2.1%, 2/97), signaling (2.1%, 2/97), molecular function regulator (2.1%, 2/97), electron carrier activity (2.1%, 2/97), antioxidant activity (1.0%, 1/97), and multiorganism process (1.0%, 1/97) (Supplementary Figure S2A).

Table 4. Identification of the differential protein spots on the proteomic profiles of the 12 V. parahaemolyticus isolates by LC-MS/MS analysis.
Proteomic Comparison of the V. parahaemolyticus Isolates From Three Kinds of Aquatic Products
The proteomic profiles of the shellfish, crustaceans, and fish origins were also different (Figure 3). Interestingly, approximately 28.9% (28/97) of the differential Intracellular proteins only appeared on the proteomic profiles of the V. parahaemolyticus isolates from the eight species of shellfish, but absent from the crustaceans and fish. Of these proteins, the glycerol dehydrogenase (GldA, Spot P12), 5′-nucleotidase surE (Spot P21), and putative glucose-6-phosphate 1-epimerase (G6PE, Spot P30) were shown only on the proteomic profile of the CHN-B2-28 isolate from R. philippinarum. The GldA (Spot P12) is required to catalyze the first step in fermentative glycerol metabolism, and its product is then funneled into the glycolytic pathway for further degradation. The 5′-nucleotidase surE (Spot P21) has nucleotidase activity and is involved in nucleotide metabolism in Escherichia coli (Proudfoot et al., 2004). The G6PE (Spot P30) catalyzes glucose-6-phosphate to fructose-6-phosphate that converted to fructose-1,6-bisphosphate under the catalysis of PFK (Spot P16). In this study, seven intracellular proteins were found only on the proteomic profile of the CHN-B5-29 isolate from P. magellanicus, including the periplasmic serine endoprotease DegP-like (Spot P33), GAPDH (Spot P40), oxidoreductase (Spot P42), threonine aldolase (Spot P43), azurin (Spot P47), s-ribosylhomocysteine lyase (LuxS, Spot P51), and tRNA-binding protein (Spot P52). Among these proteins, for instance, the oxidoreductase (Spot P42) facilitates the detoxification of xenobiotic organic compounds by various microorganisms (Khatoon et al., 2017). The threonine aldolase (Spot P43) catalyzes the cleavage of threonine into glycine and acetaldehyde and involves in threonine decomposition and glycine synthesis (Liu et al., 2015). Azurin (Spot P47) is necessary for bacterial protection from oxidative stress (electron donor to nitrate reductase) and copper toxicity in P. aeruginosa (Mohammadi-Barzelighi et al., 2019). The LuxS (Spot P51) is highly conserved among Aeromonas hydrophila, E. coli, V. cholerae, and Vibrio harveyi and has a critical role in regulation of genes associated with the transport of nucleotides, metabolism, and synthesis of cell walls or membranes. In this study, the cysteine desulfurase IscS (Spot P57) was observed only on the proteomic profile of the CHN-B6-62 isolate from S. constricta, which was involved in iron–sulfur cluster biogenesis and oxidative stress defense in Mycobacterium tuberculosis (Rybniker et al., 2014). Additionally, the CHN-N4-18 isolate from P. viridis expressed the outer membrane β-barrel domain protein (Spot P69), OmpW (Spot P70), and putative 4-hydroxy-4-methyl-2-oxoglutarate aldolase (Spot P71) (Table 4).
For the V. parahaemolyticus isolates of the crustaceans origin, six differential intracellular proteins appeared only on the proteomic profile of the CHN-N1-56 isolate from L. vannamei, including the ParB family protein (Spot P75), porin (Spot P77), outer membrane protein A (OmpA)–like domain-containing protein (Spot P78), uracil phosphoribosyltransferase (UPRT, Spot P81), ribosome-recycling factor (Spot P82), and an uncharacterized protein (Spot P84) encoded by the VPA1627 gene of V. parahaemolyticus RIMD 2210633 strain (Table 4). Recent research has indicated that the UPRT (Spot P81) converts uracil to uridine monophosphate in the pyrimidine salvage pathway in the presence of phosphoribosyl pyrophosphate (Silva et al., 2019). Additionally, the carbamoyl-phosphate synthase small chain (Spot 79) was only expressed by the CHN-N1-56 and CHN-N2-5 isolates from L. vannamei and O. oratoria, respectively (Table 4).
For the V. parahaemolyticus isolates of the fish origin, six differential intracellular proteins were expressed only by the CHN-Q5-1 isolate from C. idellus, including the OmpK (Spot P80), alcohol dehydrogenase (Spot P88), pyruvate dehydrogenase E1 component subunit alpha (Spot P89), inorganic diphosphatase (Spot P90), acetyltransferase (Spot P91), and single-stranded DNA-binding protein (SSB, Spot P92). The acetyltransferase (Spot P91) acts on acetylation of amino acids, which determines vital regulatory processes (Christensen et al., 2019). In addition, three intracellular proteins were only expressed by the CHN-L7-40 isolate from A. nobilis, including the tRNA-specific 2-thiouridylase MnmA (Spot P93), S-(hydroxymethyl) glutathione dehydrogenase (Spot P94), 2,3,4,5-tetrahydropyridine-2,6-dicarboxylate N-succinyltransferase (Spot P96), and transaldolase (Spot P97) (Table 4).
Proteomic Comparison of the V. parahaemolyticus Isolates With Pathogenic Reference Strains
The proteomic profiles of the V. parahaemolyticus ATCC33847 and ATCC17802 strains were also obtained (Supplementary Figure S3). These toxic strains of clinical origin appeared to express more intracellular proteins (459–462) than the 12 V. parahaemolyticus isolates of aquatic product origins (312–343). Comparative proteomic analysis revealed that approximately 23.7% (23/97) of the differential intracellular proteins expressed by the 12 V. parahaemolyticus isolates (Table 4) were observed at similar locations on the proteomic profiles of the ATCC33847 and the ATCC17802 strains (Supplementary Figure S3), including three membrane-related proteins: OmpA family protein (Spot P15), cytochrome c oxidase (Spot P46), and lipoprotein (Spot P48); 10 metabolism-related proteins: aldehyde-alcohol dehydrogenase (AdhE) (Spot P1), PFK (Spot P16), succinylglutamate desuccinylase (Spot P17), FMN reductase (Spot P22), autonomous glycyl radical cofactor (Spot P25), triosephosphate isomerase (Spot P28), glucose-6-phosphate isomerase (Spot P31), glycerol-3-phosphate dehydrogenase (Spot P32), GAPDH (Spot P40), and cytidylate kinase (Spot P50); three biosynthesis-related proteins: ASD (Spot P7), endoribonuclease L-PSP (Spot P24), and tRNA-binding protein (Spot P52); two translation-associated proteins: EF-Tu (Spot P11) and an EF-Ts (Spot P20); one transport protein [phosphoenolpyruvate-protein phosphotransferase (PtsA), Spot P36]; and four bacterial cell protection factors: antioxidant, alkyl hydroperoxide reductase C (AhpC)/Tsa family (AhpC) (Spot P23), periplasmic serine endoprotease DegP-like (Spot P33), cytidylate kinase (Spot P50), and ATP-dependent metallopeptidase HflB (Spot P54) (Table 4).
Notably, among the differential intracellular proteins identified in this study, some were reported to be involved in the virulence of pathogenic bacteria, including the AdhE (Spot P1), OmpAs (Spots P15 and P78), AhpC (Spot P23), PtsA (Spot P36), and phosphoglycerate kinase (PGK) (Spot P95). The latter catalyzes the transfer of a phosphate group from 1,3-diphosphoglycerate to ADP to produce 3-phosphoglycerate and ATP (Smith et al., 2011).
Effects of Aquatic Product Matrix on Proteomic Profiles of the V. parahaemolyticus Isolates
To get insights into implications of aquatic product matrices on the proteomes of the 12 V. parahaemolyticus isolates, we incubated these isolates in their corresponding matrix media and identified a total of 71 differential intracellular proteins by the 2D-GE and LC-MS/MS analysis (Figures 4–6 and Table 5). These proteins were classified into three major GO categories, in which the most abundant GO term was catalytic activity (62.0%, 44/71), followed by cell (50.7%, 36/71) and binding (49.3%, 35/71). The opposite patterns were biological regulation (1.4%, 1/71), organelle (1.4%, 1/71), and structural molecule activity (1.4%, 1/71) (Supplementary Figure S2B).

Table 5. Identification of the differential proteins of the 12 V. parahaemolyticus isolates incubated between in the TSB and aquatic product matrix media.
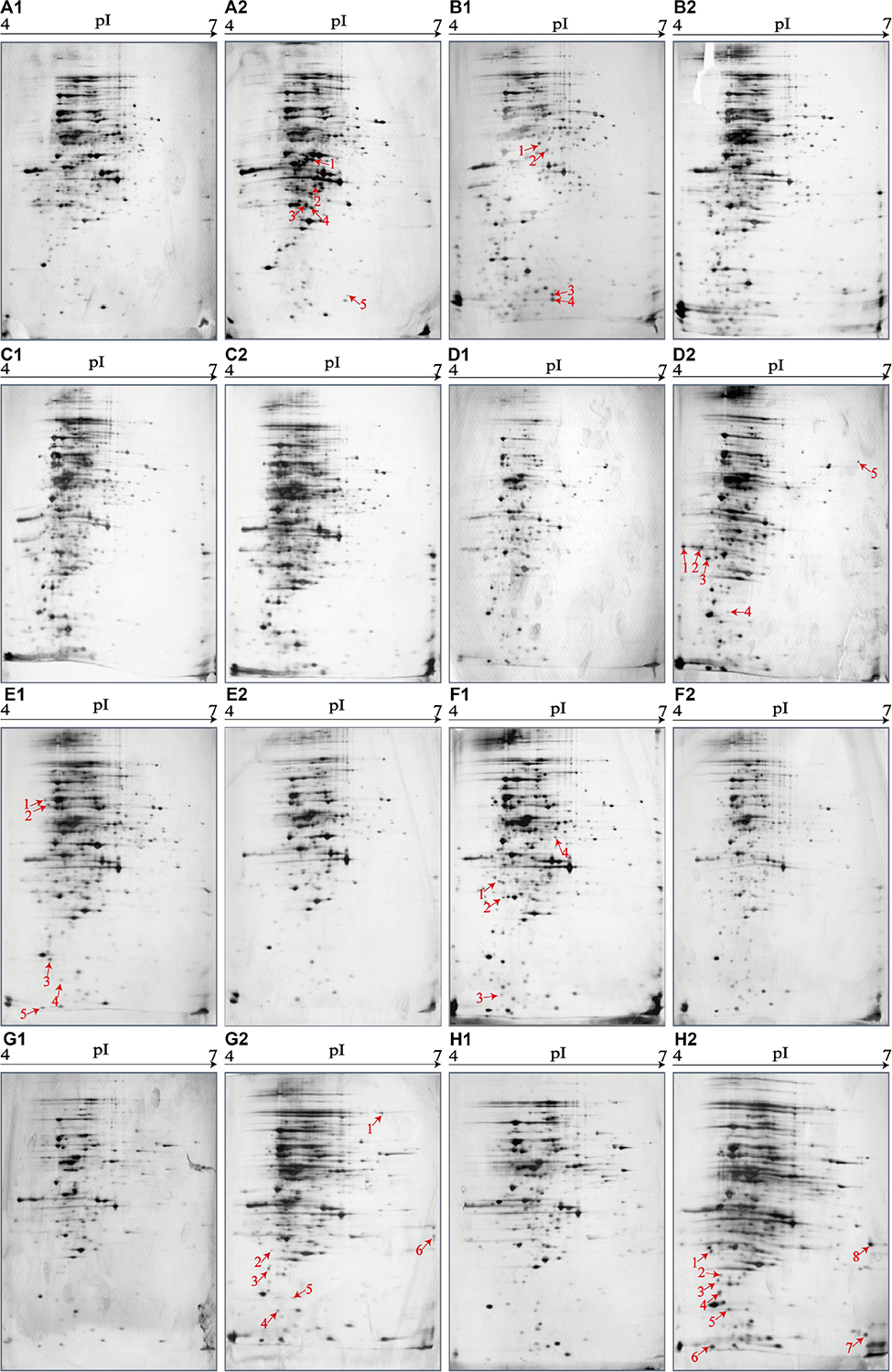
Figure 4. Effects of aquatic product matrices on proteomic profiles of the V. parahaemolyticus isolates of the shellfish origin. (A1–H1) V. parahaemolyticus CHN-2-28, CHN-B5-29, CHN-B6-62, CHN-B8-26, CHN-N3-2, CHN-N4-18, CHN-N8-5, and CHN-N10-18 isolates incubated in the TSB medium (pH 8.5, 3% NaCl) at 37°C, respectively. (A2–H2) The V. parahaemolyticus isolates were incubated in the R. philippinarum, P. magellanicus, S. constricta, S. strictus, P. undulate, P. viridis, M. veneriformis, and O. gigas thunberg matrices media at 37°C, respectively.
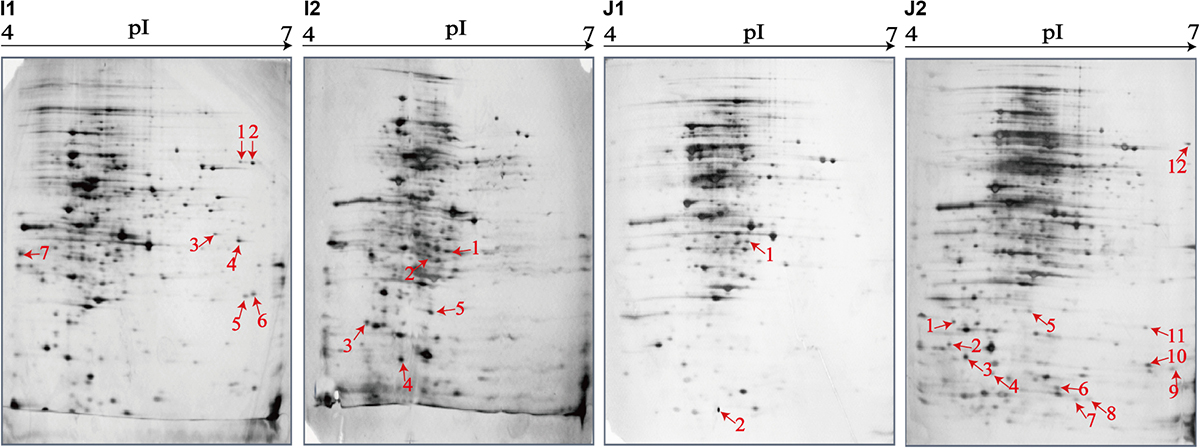
Figure 5. Effects of aquatic product matrix on proteomic profiles of the V. parahaemolyticus isolates of the crustaceans origin. (I1,J1) V. parahaemolyticus CHN-N1-56 and N2-5 isolates incubated in the TSB medium (pH 8.5, 3% NaCl) at 37°C, respectively. (I2,J2) the CHN-N1-56 and N2-5 isolates incubated in the L. vannamei and O. oratoria matrices media at 37°C, respectively.
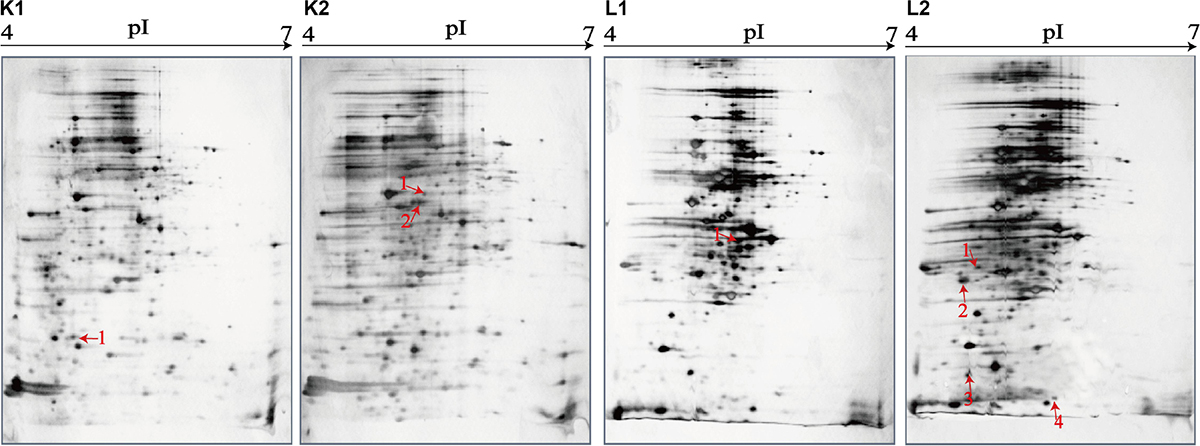
Figure 6. Effects of aquatic product matrix on proteomic profiles of the V. parahaemolyticus isolates of the fish origin. (K1,L1) V. parahaemolyticus CHN-L7-40 and Q5-1 isolates incubated in the TSB medium (pH 8.5, 3% NaCl) at 37°C, respectively. (K2,L2) the CHN-L7-40 and Q5-1 isolates incubated in the A. nobilis and C. idellus matrices media at 37°C, respectively.
The shellfish matrix media obviously changed the proteomic profiles of the 8 V. parahaemolyticus isolates of the shellfish origin (Figures 4A2–H2). For instance, approximately 319 and 326 intracellular protein spots were observed on the proteomic profiles of the CHN-N10-28 isolate grown in the TSB and O. gigas thunberg matrix media, respectively (Figures 4H1,H2). Among these, eight intracellular proteins were expressed by the CHN-N10-28 isolate grown in the O. gigas thunberg matrix medium (Figure 4H2), including the OmpA family protein (Spot H2-1), putative glucose-6-phosphate 1-epimerase (Spot H2-2), ribokinase (Spot H2-3), peptidyl-prolyl cis-trans isomerase (Spot H2-4), triosephosphate isomerase (Spot H2-5), autonomous glycyl radical cofactor (Spot H2-6), GAPDH (Spot H2-8), and an uncharacterized protein (Spot H2-7) encoded by the VPA1627 gene of V. parahaemolyticus RIMD 2210633 strain with currently unknown functions in public databases (Table 5).
For the crustacean matrix media, for example, approximately 314 and 325 intracellular proteins were produced by the CHN-N2-5 isolate grown in the TSB and O. oratoria matrix media, respectively (Figures 5J1,J2). Two differential intracellular proteins were expressed by the CHN-N2-5 isolate grown in the former medium (Figure 5J1), including the glycine betaine-binding protein OpuAC (Spot J1-1), and DNA polymerase sliding clamp subunit (Spot J1-2). Remarkably, approximately 12 differential intracellular proteins were expressed by the CHN-N2-5 isolate grown in the O. oratoria matrix medium (Figure 5J2), including the deoxyribose-phosphate aldolase (Spot J2-1), phosphoribosyltransferase (Spot J2-2), outer membrane β-barrel domain protein (Spot J2-3), GrpE (Spot J2-4), lipoprotein (Spot J2-5), azurin (Spot J2-6), 6,7-dimethyl-8-ribityllumazine synthase (Spot J2-7), histidine triad nucleotide-binding protein (Spot J2-8), 3-hydroxydecanoyl-(acyl-carrier-protein) dehydratase (Spot J2-10), succinate dehydrogenase flavoprotein subunit (Spot J2-11), glycerol-3-phosphate dehydrogenase (Spot J2-12), and an uncharacterized protein (Spot J2-9) encoded by the VPA1627 gene of V. parahaemolyticus RIMD 2210633 strain (Table 5).
Likewise, for the fish matrix media, for instance, approximately 329 and 333 intracellular protein spots were observed on the proteomic profiles derived from the CHN-Q5-1 isolate grown in the TSB and C. idellus matrix media, respectively (Figures 6L1,L2). The aspartate carbamoyltransferase (Spot L1-1) was expressed by the CHN-Q5-1 isolate grown in the former medium (Figure 6L1), whereas four were produced in the C. idellus matrix medium (Figure 6L2), including the 3-chlorobenzoate-3,4-dioxygenase dehydrogenase (Spot L2-1), OmpA family protein (Spot L2-2), GrpE (Spot L2-3), and 50S ribosomal protein L9 (Spot L2-4) (Table 5). Additionally, to validate the differential proteins induced by the aquatic product matrices, we examined gene expression of several representative proteins by the quantitative RT-PCR assay. The resulting data were generally consistent with the proteomic analysis (Supplementary Figure S4).
Aquatic Product Matrix Composition
As shown in Supplementary Figure S5, the protein (3.73‰–0.43‰), carbohydrate (1.23‰–0.01‰), and crude fat (1.00‰–0.01‰) contents of the 12 types of aquatic product matrices were remarkably different. The protein concentration in the P. undulate matrix was the highest (3.73‰), followed by 3.44‰ in the P. viridis, and 3.25‰ in the L. vannamei matrices, whereas the opposite patterns were observed in the P. magellanicus (1.50‰), S. strictus (1.36‰), and O. gigas thunberg (0.43‰) matrices. The fat contents of the aquatic product matrices were much higher in the L. vannamei (1.01‰), O. oratoria (0.85‰), and P. magellanicus (0.84%) than those in the P. undulate (0.36%), S. strictus (0.31%), and S. constricta (0.01%) matrices. The carbohydrate content of the P. viridis (1.23‰) matrix was the highest, whereas that of the O. gigas thunberg (0.01‰) matrix was the lowest (Supplementary Figure S5).
Discussion
Vibrio parahaemolyticus is the leading seafoodborne pathogenic bacterium worldwide. Nevertheless, the information in V. parahaemolyticus proteomics is minimally available to date (Fu et al., 2014; He et al., 2015; Perez-Acosta et al., 2018; Tang et al., 2018; Zhong et al., 2019). Based on our prior studies, in this study, we obtained and compared the secretomic and proteomic profiles of the V. parahaemolyticus isolates recovered from 12 species of commonly consumed aquatic products by 2D-GE and LC-MS/MS analysis.
Secreted proteins have a major role in the pathogenesis of bacterial infection of host cells. He et al. (2015) investigated secretomic profiles derived from V. parahaemolyticus strains isolated from five species of aquatic products and identified six extracellular virulence-associated proteins involved in the pathogenicity of bacteria, such as EF-Tu, pyridoxine 5′-phosphate synthase, σ54 modulation protein, dihydrolipoyl dehydrogenase, transaldolase, and PGK. Among these proteins, the EF-Tu (Spot c) and transaldolase (Spot S5) were also identified by the comparative secretomic analysis of the 12 V. parahaemolyticus isolates in this study. The EF-Tu (Spot c) secreted by all the V. parahaemolyticus isolates was reported to be exposed on the cell surface of Streptococcus, Neisseria, and Mycoplasma and under certain conditions on E. coli (Mikhalchik et al., 2019). The transaldolase (Spot S5) was secreted by the V. parahaemolyticus CHN-B2-28, CHN-B6-62, CHN-B8-26, CHN-N4-18, CHN-N10-18, CHN-L7-40, and CHN-Q5-1 isolates. Gonzalez-Rodriguez et al. reported that the transaldolase (Spot S5) recruited on the cell surface via a non-classical secretion mechanism or an uncharacterized translocation pathway and acted as an important colonization factor for the survival of Bifidobacterium bifidum in host intestinal tract (Gonzalez-Rodriguez et al., 2012). In this study, some other extracellular proteins involved in bacterial virulence were also identified. For instance, the superoxide dismutase (Spot S26) secreted by the V. parahaemolyticus CHN-B5-29 and CHN-B6-62 isolates is an important virulence factor of Vibrio alginolyticus and contributes to the antioxidative stress with potential application for live attenuated vaccine (Chen et al., 2019).
The initial contact and anchoring of bacteria to a host cell are essential during the process of infection (Li et al., 2019). Adhesions are present at the bacterial cell surface or released into extracellular space to form a contact platform for bacterial attachment onto a host cell (Zhang and Orth, 2013). In this study, our secretomic data derived from the 12 V. parahaemolyticus isolates revealed such proteins involved in the adhesions of pathogenic bacteria. For example, the TolC (Spot a), secreted by all the V. parahaemolyticus isolates, is a major adhesin in V. harveyi (Zhu et al., 2019). The enolase (Spot S2), secreted by the V. parahaemolyticus CHN-B2-28, CHN-B5-29, CHN-B6-62, CHN-N1-56, CHN-N3-2, CHN-N4-18, CHN-N8-5, and CHN-Q5-1 isolates, is an adhesion-related factor that binds plasminogen and allows bacteria to acquire surface-associated proteolytic activity that facilitates invasion and dissemination in the infected host (Jiang et al., 2014). Bacterial membrane proteins can act as adhesion factors or adhesion enhancers (Gordon et al., 2015). For example, the GAPDH (Spot S13), like many housekeeping proteins, has been presumed to exist only in the cytoplasm to involve in glycolysis. However, it has been reported that GAPDH can be recruited on the cell surface and secreted via a non-classical secretion mechanism, and therefore it is a suitable vaccine candidate for protection against bacterial and parasitic diseases (Perez-Casal and Potter, 2016). The nitrogen regulatory protein P-II (Spot S28) is one of the most widely distributed families of signal transduction proteins widespread among bacteria, archaea, and plants (Radchenko and Merrick, 2011) and control the activities of a very diverse range of enzymes, transcription factors and some membrane transport proteins by direct interaction with their target hosts (Merrick, 2014). The maltoporin (Spot b), secreted by all the V. parahaemolyticus isolates, belongs to the outer membrane porin family of Gram-negative bacteria (Thoma et al., 2017) and is a versatile vaccine candidate in Vibrio species (Lun et al., 2014). Recently, Yang B. et al. (2019) reported that the maltoporin (Spot b) also contributed to the adhesion and invasion ability of Aeromonas veronii TH0426 to epithelioma papulosum cyprini cells. Additionally, bacterial flagellins, potent immunomodulatory agents, contribute to bacterial adhesion and invasion of host cells as well (Hajam et al., 2017). In this study, the flagellin C (Spot S7) was secreted by the V. parahaemolyticus CHN-B2-28, CHN-B8-26, CHN-N4-18, CHN-L7-40, and CHN-Q5-1 isolates, whereas the polar flagellin B/D (Spot S8) was secreted by the CHN- B2-28 and CHN-B8-26 isolates. These identified extracellular proteins could be the main targets of vaccine development because of their exposed epitopes on the cell surface.
In this study, comparative proteomic analysis also revealed several intracellular proteins related to bacterial virulence, including the AdhE (Spot P1), OmpAs (Spot P15 and Spot P78), AhpC (Spot P23), PtsA (Spot P36), and PGK (Spot P95). For instance, the AdhE (Spot P1) was expressed by the majority of V. parahaemolyticus strains (except the CHN-B8-26, N2-5, N8-5, and L7-40 isolates). Luong et al. (2015) reported that ΔadhE mutant strain decreased pneumolysin (Ply) under ethanol stress condition when compared to wild-type strain and implied that AdhE was a pneumococcal virulence factor in Streptococcus pneumoniae. The OmpAs (Spot P15 and Spot P78), expressed by the V. parahaemolyticus CHN-B2-28, CHN-B5-29, CHN-N1-56, CHN-N4-18, and CHN-Q5-1 isolates, belong to a group of surface-exposed porins associated with bacterial pathogenesis in V. parahaemolyticus (Cheng et al., 2018). In this study, the AhpC (Spot P23) was expressed by the CHN-B2-28, CHN-N1-56, CHN-N3-2, CHN-N4-18, CHN-N10-23, and CHN-Q5-1 isolates. It has been reported that AhpC in highly virulent Francisella tularensis serves as a key antioxidant enzyme and contributes to its robust oxidative and nitrosative stress resistance and intramacrophage survival and consequently serves as a virulence factor (Alharbi et al., 2019). The PtsA (Spot P36), expressed by the CHN-B5-29, CHN-B6-62, CHN-N2-5, CHN-N3-2, CHN-N4-18, and CHN-L7-40 isolates, is an intracellular protein of the monosaccharide phosphotransferase systems and also localizes to the bacterial cell wall as an adhesin in S. pneumoniae (Mizrachi Nebenzahl et al., 2016). The PGK (Spot P95), expressed by the CHN-Q5-1 isolate from C. idellus, is a key enzyme of glycolysis and also acts as a mediator of DNA replication and repair in the nucleus (Kumar et al., 2019). This protein, also identified in our prior research (He et al., 2015), has been used as an antigen in a neonatal-animal model against Streptococcus agalactiae infection (Wang et al., 2014).
The increase in MDR pathogenic bacteria has raised a serious public health and economic concern (Elmahdi et al., 2016). One common mechanism for bacteria to obtain antibiotic resistance is to actively pump drugs from bacterial cells by employing ABC transporters (Wilson, 2016). The functions of ABC transporters are very diverse, ranging from importing essential nutrients to conferring drugs in bacteria, archaea, and eukaryote (Beis, 2015). In this study, comparative secretomic analysis revealed several ABC transporters of the V. parahaemolyticus isolates with resistance phenotypes. For instance, the maltodextrin-binding protein (Spot d), secreted by all the V. parahaemolyticus isolates, is part of the maltose ABC complex MalEFGK (Machtel et al., 2019). The D-ribose ABC TSBP (Spot S15) and arginine ABC TSBP (Spot S18) were secreted by most of the isolates, except the CHN-B6-62 and CHN-Q5-1, as well as CHN-B6-62 and CHN-N1-56 isolates, respectively. The choline ABC TSBP (Spot S14) was secreted by the CHN-B2-28, CHN-B5-29, CHN-N1-56, CHN-N2-5, and CHN-N8-5 isolates. The glycine/betaine ABC TSBP (Spot S12) was observed only on the secretomic profile derived from the CHN-B2-28 and CHN-B8-26 isolates, whereas the peptide ABC TPPBP (Spot S24) was observed from the CHN-B2-28 and CHN-N2-5 isolates. The other possible mechanism of bacterial resistance is the ribosome protection (Wilson, 2016). Bacterial ribosome, being one of the main antibiotic targets in bacterial cells (Wilson, 2014), is a large protein–RNA complex that consists of two major subunits (a small 30S subunit and a large 50S subunit), each of which is composed of a variety of proteins. In this study, two translation-associated proteins EF-Tu (Spot c) and EF-Ts (Spot S22) were identified by the secretomic and proteomic analysis. The former existed in all the V. parahaemolyticus isolates, whereas the latter was secreted by the CHN-B2-28, CHN-B8-26, CHN-N3-2, CHN-N4-18, CHN-N10-18, CHN-L7-40, and CHN-Q5-1 isolates. The EF-Ts is a guanosine nucleotide exchange factor for EF-Tu (He et al., 2015), and the EF-Tu catalyzes the binding of aminoacyl-tRNA (aa-tRNA) to a site of the ribosome during protein synthesis (Daviter et al., 2003). Agarwal and O’Connor (2014) reported that a ribosomal protein S12 binding the EF-Tu contributed to streptomycin resistance in E. coli MC323. Overall, these identified proteins may serve as an explanation for the resistance phenotypes of the V. parahaemolyticus isolates with aquatic product origins.
In this study, all the V. parahaemolyticus isolates (except the CHN-B6-62 isolate) produced more intracellular protein spots on their proteomic profiles responding to aquatic product matrices. A total of 71 differential intracellular proteins were identified by the LC-MS/MS analysis, most of which were involved in biosynthesis process (e.g., diaminopimelate, lysine, ADP-L-glycero-β-D-manno-heptose, S-adenosylmethionine, GMP, riboflavin, and fatty acid biosynthesis), metabolic processes (e.g., gluconeogenesis, glycerol, one-carbon, glycerol-3-phosphate, alcohol, carbohydrate, D-ribose, deoxyribonucleotide, and amino acid metabolism), and cell membrane composition. The growth of microorganism may vary with the available carbon and nitrogen sources (Chiang and Chou, 2008). In this study, our comparative proteomic analysis showed that the crustacean matrices changed the proteomes of the V. parahaemolyticus CHN-N1-56 and CHN-N2-5 isolates, recovered from L. vannamei and O. oratoria, respectively, more than the other 10 types of aquatic product matrices. Wang et al. (2018) evaluated the influence of food matrices (shrimp, oyster, freshwater fish, pork, chicken, and egg fried rice) on extracellular products of V. parahaemolyticus and found that V. parahaemolyticus expressed significantly higher activity (p < 0.05) of gelatinase, caseinase, urease, DNase, and amylase in shrimp matrix than freshwater fish. In this study, our data also showed that the crude fat contents of the crustacean matrices were approximately 101- to 85-fold higher than those of the fish and shellfish matrices. Lipases expressed by Vibrio species can hydrolyze fats into glycerol and fatty acids (Beshiru and Igbinosa, 2018). Moravec et al. (2017) reported the Vibrio’s ability to acquire fatty acids from environmental sources. Exogenous fatty acids can affect bacterial metabolism, modification of membrane lipids, alteration of protein function, regulation of gene expression, and stress responses (Moravec et al., 2017; Yao and Rock, 2017). In this study, our comparative proteomic data highlighted the significance of monitoring V. parahaemolyticus contamination in fat-rich aquatic products in the future research.
In this study, the other interesting finding was that some V. parahaemolyticus isolates produced virulence-associated proteins when incubated only in aquatic product matrices media. For instance, the OmpA family proteins (Spots D2-1, D2-2, D2-3, H2-1, and L2-2) were expressed by the V. parahaemolyticus CHN-B8-26 and CHN-N10-18 isolates when grown in the S. strictus and O. gigas thunberg matrices media, respectively. Recent research has indicated that proteins and lipids can form complexes (called liprotides) that assist in folding of outer membrane proteins, for example, OmpA, especially optimal folding at pH 8–9 (Nedergaard Pedersen et al., 2018). Protein folding is the essential process by which a polypeptide chain acquires its functional, native 3D structure. In this study, our data suggested that the aquatic product matrices with higher contents of protein and fat may facilitate the expression of virulence-associated factors (e.g., the OmpA family proteins) in the V. parahaemolyticus isolates.
Although gel-based proteomes are laboring and time-consuming, images of 2D-GE gels can be compared so as to quantify each protein spot from different samples, and these protein spots can subsequently be excised, sequenced, and identified with MS, especially LC-MS/MS (Lee et al., 2019). Each protein has multiple forms as a result of genetic variations, splicing, truncation, degradation, and posttranslational modifications; therefore, innovated proteomic technologies such as MS-based shotgun proteomics (Lee et al., 2018) should be employed to explore more virulence and resistance-associated factors in V. parahaemolyticus. Also, new proteins during culture handing/extraction processing should be prevented by adding a compound (e.g., chloramphenicol), and the function of the virulence and resistance-related proteins identified in the V. parahaemolyticus isolates should be further pursued by cell and animal infection mode analysis in the future research.
Data Availability Statement
The datasets generated for this study are available on request to the corresponding author.
Author Contributions
ZZ, LY, PY, YW, XP, and LC participated in the design and discussion of the study. ZZ carried out the experiments. LY performed the qRT-PCR assay. ZZ and LC wrote the manuscript. All the authors read and approved the final version to be published.
Funding
This study was supported by the grants from the Shanghai Municipal Science and Technology Commission (No. 17050502200) and the National Natural Science Foundation of China (No. 31671946).
Conflict of Interest
The authors declare that the research was conducted in the absence of any commercial or financial relationships that could be construed as a potential conflict of interest.
Supplementary Material
The Supplementary Material for this article can be found online at: https://www.frontiersin.org/articles/10.3389/fmicb.2020.01453/full#supplementary-material
FIGURE S1 | The secretomic profiles of the V. parahaemolyticus ATCC33847 and ATCC17802 strains by the 2D-GE analysis. (A) ATCC33847. (B) ATCC17802. The protein spots marked with blue circles and the numbers in red were the same at similar locations on the secretomic profiles of the 12 V. parahaemolyticus isolates. pI, isoelectric point.
FIGURE S2 | The GO functional classification of the differential intracellular proteins. (A) The differential intracellular proteins of 12 V. parahaemolyticus isolates. (B) The differential intracellular proteins of the 12 V. parahaemolyticus isolates incubated between in the TSB and aquatic product matrix media.
FIGURE S3 | The proteomic profiles of the V. parahaemolyticus ATCC33847 and ATCC17802 strains. (A) ATCC33847. (B) ATCC17802. The intracellular protein spots marked with the numbers in red were the same at similar locations on the proteomic profiles of the 12 V. parahaemolyticus isolates.
FIGURE S4 | The expression of several representative genes encoding differential proteins by the RT-PCR assay.
FIGURE S5 | The protein, carbohydrate and fat contents of the 12 types of aquatic product matrices.
TABLE S1 | Oligonucleotide primers used for the RT-PCR assay in this study.
Footnotes
References
Agarwal, D., and O’Connor, M. (2014). Diverse effects of residues 74-78 in ribosomal protein S12 on decoding and antibiotic sensitivity. Biochem. Biophys. Res. Commun. 445, 475–479. doi: 10.1016/j.bbrc.2014.02.031
Albalasmeh, A. A., Berhe, A. A., and Ghezzehei, T. A. (2013). A new method for rapid determination of carbohydrate and total carbon concentrations using UV spectrophotometry. Carbohydr. Polym. 97, 253–261. doi: 10.1016/j.carbpol.2013.04.072
Alharbi, A., Rabadi, S. M., Alqahtani, M., Marghani, D., Worden, M., Ma, Z., et al. (2019). Role of peroxiredoxin of the AhpC/TSA family in antioxidant defense mechanisms of Francisella tularensis. PLoS One 14:e0213699. doi: 10.1371/journal.pone.0213699
Aslam, B., Basit, M., Nisar, M. A., Khurshid, M., and Rasool, M. H. (2017). Proteomics: technologies and their applications. J. Chromatogr. Sci. 55, 182–196. doi: 10.1093/chromsci/bmw167
Baker-Austin, C., Oliver, J. D., Alam, M., Ali, A., Waldor, M. K., Qadri, F., et al. (2018). Vibrio spp. infections. Nat. Rev. Dis. Primers. 4:8. doi: 10.1038/s41572-018-0005-8
Baumann, P., Baumann, L., and Reichelt, J. L. (1973). Taxonomy of marine bacteria: Beneckea parahaemolytica and Beneckea alginolytica. J. Bacteriol. 113, 1144–1155. doi: 10.1128/jb.113.3.1144-1155.1973
Beis, K. (2015). Structural basis for the mechanism of ABC transporters. Biochem. Soc. Trans. 43, 889–893. doi: 10.1042/bst20150047
Ben-Yaakov, R., and Salomon, D. (2019). The regulatory network of Vibrio parahaemolyticus type VI secretion system 1. Environ. Microbiol. 21, 2248–2260. doi: 10.1111/1462-2920.14594
Beshiru, A., and Igbinosa, E. O. (2018). Characterization of extracellular virulence properties and biofilm-formation capacity of Vibrio species recovered from ready-to-eat (RTE) shrimps. Microb. Pathog. 119, 93–102. doi: 10.1016/j.micpath.2018.04.015
Castillo, D., Perez-Reytor, D., Plaza, N., Ramirez-Araya, S., Blondel, C. J., Corsini, G., et al. (2018). Exploring the genomic traits of non-toxigenic Vibrio parahaemolyticus strains isolated in southern chile. Front. Microbiol. 9:161. doi: 10.3389/fmicb.2018.00161
Chaitiemwong, N., Hazeleger, W. C., and Beumer, R. R. (2010). Survival of Listeria monocytogenes on a conveyor belt material with or without antimicrobial additives. Int. J. Food Microbiol. 142, 260–263. doi: 10.1016/j.ijfoodmicro.2010.06.021
Chen, L., and Alali, W. (2018). Editorial: recent discoveries in human serious foodborne pathogenic bacteria: resurgence, pathogenesis, and control strategies. Front. Microbiol. 9:2412. doi: 10.3389/fmicb.2018.02412
Chen, Y., Wu, F., Pang, H., Tang, J., Cai, S., and Jian, J. (2019). Superoxide dismutase B (sodB), an important virulence factor of Vibrio alginolyticus, contributes to antioxidative stress and its potential application for live attenuated vaccine. Fish. Shellf. Immunol. 89, 354–360. doi: 10.1016/j.fsi.2019.03.061
Cheng, Z. X., Chu, X., Wang, S. N., Peng, X. X., and Li, H. (2018). Six genes of ompA family shuffling for development of polyvalent vaccines against Vibrio alginolyticus and Edwardsiella tarda. Fish. Shellf. Immunol. 75, 308–315. doi: 10.1016/j.fsi.2018.02.022
Chiang, M. L., and Chou, C. C. (2008). Expression of superoxide dismutase, catalase and thermostable direct hemolysin by, and growth in the presence of various nitrogen and carbon sources of heat-shocked and ethanol-shocked Vibrio parahaemolyticus. Int. J. Food Microbiol. 121, 268–274. doi: 10.1016/j.ijfoodmicro.2007.11.001
Christensen, D. G., Xie, X., Basisty, N., Byrnes, J., McSweeney, S., Schilling, B., et al. (2019). Post-translational protein acetylation: an elegant mechanism for bacteria to dynamically regulate metabolic functions. Front. Microbiol. 10:1604. doi: 10.3389/fmicb.2019.01604
Daviter, T., Wieden, H. J., and Rodnina, M. V. (2003). Essential role of histidine 84 in elongation factor Tu for the chemical step of GTP hydrolysis on the ribosome. J. Mol. Biol. 332, 689–699. doi: 10.1016/s0022-2836(03)00947-1
De Nisco, N. J., Kanchwala, M., Li, P., Fernandez, J., Xing, C., and Orth, K. (2017). The cytotoxic type 3 secretion system 1 of Vibrio rewires host gene expression to subvert cell death and activate cell survival pathways. Sci. Signal. 10:aal4501. doi: 10.1126/scisignal.aal4501
De Souza Santos, M., Salomon, D., and Orth, K. (2017). T3SS effector VopL inhibits the host ROS response, promoting the intracellular survival of Vibrio parahaemolyticus. PLoS Pathog. 13:e1006438. doi: 10.1371/journal.ppat.1006438
Elmahdi, S., DaSilva, L. V., and Parveen, S. (2016). Antibiotic resistance of Vibrio parahaemolyticus and Vibrio vulnificus in various countries: a review. Food Microbiol. 57, 128–134. doi: 10.1016/j.fm.2016.02.008
Fu, L. L., Wang, R., Wang, Y., and Lin, J. (2014). Proteomic identification of responsive proteins of Vibrio parahaemolyticus under high hydrostatic pressure. J. Sci. Food Agric. 94, 2630–2638. doi: 10.1002/jsfa.6595
Fujino, T., Miwatani, T., Yasuda, J., Kondo, M., Takeda, Y., Akita, Y., et al. (1965). Taxonomic studies on the bacterial strains isolated from cases of “shirasu” food-poisoning (Pasteurella parahaemolytica) and related microorganisms. Biken. J. 8, 63–71.
Ghenem, L., Elhadi, N., Alzahrani, F., and Nishibuchi, M. (2017). Vibrio parahaemolyticus: a review on distribution, pathogenesis, virulence determinants and epidemiology. Saudi. J. Med. Med. Sci. 5, 93–103. doi: 10.4103/sjmms.sjmms_30_17
Gonzalez-Rodriguez, I., Sanchez, B., Ruiz, L., Turroni, F., Ventura, M., Ruas-Madiedo, P., et al. (2012). Role of extracellular transaldolase from Bifidobacterium bifidum in mucin adhesion and aggregation. Appl. Environ. Microbiol. 78, 3992–3998. doi: 10.1128/aem.08024-11
Gordon, V. D., O’Halloran, T. J., and Shindell, O. (2015). Membrane adhesion and the formation of heterogeneities: biology, biophysics, and biotechnology. Phys. Chem. Chem. Phys. 17, 15522–15533. doi: 10.1039/c4cp05876c
Hajam, I. A., Dar, P. A., Shahnawaz, I., Jaume, J. C., and Lee, J. H. (2017). Bacterial flagellin-a potent immunomodulatory agent. Exp. Mol. Med. 49:e373. doi: 10.1038/emm.2017.172
He, Y., Jin, L., Sun, F., Hu, Q., and Chen, L. (2016). Antibiotic and heavy-metal resistance of Vibrio parahaemolyticus isolated from fresh shrimps in Shanghai fish markets. China. Environ. Sci. Pollut. Res. Int. 23, 15033–15040. doi: 10.1007/s11356-016-6614-4
He, Y., Wang, H., and Chen, L. (2015). Comparative secretomics reveals novel virulence-associated factors of Vibrio parahaemolyticus. Front. Microbiol. 6:707. doi: 10.3389/fmicb.2015.00707
Hu, Q., and Chen, L. (2016). Virulence and antibiotic and heavy metal resistance of Vibrio parahaemolyticus isolated from crustaceans and shellfish in Shanghai. China. J. Food Prot. 79, 1371–1377. doi: 10.4315/0362-028x.Jfp-16-031
Jiang, W., Han, X., Wang, Q., Li, X., Yi, L., Liu, Y., et al. (2014). Vibrio parahaemolyticus enolase is an adhesion-related factor that binds plasminogen and functions as a protective antigen. Appl. Microbiol. Biotechnol. 98, 4937–4948. doi: 10.1007/s00253-013-5471-z
Khatoon, N., Jamal, A., and Ali, M. I. (2017). Polymeric pollutant biodegradation through microbial oxidoreductase: a better strategy to safe environment. Int. J. Biol. Macromol. 105, 9–16. doi: 10.1016/j.ijbiomac.2017.06.047
Kim, Y. I., and Cho, J. Y. (2019). Gel-based proteomics in disease research: is it still valuable? Biochim. Biophys. Acta Proteins Proteom. 1867, 9–16. doi: 10.1016/j.bbapap.2018.08.001
Kumar, R., Ahmad, F., and Rathaur, S. (2019). Characterization of filarial phosphoglycerate kinase. Biochimie 165, 258–266. doi: 10.1016/j.biochi.2019.08.012
Lee, P. Y., Chin, S. F., Low, T. Y., and Jamal, R. (2018). Probing the colorectal cancer proteome for biomarkers: current status and perspectives. J. Proteomics 187, 93–105. doi: 10.1016/j.jprot.2018.06.014
Lee, P. Y., Saraygord-Afshari, N., and Low, T. Y. (2019). The evolution of two-dimensional gel electrophoresis-from proteomics to emerging alternative applications. J. Chromatogr. A 1615:460763. doi: 10.1016/j.chroma.2019.460763
Li, L., Meng, H., Gu, D., Li, Y., and Jia, M. (2019). Molecular mechanisms of Vibrio parahaemolyticus pathogenesis. Microbiol. Res. 222, 43–51. doi: 10.1016/j.micres.2019.03.003
Li, P., Kinch, L. N., Ray, A., Dalia, A. B., Cong, Q., Nunan, L. M., et al. (2017). Acute hepatopancreatic necrosis disease-causing Vibrio parahaemolyticus strains maintain an antibacterial Type VI secretion system with versatile effector repertoires. Appl. Environ. Microbiol. 83:e0737-17. doi: 10.1128/aem.00737-17
Liu, G., Zhang, M., Chen, X., Zhang, W., Ding, W., and Zhang, Q. (2015). Evolution of threonine aldolases, a diverse family involved in the second pathway of glycine biosynthesis. J. Mol. Evol. 80, 102–107. doi: 10.1007/s00239-015-9667-y
Liu, J., Bai, L., Li, W., Han, H., Fu, P., Ma, X., et al. (2018). Trends of foodborne diseases in China: lessons from laboratory-based surveillance since 2011. Front. Med. 12, 48–57. doi: 10.1007/s11684-017-0608-6
Lopatek, M., Wieczorek, K., and Osek, J. (2018). Antimicrobial resistance, virulence factors, and genetic profiles of Vibrio parahaemolyticus from seafood. Appl. Environ. Microbiol. 84:e00537-18. doi: 10.1128/aem.00537-18
Lun, J., Xia, C., Yuan, C., Zhang, Y., Zhong, M., Huang, T., et al. (2014). The outer membrane protein, LamB (maltoporin), is a versatile vaccine candidate among the Vibrio species. Vaccine 32, 809–815. doi: 10.1016/j.vaccine.2013.12.035
Luo, L., Matthews, J. D., Robinson, B. S., and Jones, R. M. (2019). Vibrio parahaemolyticus VopA is a potent inhibitor of cell migration and apoptosis in the intestinal epithelium of Drosophila melanogaster. Infect. Immun. 87:e00669-18. doi: 10.1128/iai.00669-18
Luong, T. T., Kim, E. H., Bak, J. P., Nguyen, C. T., Choi, S., Briles, D. E., et al. (2015). Ethanol-induced alcohol dehydrogenase E (AdhE) potentiates pneumolysin in Streptococcus pneumoniae. Infect. Immun. 83, 108–119. doi: 10.1128/iai.02434-14
Machtel, R., Narducci, A., Griffith, D. A., Cordes, T., and Orelle, C. (2019). An integrated transport mechanism of the maltose ABC importer. Res. Microbiol. 170, 321–337. doi: 10.1016/j.resmic.2019.09.004
Makino, K., Oshima, K., Kurokawa, K., Yokoyama, K., Uda, T., Tagomori, K., et al. (2003). Genome sequence of Vibrio parahaemolyticus: a pathogenic mechanism distinct from that of V. cholerae. Lancet 361, 743–749. doi: 10.1016/s0140-6736(03)12659-1
Merrick, M. (2014). Post-translational modification of P II signal transduction proteins. Front. Microbiol. 5:763. doi: 10.3389/fmicb.2014.00763
Mikhalchik, E., Balabushevich, N., Vakhrusheva, T., Sokolov, A., Baykova, J., Rakitina, D., et al. (2019). Mucin adsorbed by E. coli can affect neutrophil activation in vitro. FEBS. Open Biol. 10, 180–196. doi: 10.1002/2211-5463.12770
Mizrachi Nebenzahl, Y., Blau, K., Kushnir, T., Shagan, M., Portnoi, M., Cohen, A., et al. (2016). Streptococcus pneumoniae cell-wall-localized phosphoenolpyruvate protein Phosphotransferase can function as an adhesin: identification of its host target molecules and evaluation of its potential as a vaccine. PLoS One 11:e0150320. doi: 10.1371/journal.pone.0150320
Mohammadi-Barzelighi, H., Nasr-Esfahani, B., Bakhshi, B., Daraei, B., Moghim, S., and Fazeli, H. (2019). Analysis of antibacterial and antibiofilm activity of purified recombinant Azurin from Pseudomonas aeruginosa. Iran. J. Microbiol. 11, 166–176. doi: 10.18502/ijm.v11i2.1083
Moravec, A. R., Siv, A. W., Hobby, C. R., Lindsay, E. N., Norbash, L. V., Shults, D. J., et al. (2017). Exogenous polyunsaturated fatty acids impact membrane remodeling and affect virulence phenotypes among pathogenic Vibrio species. Appl. Environ. Microbiol. 83:e001415-17. doi: 10.1128/aem.01415-17
Nedergaard Pedersen, J., Skov Pedersen, J., and Otzen, D. E. (2018). Liprotides assist in folding of outer membrane proteins. Protein. Sci. 27, 451–462. doi: 10.1002/pro.3337
Osorio, C. R. (2018). T3SS effectors in Vibrios: homology in sequence, diversity in biological functions? Virulence 9, 721–723. doi: 10.1080/21505594.2018.1435965
Pang, H., Li, W., Zhang, W., Zhou, S., Hoare, R., Monaghan, S. J., et al. (2020). Acetylome profiling of Vibrio alginolyticus reveals its role in bacterial virulence. J. Proteomics 211:103543. doi: 10.1016/j.jprot.2019.103543
Park, K., Mok, J. S., Ryu, A. R., Kwon, J. Y., Ham, I. T., and Shim, K. B. (2018). Occurrence and virulence of Vibrio parahaemolyticus isolated from seawater and bivalve shellfish of the gyeongnam coast, Korea, in 2004-2016. Mar. Pollut. Bull. 137, 382–387. doi: 10.1016/j.marpolbul.2018.10.033
Perez-Acosta, J. A., Martinez-Porchas, M., Elizalde-Contreras, J. M., Leyva, J. M., Ruiz-May, E., Gollas-Galvan, T., et al. (2018). Proteomic profiling of integral membrane proteins associated to pathogenicity in Vibrio parahaemolyticus strains. Microbiol. Immunol. 62, 14–23. doi: 10.1111/1348-0421.12556
Perez-Casal, J., and Potter, A. A. (2016). Glyceradehyde-3-phosphate dehydrogenase as a suitable vaccine candidate for protection against bacterial and parasitic diseases. Vaccine 34, 1012–1017. doi: 10.1016/j.vaccine.2015.11.072
Proudfoot, M., Kuznetsova, E., Brown, G., Rao, N. N., Kitagawa, M., Mori, H., et al. (2004). General enzymatic screens identify three new nucleotidases in Escherichia coli. biochemical characterization of SurE, YfbR, and YjjG. J. Biol. Chem. 279, 54687–54694. doi: 10.1074/jbc.M411023200
Radchenko, M., and Merrick, M. (2011). The role of effector molecules in signal transduction by PII proteins. Biochem. Soc. Trans. 39, 189–194. doi: 10.1042/bst0390189
Raszl, S. M., Froelich, B. A., Vieira, C. R., Blackwood, A. D., and Noble, R. T. (2016). Vibrio parahaemolyticus and Vibrio vulnificus in South America: water, seafood and human infections. J. Appl. Microbiol. 121, 1201–1222. doi: 10.1111/jam.13246
Rivera, I. G., Chowdhury, M. A., Huq, A., Jacobs, D., Martins, M. T., and Colwell, R. R. (1995). Enterobacterial repetitive intergenic consensus sequences and the PCR to generate fingerprints of genomic DNAs from Vibrio cholerae O1, O139, and non-O1 strains. Appl. Environ. Microbiol. 61, 2898–2904. doi: 10.1007/BF00873103
Rybniker, J., Pojer, F., Marienhagen, J., Kolly, G. S., Chen, J. M., van Gumpel, E., et al. (2014). The cysteine desulfurase IscS of Mycobacterium tuberculosis is involved in iron-sulfur cluster biogenesis and oxidative stress defence. Biochem. J. 459, 467–478. doi: 10.1042/bj20130732
Salomon, D., Gonzalez, H., Updegraff, B. L., and Orth, K. (2013). Vibrio parahaemolyticus type VI secretion system 1 is activated in marine conditions to target bacteria, and is differentially regulated from system 2. PLoS One 8:e61086. doi: 10.1371/journal.pone.0061086
Salomon, D., Kinch, L. N., Trudgian, D. C., Guo, X., Klimko, J. A., Grishin, N. V., et al. (2014). Marker for type VI secretion system effectors. Proc. Natl. Acad. Sci. U.S.A. 111, 9271–9276. doi: 10.1073/pnas.1406110111
Shin, J. M., Hwang, Y. O., Tu, O. J., Jo, H. B., Kim, J. H., Chae, Y. Z., et al. (2013). Comparison of different methods to quantify fat classes in bakery products. Food Chem. 136, 703–709. doi: 10.1016/j.foodchem.2012.08.033
Silva, R., Aguiar, T. Q., Oliveira, C., and Domingues, L. (2019). Physiological characterization of a pyrimidine auxotroph exposes link between uracil phosphoribosyltransferase regulation and riboflavin production in Ashbya gossypii. Nat. Biotechnol. 50, 1–8. doi: 10.1016/j.nbt.2018.12.004
Smith, C. D., Chattopadhyay, D., and Pal, B. (2011). Crystal structure of Plasmodium falciparum phosphoglycerate kinase: evidence for anion binding in the basic patch. Biochem. Biophys. Res. Commun. 412, 203–206. doi: 10.1016/j.bbrc.2011.07.045
Tang, J., Jia, J., Chen, Y., Huang, X., Zhang, X., Zhao, L., et al. (2018). Proteomic analysis of Vibrio parahaemolyticus under cold stress. Curr. Microbiol. 75, 20–26. doi: 10.1007/s00284-017-1345-4
Theodoulou, F. L., and Kerr, I. D. (2015). ABC transporter research: going strong 40 years on. Biochem. Soc. Trans. 43, 1033–1040. doi: 10.1042/bst20150139
Thoma, J., Ritzmann, N., Wolf, D., Mulvihill, E., Hiller, S., and Muller, D. J. (2017). Maltoporin LamB unfolds beta hairpins along mechanical stress-dependent unfolding pathways. Structure 25, 1139–1144. doi: 10.1016/j.str.2017.05.010
Thongjun, J., Mittraparp-Arthorn, P., Yingkajorn, M., Kongreung, J., Nishibuchi, M., and Vuddhakul, V. (2013). The trend of Vibrio parahaemolyticus infections in Southern Thailand from 2006 to 2010. Trop. Med. Health 41, 151–156. doi: 10.2149/tmh.2013-06
Wang, R., Sun, L., Wang, Y., Deng, Y., Fang, Z., Liu, Y., et al. (2018). Influence of food matrix type on extracellular products of Vibrio parahaemolyticus. BMC Microbiol. 18:65. doi: 10.1186/s12866-018-1207-7
Wang, Y. T., Huang, H. Y., Tsai, M. A., Wang, P. C., Jiang, B. H., and Chen, S. C. (2014). Phosphoglycerate kinase enhanced immunity of the whole cell of Streptococcus agalactiae in tilapia, Oreochromis niloticus. Fish Shellf. Immunol. 41, 250–259. doi: 10.1016/j.fsi.2014.09.008
Wilson, D. N. (2014). Ribosome-targeting antibiotics and mechanisms of bacterial resistance. Nat. Rev. Microbiol. 12, 35–48. doi: 10.1038/nrmicro3155
Wilson, D. N. (2016). The ABC of ribosome-related antibiotic resistance. mBio 7:e0598-16. doi: 10.1128/mBio.00598-16
Xu, M., Wu, J., and Chen, L. (2019). Virulence, antimicrobial and heavy metal tolerance, and genetic diversity of Vibrio cholerae recovered from commonly consumed freshwater fish. Environ. Sci. Pollut. Res. Int. 26, 27338–27352. doi: 10.1007/s11356-019-05287-8
Yang, C., Zhang, X., Fan, H., Li, Y., Hu, Q., Yang, R., et al. (2019). Genetic diversity, virulence factors and farm-to-table spread pattern of Vibrio parahaemolyticus food-associated isolates. Food Microbiol. 84:103270. doi: 10.1016/j.fm.2019.103270
Yang, B., Zhang, D., Wu, T., Zhang, Z., Raza, S. H. A., Schreurs, N., et al. (2019). Maltoporin (LamB protein) contributes to the virulence and adhesion of Aeromonas veronii TH0426. J. Fish Dis. 42, 379–389. doi: 10.1111/jfd.12941
Yang, W., Ding, D., Zhang, C., Zhou, J., and Su, X. (2015). iTRAQ-based proteomic profiling of Vibrio parahaemolyticus under various culture conditions. Proteome Sci. 13:19. doi: 10.1186/s12953-015-0075-4
Yang, Y., Xie, J., Li, H., Tan, S., Chen, Y., and Yu, H. (2017). Prevalence, antibiotic susceptibility and diversity of Vibrio parahaemolyticus isolates in seafood from south China. Front. Microbiol. 8:2566. doi: 10.3389/fmicb.2017.02566
Yao, J., and Rock, C. O. (2017). Exogenous fatty acid metabolism in bacteria. Biochimie 141, 30–39. doi: 10.1016/j.biochi.2017.06.015
Zhang, L., and Orth, K. (2013). Virulence determinants for Vibrio parahaemolyticus infection. Curr. Opin. Microbiol. 16, 70–77. doi: 10.1016/j.mib.2013.02.002
Zhang, X., Xiao, F., and Li, S. (2018). China Fishery Statistical Yearbook 2018. Beijing: China Agriculture Press.
Zhong, Q., Wang, B., Wang, J., Liu, Y., Fang, X., and Liao, Z. (2019). Global proteomic analysis of the resuscitation state of Vibrio parahaemolyticus compared with the normal and viable but non-culturable state. Front. Microbiol. 10:1045. doi: 10.3389/fmicb.2019.01045
Zhu, C., Sun, B., Liu, T., Zheng, H., Gu, W., He, W., et al. (2017). Genomic and transcriptomic analyses reveal distinct biological functions for cold shock proteins (VpaCspA and VpaCspD) in Vibrio parahaemolyticus CHN25 during low-temperature survival. BMC Genomics 18:436. doi: 10.1186/s12864-017-3784-5
Zhu, Z., Dong, C., Weng, S., and He, J. (2019). Identification of outer membrane protein TolC as the major adhesin and potential vaccine candidate for Vibrio harveyi in hybrid grouper, Epinephelus fuscoguttatus (female symbol) x E. lanceolatus (male symbol). Fish Shellf Immunol. 86, 143–151. doi: 10.1016/j.fsi.2018.11.037
Keywords: Vibrio parahaemolyticus, secretome, proteome, virulence, resistance, aquatic products
Citation: Zhu Z, Yang L, Yu P, Wang Y, Peng X and Chen L (2020) Comparative Proteomics and Secretomics Revealed Virulence and Antibiotic Resistance-Associated Factors in Vibrio parahaemolyticus Recovered From Commonly Consumed Aquatic Products. Front. Microbiol. 11:1453. doi: 10.3389/fmicb.2020.01453
Received: 23 January 2020; Accepted: 04 June 2020;
Published: 14 July 2020.
Edited by:
Arun K. Bhunia, Purdue University, United StatesReviewed by:
Pendru Raghunath, Texila American University, GuyanaJessica L. Jones, United States Food and Drug Administration, United States
Copyright © 2020 Zhu, Yang, Yu, Wang, Peng and Chen. This is an open-access article distributed under the terms of the Creative Commons Attribution License (CC BY). The use, distribution or reproduction in other forums is permitted, provided the original author(s) and the copyright owner(s) are credited and that the original publication in this journal is cited, in accordance with accepted academic practice. No use, distribution or reproduction is permitted which does not comply with these terms.
*Correspondence: Lanming Chen, lmchen@shou.edu.cn