- 1Department of Neurosurgery, Institute of Medical Sciences, University of Rzeszów, Rzeszów, Poland
- 2Department of Neurosurgery, Faculty of Medicine and Saarland University Medical Center, Saarland University, Homburg, Germany
- 3Department of Pathophysiology, Institute of Medical Sciences, University of Rzeszów, Rzeszów, Poland
- 4Chair of Internal Medicine and Department of Internal Medicine in Nursing, Faculty of Health Sciences, Medical University of Lublin, Lublin, Poland
In the past, water homeostasis of the brain was understood as a certain quantitative equilibrium of water content between intravascular, interstitial, and intracellular spaces governed mostly by hydrostatic effects i.e., strictly by physical laws. The recent achievements in molecular bioscience have led to substantial changes in this regard. Some new concepts elaborate the idea that all compartments involved in cerebral fluid homeostasis create a functional continuum with an active and precise regulation of fluid exchange between them rather than only serving as separate fluid receptacles with mere passive diffusion mechanisms, based on hydrostatic pressure. According to these concepts, aquaporin-4 (AQP4) plays the central role in cerebral fluid homeostasis, acting as a water channel protein. The AQP4 not only enables water permeability through the blood-brain barrier but also regulates water exchange between perivascular spaces and the rest of the glymphatic system, described as pan-cerebral fluid pathway interlacing macroscopic cerebrospinal fluid (CSF) spaces with the interstitial fluid of brain tissue. With regards to this, AQP4 makes water shift strongly dependent on active processes including changes in cerebral microcirculation and autoregulation of brain vessels capacity. In this paper, the role of the AQP4 as the gatekeeper, regulating the water exchange between intracellular space, glymphatic system (including the so-called neurovascular units), and intravascular compartment is reviewed. In addition, the new concepts of brain edema as a misbalance in water homeostasis are critically appraised based on the newly described role of AQP4 for fluid permeation. Finally, the relevance of these hypotheses for clinical conditions (including brain trauma and stroke) and for both new and old therapy concepts are analyzed.
1. Introduction
Apart from the exchange of information, one of the most challenging tasks of the mammalian brain is to maintain the internal water and electrolyte homeostasis independent from the caprices of the external environment, in order to provide the neurons with nourishing substances and guarantee them a constancy of electrolyte concentration and osmolarity, required for their proper function (1, 2). As the modern techniques of histopathological and physiological research developed, the various tasks regarding global cerebral function have been attributed to the different cellular and acellular components of the brain tissue. Here, the neurons as the cells generating and propagating electrical impulses (which is considered as the major task of the whole brain), have been accorded the exclusive role of managing the information. Meanwhile, other brain components only play a minor role in maintaining the intracellular and molecular environment in optimal conditions for the appropriate function of the fastidious neural cells (3, 4). For instance, according to the common perception, the extracellular compartment is merely a vast space filled with a quite homogenous fluid, consisting mostly of water, substrate molecules, and the products of both the neuronal and glial metabolism floating together with nourishing vessels (2).
Certainly, this oversimplification is far from even approximating the whole complexity of the structure of brain fluid spaces, not to mention its extremely composed function regarding cerebral water turnover. The multidisciplinary research of recent years has delivered solid evidence that the intracerebral water balance is a highly complex, actively regulated process, involving all types of glia cells as well as the neurons and being highly responsible for the electrolyte and water homeostasis of the latter, thus impacting significantly the proper function of the whole central nervous system as a physiological unit (2, 4–6).
Due to a variable number of (sometimes concurring) theories, it is impossible to outline all neurobiological concepts describing how the brain water homeostasis is maintained in the limited text volume of the journal paper. Thus, the main goal of this narrative review is to provide the Reader with the critical appraisal of some of the latest ideas, which attempt to unify the recent findings in (micro-)anatomy, molecular neurophysiology, and biophysics into the form of a concise model of brain fluid turnover. In particular, the concept of the glymphatic system, conjoining the anatomic spaces filled with cerebrospinal fluid and the ultrastructures of extracellular space needs to be outlined (2, 7–10). The common denominator of all these theories is the function of cellular membrane components, called water channel proteins. Among these, particular attention was paid recently to the structure and function of aquaporins (AQPs), where aquaporine-4 (AQP4) has been acknowledged as the water channel protein of main importance for water turnover in the mammalian brain (11). First, recognized as a passive water channel, due to results of numerous neuromolecular studies, AQP4 has recently been acknowledged as an active and precise water homeostasis regulator, playing a crucial role both in physiological conditions as well as in situations where the exchange of fluids between all cerebral compartments is essential for the course of the disease (12–15). Here, the prime example is the development and subsidence of brain edema, being the major manifestation of the secondary cerebral damage in traumatic brain injury and in cerebral ischemia (16–18). For this reason, the potential of AQP4 as the target point for therapeutic methods will also be discussed.
2. Concepts of Cerebral Integrated Water Space
With the advent of modern neurosurgery, several concepts of cerebral fluid circulation and water turnover have been developed with the classic model of cerebrospinal fluid (CSF) flow also termed “third circulation” published by Cushing, which has since then become universally accepted (2, 19). According to his view, the brain was enveloped by the CSF layer being in constant flow. The CSF is produced in the lateral ventricles/choroid plexi, transported to the third ventricle, passing through the aqueduct and fourth ventricle, flowing to basal cisterns and distributed upon both hemispheres, where a paramedial area (superior sagittal sinus and arachnoid granulations) plays a major role in CSF reabsorption (2, 20). Already an important remark has been made, that the brain, despite its high water content lacks a usual lymphatic apparatus and lymph flow, and the CSF circulation was assumed to fulfill the role of the lymphatic circulation (provision and cleavage of water-soluble metabolites) in the brain (7, 21, 22). This macroscopic and very gross description of CSF turnover has been modified recently. In particular, the view that CSF production and resorption are the main forces behind brain fluid transportation needed to be revised (23–30). Here, the importance of perivascular spaces, called Virchow-Robin spaces (VRS) should be outlined. These fluid-filled areas, surrounding both arteries and veins running in the direct proximity or through the nervous tissue was attributed the role of the intermediate zone, joining the macroscopical subpial space, filled with CSF with the microscopically delineated extracellular area, in which single brain cells, including neurons and glia, were sustained (12, 31). Of note, in several studies, it was demonstrated that the fluid contained in VRS is moved not by simple diffusion or only due to a high pressure gradient, but is rather propelled by the pulsatile activity of arterial vessels (32–36). Such a pump mechanism seems to depend upon the cerebral microcirculation (37, 38) and the condition of disturbed vascular autoregulation impairs also the mechanism of bulk flow along the VRS (39–45). On the other hand, the raise of cerebral blood flow on the level of microcirculation can increase the dynamics of perivascular fluid (37). Clearly, cerebral microcirculation in physiological conditions relies on the metabolic demand of the nervous tissues, supplied by both blood and cerebral fluid flow (46–48). In respect to complex interactions between the cerebral vessels (including cerebral vasculature i.e., endothelial cells and pericytes, as well as astrocytes and neurons with their processes), the term neurovascular unit (NVU) has been coined. The concept of an NVU [exhaustively reviewed in (49)] encompasses these varieties of cells and their function, the interactions of which maintain the ionic, metabolic, and molecular homeostasis of the brain. In particular, the neuronal and astrocytic activity is able to provoke a dilation or a contraction of the arterial vessels [executed by smooth muscle cells (50)] or capillaries [provided by pericytes, being an integral part of NVU (51)] via a number of mediator substances, the release of which is strictly dependent on neuronal or astrocytic activity. This list includes not only the nitric oxide (NO), as the prime example of vasoactive substance (52, 53), but also products of cyclooxygenase-2 activity (prostanoids) (54, 55), D-serine of astrocytic origin (56), peptide-based vasoactive mediators including vasopressin (57), somatostatin (58), neuropeptide Y (NPY) (59, 60), and vasoactive intestinal peptide (VIP) (61), all of which the neurons or astrocytes are capable of secreting. This means, that depending on the current activity of the neurons, the autoregulation of the cerebral blood flow (on the level of microcirculation/NVU) would be able to adapt not only the blood supply but also, indirectly the control of CSF and the extracellular fluid extravasal flow (62).
Though the view of arteries and arterioles and their pulsatile action as the main pumping mechanism for cerebral fluid movement is quite straightforward and easy to understand, several physiological observations undermine this simplified concept of brain fluid mechanics (63). Here, the oscillating or even retrograde flow along VRS has been postulated and documented in several in vivo experiments (64, 65), drawing a conclusion that additional mechanisms exist (possibly on the molecular level) which contribute to the production, mixing, and flow of the fluid on the level of cerebral extracellular spaces. One of the most important factors is the temporal change in permeability for water and electrolytes or even larger particles of the blood-brain barrier (BBB) (66–69). The BBB, with its key component—tight junctions between endothelial cells lining the interior wall of cerebral microcirculation, used to be perceived as a seal, which prevented larger molecules from passing between the intravascular lumen and extracellular space. In this early concept, water and electrolytes were allowed to pass the BBB depending mostly on physical and chemical laws of osmosis and hydrostatic pressure (70, 71). However, the idea of BBB as the passive membrane exposed to the tides of CSF and blood circulation has been revised recently. Here, the exchange of electrolytes and larger particles (e.g., aminoacids) across the BBB has been described as an active, closely regulated process (72, 73), dependent on the energetic state of neurons, astrocytes, and endothelial cells (74, 75). The key argument, that BBB is not a passive, but an active structure, regulating the circulation of cerebral fluid on the ultramicroscopic level, was the capability of BBB to precisely regulate the amount of water passing across it. Moreover, in relation to water permeability, the BBB demonstrated high dynamics in changes of this property, both temporal and spatial (76–79). Thus, due to the rapid and physiological changes in BBB permeability to water and electrolytes, the brain can create compartments of fluid spaces, slightly but significantly different from the rest of global fluid space (80–83), in order to create a biochemical environment that is optimally adjusted to the current needs of the population of brain cells, both neurons and glia. Certainly, this dynamic function requires the presence of multiple molecular control systems, responsible for rapid changes in the transmission rate across BBB for different compounds (84). Regarding water permeability, the major control system is composed of several membrane proteins, labeled water channel proteins, with the AQP4 being appreciated as the most relevant for cerebral water turnover (85). The physiological function of AQP4 clearly results from its biochemical structure and gene expression as is described in the following chapter.
3. Structure, Genetics, and Distribution of AQP4
The AQP4 protein is a member of the large family of AQPs, the membrane water channels, which are widespread in all investigated organisms from bacteria and plants to vertebrates and responsible for bidirectional water permeability of phospholipid bilayers of cells (86). The AQP4 was first identified as 32-kDa mercurial-insensitive water channel in a rat lung (87) and then described in many different epithelial cells such as renal principal cells of collecting ducts, retina, iris, ciliary body, stomach parietal cells, colon epithelial cells, excretory tubules of lacrimal and salivary glands, organ of Corti, and in skeletal muscles. But it is mostly present in the mammalian brain and spinal cord, where it is localized in astrocytes directly in contact with capillaries and pia and in subpopulations of ependymal cells (88–91).
3.1. AQP4 Protein Structure
The structure of the monomeric subunit of AQP4 is similar for all AQPs and was at first described for AQP1 in human erythrocytes membrane (92). Any single subunit comprises two repeated segments, each built from three domains of the alpha-helix structure. All six domains (in pairs of the three) are arranged in the form of a non-polar bilayer and connected by five loops (A to E). The loops B and E (which connect the second and third domain in each segment) consist of highly conserved located motifs of three amino acids: asparagine—proline—arginine (NPA). According to the hourglass model, they cover the space between the bilayer leaflets and allow the water pore formation (92–94). The hemipore (as are also called B and E loops) is maintained by the van der Waals forces (95). The width of the pore along its lumen is not identical. The narrowest part, localized about 8Å above the center of the membrane, has a diameter of 2.8Å, similar to a single particle of water. In this site, NPA motifs make contact with each other. The pore diameter increases in the direction of the extra and intracellular layer of the membrane, which creates the hourglass-like shape of the whole structure (96). Several isoforms of AQP4 have been identified. In the rat brain, Jung et al. described two overlapping polypeptides of 323 or 301 amino acids, currently known as classical forms M1 and M23, transcribed from this same gene, but from differently localized initiation sites at the upstream (M1) and downstream (M23) of the gene. Authors have determined a polypeptide structure, similar to earlier identified AQP1, consisting of six membrane bilayer-spanning domains and five connecting loops, including hydrophobic loops B and E and containing, respectively, NPA 97–99 and NPA 213–216 sequences. The cytoplasmic amino terminus comprises both potential initiation sites, the carboxyl terminus, also localized in the cytoplasm consists of approximately 70 amino acids. Opposite to other AQPs, in the AQP4 amino acid chain no cysteine at site G94 nor at site A210, both responsible for mercurial inhibition, was found. In the amino acid sequence also three potential N-glycosylation sites were identified with the first (N153) localized in extracellular loop C. Both protein isoforms were synthesized in the presence of microsomes. When cRNA contained the downstream site, a single polypeptide of 301 amino acids and 30 kDA arose. In the presence of both initiation sites, besides the minor product, also the 323 amino acids polypeptide of 32 kDa were synthesized (93). Together with these first two AQP4 isoforms identified in humans, rats, and mice (87, 93, 97), nine AQP4 isoforms are as yet found (AQPa–f, 4, a ex and c ex) (98–100). When, as a result of the AQP4 rat gene mapping, four additional forms of AQP4 were described, the new terminology was implemented. M1 and M23 isoforms, respectively, have received names AQP4a and AQP4c, and AQP4 isoforms newly identified in rats were named AQPb and e-f (101). AQP4a, AQP4c, and AQP4e, considered classic, have six bilayer-spanning domains (1–6) and five interconnecting loops (A–E). AQP4b, AQP4d, AQP4f isoforms are devoid of helices 4 and 5 as well as connecting loop D. AQP4Δ found in human skeletal muscles is devoid of the terminal part of helix 5 and loop E (94). The recently identified isoforms of AQP4 in humans named a ex and c ex are characterized by C—terminal extension containing 29 amino acids (102). The AQP4 monomers independently of the isoform are organized into more complex structures in the form of tetramers, which additionally aggregate into orthogonal arrays of particles (OAPs) considerably various in respect of the size and shape as well as the isoform content (100, 103). The size of OAPs diameter evaluated by different microscopic methods reaches 100–500 nm (100) and the molecular weight of these higher-order structures is about 1,000 kDa (104). AQP4 a and AQP4 c are both incorporated into OAPs (105) as well as their extended variants AQPa ex and AQP4c ex (102). Additionally, it was reported that AQP4 a is able to attach to OAPs only in the presence of AQP4c, being the component of the OAPs core (106) and AQP4 c ex by the limitation of incorporated tetramers affect the size of OAPs (102). The AQP4e undergoes the incorporation into OAPs, while AQPs b and d do not (although both indirectly modulate the OAPs amount) and AQP4 f was not yet evaluated (100, 101, 103). Similarly, AQP4Δ lacks the ability to be attached to OAPs, but in the endoplasmatic reticulum, it exerts an effect limiting both the abundance and size of OAPs. This dominant-negative modulation is imposed through the interactions between AQP4 isoforms of the plasma membrane (99, 100).
3.2. AQP4 Gene Arrangement
All AQP4 isoforms are coded by a single copy of the gene localized in humans on chromosome 18 at the junction of q11.2 and q 12.1 (97, 98). As with other AQPs, the gene coding AQP4 consists of four exons including, respectively, 127, 55, 27, and 92 amino acids, between which three introns of 0.8, 0.3, and 5.2 kb are located. The unique feature, distinguishing the AQP4 gene from other AQPs genes is an alternative initiation sequence situated 2.7 kb upstream and named exon 0. It allows, after the splicing process, to encode the M1 and next 10 amino acids by exon 0 and subsequent 11 amino acids with M23 by exon 1 (97). In the promoter region, such regulatory elements as TATAAAA (TATA box) at 385 bp upstream from initiation codon, one CAAT box, and AP-1 were identified and additionally SP1, two E-boxes, two AP-2, and acute phase response elements (APRE). It was shown that the transcription initiation site is located at 46 bp downstream from the TATA box. In addition, it was revealed that at 138 bp downstream of the stop codon a sequence AATAAA is situated which is the signal of polyadenylation (107). The mRNA of AQP4 b, d, and f is formed after alternative splicing omitting exon 2 from AQP4 a, c, and e, respectively (101, 107). The AQP4Δ mRNA is alternatively spliced from AQP4 a with a lack of exon 4 (99). The variants AQP4 a and c ex are extended through translational readthrough (102). In the AQP4 gene, numerous polymorphic sites were reported across the entire gene including coding and non-coding regions, as well as 3' and 5', flanking regions (108), but the gene is considered as highly conservative and non-synonymous single nucleotide polymorphisms (nsSNPs) are rather rare (approximately 1–2% allele frequencies) (109). Several known nsSNPs influence the protein structure and function. The occurrence of variants I128T, D184E, I205L, M224T, and M278T, although all are localized relatively far from the NPA motifs, affect protein stability. The Ile-Thr substitution in position 128 results in the change of hydrophobic to hydrophilic residue in the transmembrane region and Met-Thr substitution exerts a similar effect in a loop if it involves position 224 or the C—terminal domain and position 278. Additionally, the substitution Met—Thr deprives the amino–acid residue of a sulfur atom. The chemical relevance of two other substitutions Asp—Glu and Ile—Leu is less significant. Nevertheless, all five nsSNPs impact the AQP4 function—I128T, D184E, I205L, M224T reducing, and M278T increasing water permeability (109).
3.3. AQP4 Distribution
As it was mentioned AQP4 is found predominantly in the astrocytes, but the AQP4 gene expression is different in various areas of the central nervous system (CNS) with the highest levels detected in astrocytes localized near the subarachnoid space, along ventricles and blood vessels. Also in areas engaged with water balance maintaining and responsible for the osmoregulation such as the supraoptic nucleus or subfornical organ, the intense AQP4 expression was recorded (90). The distribution of AQP4 isoforms inside astrocytes varies depending on the individual isoform. The most accurately is determined for AQP4a and AQP4c (known also as M1 and M23), being two first described and best-investigated isoforms. Both of them as well as their extended forms (AQP4a ex and AQP4c ex) were found at the plasma membrane aggregated in OAPs with the isoform c in the core of OAP and isoform an attached to c (98, 100, 105, 110). The isoform a may also occur in the plasma membrane in the simpler form of tetramers (111). The isoform e is localized not only at the plasma membrane, but also intracellularly (100, 101). Other isoforms were detected only in the intracellular structures such as Golgi apparatus (isoforms b,d, and f) or endoplasmatic reticulum (Δ4) (99, 100). Additionally, isoforms b and d were found in lysosomes and early endosomes (100, 103).
Several studies underlined the fact, that the regulation of AQP4 activity relies more on the subcellular relocation than on the expression of its gene. Both isoforms of AQP4 can be translated from the same full-length transcript by a “leaky scanning” mechanism (112, 113). Previous evidence shows that both isoforms are relocated equally and that the surface localization of AQP4 increased without changing the level of protein expression. In a study by Salman et al. mild hypothermic treatment increased the surface localization of AQP4 in human astrocytes even in the lack of significant change in total protein expression levels. Here, AQP4 mRNA increased modestly in cultured human primary astrocytes following 4 h mild hypothermia (32°C) compared with control cells grown at 37°C but this increase in transcript did not result in a change in protein level. Nevertheless, the decrease in temperature influenced the surface localization of AQP4, creating a space for the potential use of therapeutic brain hypothermia as an antiedematous treatment (114). Furthermore, analysis of Ciappelloni et al. indicated that the deleterious effect of anti-AQP4 autoantibodies involved in neuromyelitis optica (NMO) is probably based on perturbation of AQP4 surface dynamic and distribution. This impact differed between both isoforms of AQP4. Notably, in this study, the water transporting function of single AQP4 molecules remained intact despite exposition to AQP4 antibodies. This puts the nanoscale distribution of AQP4 in the spotlight as a major pathophysiological mechanism and the target for potential therapeutic strategy (15, 115), see also Chapter 6.
4. Aquaporin 4: Its Physiological Function
The biochemical and molecular properties of AQP4 including its expression, assembly of subunits, and integration into organelle clearly define it as one of the membrane proteins. Indeed, the proper physiological function of AQP4 requires its polarized integration and anchoring into astrocytic cell membranes (116–119) and this process is regulated already at the stage of translation and protein folding (120). In particular, the location of the AQP4 along the parts of astrocytic membranes reflects its crucial function in regulating the water exchange between intra- and extravascular space: the density of AQP4 arrays is about 10 times higher in endfeet areas adjacent to cerebral microvasculature than in other zones (90, 117, 121) and this inhomogeneous localization seems to be crucial for the BBB integrity (122, 123). But even if the majority of AQP4 complexes are located in endfoot areas, the presence of AQP4 has also been demonstrated in astrocytic membrane zones, directly neighboring synaptic areas (124, 125), in particular excitatory synapses (90). This localization of AQP4 defines its main physiological functions: a direct impact on the clearance of water and cellular metabolites, altering extracellular fluid dynamics, and (most probably indirect and less precisely described) regulation of neuronal and synaptic activity including plasticity (thus impacting memory and behavior). Certainly, the role of AQP4 and the whole AQP family in the physiology of the nervous system is not limited to these two domains. Currently, up to 13 different AQPs have been identified. The diversity of their physiological roles comprises physiological solute transport including glycerol, ammonia, urea, carbon dioxide, and hydrogen peroxide (126). The permeability of water channels for different small, polar substrates depends not exclusively on transmembrane proteins, which form a more narrow or wider space but expresses considerably more complex interactions between the features of the solute as well as the pore constriction and polarity. Especially important in the highlighting of these phenomena seems to be recently described relevance between the single amino acid substitutions within the aromatic/arginine (ar/R) motifs known as the selectivity filters of different AQPs and between glycerol and urea permeability. In AQP4 the ar/R- motif is formed by phenylalanine in position 1, histidine, in position 2, and, being a small residue, alanine in position 3. In vitro, the mutagenesis of ar/R motifs of AQP4 consisting in substitution of histidine in position 2 and arginine in position 4 creates glycerol or urea permeable channels. The H201A and H206G substitutions, respectively, allow the glycerol and the urea permeable channels to form, while the R216A substitution creates the channel permeable for both substrates. Some authors hypothesized that the H201A mutation along with F77 composes a hydrophobic corner contacting with the alkyl chain of the glycerol due to van der Waals forces, while the loss of the alanine in the H201G mutation causes a disruption of this corner and accessibility of the V197 backbone carbonyl group for binding with water or solutes such as urea due to hydrogen bounds. Oppositely, analogous mutagenesis of AQP1 (R195A and H180/G) did not lead to the formation of urea or glycerol permeable channels (127). AQPs are also responsible for the trafficking of other membrane proteins and are involved in intercellular molecular interactions resulting in cell-cell adhesions. Due to their selectivity in ion transfer across the cell membrane and ability to counteract the osmotic changes, AQP has been attributed the role of cell volume/size regulators. As to the AQP4 itself, its role in cell adhesion (probably by facilitating aggregation or localization of other adhesion molecules) has been previously described (128, 129). For the exhaustive reviews on diversity in AQP family and AQP4 function see also (13, 130, 131), however for the sake of clarity and clinical context of this review we will focus on the AQP4 functions that are the most relevant for the function of the perivascular unit.
4.1. Role of AQP4 in Fluid Management
The information that is crucial for understanding AQP4 function for fluid homeostasis has been mostly (but not exclusively) gained through studies implementing animal lines with the genetic modification of AQP4 function. Accordingly, AQP4 knockout animals demonstrate enlarged interstitial fluid spaces (132, 133), increased brain water content (134, 135), and reduced capability to get rid of extracellular brain water excess (135, 136). These findings are highly suggestive of a regulatory role of AQP4 in water transportation across BBB between extracellular and perivascular space (137). Indeed, multiple attempts to trace the fluid movement demonstrated suppression of glymphatic flow in the absence of AQP4. Of note, this observation has been made not only in regard to exogenous, drug-like substances as mannitol (138) or dextran (137) but also applied to endogenous substances like tau (139–141), beta-amyloid (138, 140, 142, 143) or lipoproteins (144), which are involved in the pathogenesis of degenerative encephalopathies. Of note, the AQP4 role in facilitating the exchange of solute distribution and waste substance clearance is strongly dependent on adequate localization of AQP4 in the perivascular processes (145). Disturbance in the cell-level distribution of AQP4, as provoked by syntrophin-1-alpha (Snta-1) gene deletion (146) or seen in brains affected by aging (147), trauma (148), or ischemic damage (149) is related to impaired function of glymphatic clearance. Undoubtedly, it sheds new light on the role of the glymphatic system in the pathophysiology of diseases such as Alzheimer's disease or posttraumatic neurodegeneration.
Notably, based on the results of (150) and (151) a competitive hypothesis has emerged, assuming that an alternative, AQP4 independent system of fluid transportation exists. In both experiments, implementing alternative ways of tracer administration to the extracellular fluid space in experimental animals, the fluid/tracer transportation was not impacted by the AQP4 genetic status and thus by aquaporin function in both wildtype and AQP4-knockout animals.
However, the recent multicenter research effort, provided by five laboratories implementing independently developed transgenic animal models with impaired AQP function, clearly demonstrated, that transport of the tracers, cleared from extracellular space via perivascular fluid compartment is strongly dependent on the proper function of perivascular aquaporins (146).
In conclusion, the main and widely accepted role of AQP4 is the facilitation of fluid exchange between the extracellular space and the perivascular spaces (both being essential parts of the glymphatic system and incorporated in brain fluid circulation) as well as in the cleavage of several cerebral metabolites, crucial in pathophysiology of neurodegeneration. Importantly, even under the physiological condition, transportation of cerebral fluid does not represent a steady-state but is a very dynamic process constantly adapting to the current needs, being related to the energetic state of neurons and thus linked to autoregulation of microvasculature. Let us take a closer look at the previous evidence regarding this area.
4.2. AQP4 as a Potential Regulator of Glymphatic Flow
Soon after describing glymphatic system with the continuous fluid flow as its main function, the evidence about its dynamic adaptation to the current physiological status appeared. Of importance, the increased energetic demand of neurons on the one hand clearly increases cerebral blood flow on the level of microcirculation (152–155) [a phenomenon described as neurovascular coupling, for some recent reviews of molecular background, see also (156–159)], but on the other hand reduction of interstitial flow as the neuronal activity grew has been observed (160). More so, the conditions, that are clearly related to reduced neuronal activity i.e., sleep (161–163) and general anesthesia (164–167)—albeit in a dose-dependent manner (168) [reviewed also recently in (169, 170)]—have been associated with the enhanced glymphatic flow and interstitial fluid circulation.
Is the activity of AQP4 channels somehow responsible for this inversed relationship between neurovascular coupling and glymphatic flow? Indeed, the trend to the physiological flow reduction in regions of neuronal activation was reversed in AQP4 knockouts (171). AQP4 expression and polarization are also strongly dependent on circadian rhythm (162, 172), suggesting that proper AQP4 activity is required for physiological glymphatic stagnancy in periods/areas of neuronal excitation. Also, in clinical conditions, an increased volume of extracellular fluid/PVS spaces [as seen in AQP4 knockout animals (132, 133)] have been observed in subjects affected by neurodegenerative conditions with documented reduced daily cognitive activity (41). The linkage between neuronal excitation, increased blood microcirculation, and reduced glymphatic flow is not completely understood, but the properties of AQP4 allow us to hypothesize several interrelations between these physiological phenomena. One possibility is the direct impact of vasoactive substances on AQP4 function and expression. Indeed, NO was able to modulate AQP4 expression in cultured astrocytes via a cGMP-/ MAPK controlled mechanism (173, 174) as well as in the setting of animal experiments (175). Also, vasopressin an activation of its receptors seems to impact the density and function of AQP4 (176, 177) or AQP1 (178) channels. Finally, inflammatory vasoactive substances as thromboxane (179) seem to share AQP4 as the parallel lever of action triggering astrocytic swelling. However, some more direct and swifter response mechanisms of AQP4 response to increased neuronal activity do exist. Here, the participation of AQP4 channels in moderating the K+ exchange related to increased neuronal activity needs to be discussed [albeit some reports deny the importance of Kir4.1/AQP4 complex for the mechanism of astrocytic swelling (180), being proposed as the mechanism of the reduced glymphatic flow (181)]. The participation of AQP4 channels in potassium homeostasis is well-documented [as reviewed exhaustively in (130) and (182)] and relies mostly on providing the water flux necessary for spatial redistribution of K+ ions, released during the phase of neuronal activation (183). Importantly, the key role of AQP4 in managing K+ excess has been underlined by molecular studies in conditions directly related to neuronal hyperexcitation as spreading depolarization (184, 185) or seizures [both in experimental (186–190) and clinical (191–193) settings]. Since K+ surplus in extracellular fluid space is linked to the function of cerebral micro perfusion, including neurovascular coupling (194–197), it may be hypothesized, that disturbed AQP4 function underlies the pathophysiology of several conditions related to improper reactivity of small vessels, including migraine and cluster headache (198, 199) via this mechanism. More importantly, the disturbance in potassium homeostasis attributable to AQP4 misfunction seems to result in ischemic exacerbation of secondary brain damage as may be noticed in stroke (200–202), subarachnoid hemorrhage (203–207), spontaneous intracerebral hemorrhage (208, 209) or traumatic brain injury (210–213). With regard to these conditions, even stronger links between secondary injury and AQP4 function do exist, namely the development of brain edema, which is the most direct result of impaired cerebral fluid homeostasis.
5. Brain Edema and Role of AQP4 in Its Pathophysiology
Certainly, the role of AQP4 in the development and subsiding of brain edema in different cerebral pathologies is of paramount importance for our understanding of the (patho-) physiology of cerebral fluid circulation. According to the canonical concept, forged by Klatzo and his research group, there are two main forms of cerebral edema existing. Vasogenic edema is characterized by extracellular water accumulation due to BBB dysfunction and increased transcytosis of plasma elements, including water (145). In turn, in cytotoxic edema water excess is gathered inside the cells (both neurons and astrocytes), manifested by beading i.e., swelling of astrocytic cells and neuronal dendrites (214–218). This dichotomy has, later on, been refined by numerous works by Marmarou and associates, describing in detail energetic depletion as the major drive for cytotoxic edema development as well as radiological manifestation of both edema types (219–224). In more recent works, a third kind of brain swelling, namely ionic edema, is distinguished. This type of edema is characterized by an early influx of both water and sodium ions from the perivascular compartment into the brain parenchyma, predominantly into the astrocytic cells. Ionic edema usually precedes the impairment of tight junctions being the first phase of ischemia-related edema formation (217, 225) and is associated with brain swelling of cytotoxic character (145, 217). Until the appearance of AQP4 on the stage, the main role in the molecular performance of both ionic and vasogenic edema remained vacant. Upon discovery and description of AQP4 function in water transportation (both in physiological and pathological conditions), our view on extracellular space and, more recently, the glymphatic system for development of brain edema has evolved dramatically (226).
Initially, the results of the experiments both in vivo and in vitro seemed to be inconclusive, since AQP4 and its expression demonstrated both surge and depletion of its activity due to developing brain edema. Thus, Ke et al. reported a reduction of AQP4 expression in areas of the traumatically swollen brain (227) and a similar observation has been made by Kiening et al. (228) and Bixt et al. in a rat model of posttraumatic edema (229). On the other hand, Fukuda et al. reported a delayed but significant raise in AQP4 level, following the development of posttraumatic brain edema (230) in juvenile rats, and similar observation has been made in adult animals by Taya et al. (231) and Zhang et al. (232). These observations were hard to reconcile until AQP4 knockout animals were available. Here, consequent analysis of different forms of edema in diverse experimental paradigms revealed that in the models with predominating cytotoxic edema demonstrable in transient or persistent ischemia models, lack of AQP4 function resulted in reduced water accumulation (233–235) and/or improved outcome (236–238). One possible explanation of these findings is, that in the absence of AQP4 channels, water excess, that would be accumulated in the swelling astrocytes due to compensatory mechanism after energetic depletion, remains in extracellular space and is managed by the glymphatic system and transported by perivascular spaces, being less effective (141) although more abundant in AQP4 knockouts (233). In conditions of vasogenic edema, the AQP4 channels seem to play a beneficial role, helping in the transportation of the fluid excess from the interstitial space to the glymphatic system. This hypothesis is sound with the observation, that in animal models of predominantly vasogenic edema, as in hemorrhagic stroke (209, 239–241) brain infection (136, 242–245) or brain tumor/cold lesion model (136, 246) brain edema subsides more efficiently in the presence of properly functioning AQP4. Importantly, not only the crude amount of AQP4 units defines its impact on brain edema or spinal cord edema development. AQP4-related permeability of astrocytic membranes is strongly dependent from subcellular localization of AQP4 water channels (112, 114). Pivotal study of Kitchen et al. demonstrated, that relocation of AQP4 units is modulated mainly by calmodulin (CaM), binding directly with AQP4 domains, while this action is further enhanced by AQP4 phosphorylation, performed by protein kinase A (PKA) (15). Thus, subcellular localization of AQP4 particles seems to be even more important for brain edema formation than expression of AQP4 genes.
The topic of AQP4 dual impact on brain edema development/resolution is the most clearly seen in neurotrauma research. Here, several traumatic brain injury (TBI) models exist, in which the dominance of cytotoxic or vasogenic edema type relies not only on the mechanism of primary injury but changes dynamically over time as the influence of AQP4 does. Several studies implementing controlled cortical impact paradigm (CCI) (227, 229, 247) (with an initial predominance of cytotoxic edema) demonstrated a decrease in AQP4 activity and expression accompanying edema development (227–229) [although Taya et al. (231) and Fukuda et al. (248) described an AQP4 concentration raise in early stages of CCI]. To the contrary, animal studies using fluid percussion injury (with predominantly vasogenic edema) (249, 250) or weight drop models (148) demonstrated a rise of AQP activity/expression. Notably, in models of more severe brain damage, the molecular effect of AQP4 activation may be counteracted by loss of the cells being AQP4 carriers, possibly making the interpretation of data even more difficult (251). The same refers to the models with mixed type of posttraumatic edema (232), demonstrable in several head injury studies conducted in AQP4 knockout animals, where the net differences in edema development were not as clear as in experiments, in which conditions of purely cytotoxic or purely vasogenic edema were analyzed (13, 252). Nevertheless, in long-term outcome analysis, it was documented that animals lacking AQP4 demonstrated better recovery regarding neuroinflammatory events and cognitive function (18). On the other hand, AQP4 deficiency was also associated with the lower threshold of posttraumatic seizures (188). Notably, in the animal model of minor head injury, where brain edema is of lesser relevance for the posttraumatic course, lack of AQP4 was demonstrated to be neuroprotective (253) (an effect similar to pathophysiological conditions with cerebral edema of cytotoxic type) (13). As was discussed above, previous studies have shown that AQP4 seems to have different functions and outcomes in different CNS disorders. Hence, the need for accurate and reproducible methods evaluating the activity of AQP4 should be underlined. These needs meet the recently developed calcein fluorescence assay. Shortly, calcein is a dye with fluorescent properties that is provided to plate adherent cells as the membrane-permeable and non-fluorescent acetoxymethyl ester (calcein-AM). Next, the calcein-AM is metabolized by intracellular enzymes to fluorescent calcein. Then, cell shrinkage is induced by using a hypertonic medium and the quenching fluorescence of calcein is continuously measured. The concentration-dependent fluorescence reflects cells volume and enables the evaluation of water transport across the plasma membrane. Obtained curves of the shrinkage of the cell allow quantifying relative and absolute water permeability (254). Of note, calcein fluorescent assay is only one of several ex vivo methods to assess AQP4 function. Here, the spectrum of methodology reaches from cell culture-based osmotic swelling tests over stopped-flow spectroscopy tests in e.g., liposome suspensions up to in silico computational assays. This variety of research methods should be critically considered, since every single assay carries its advantages and limitations, as outlined in exhaustive reviews of Verkman et al. (255) and Abi-Awan et al. (256).
6. Discussion: AQP4 as a Target for Therapeutical Approaches
Due to the ambiguous properties of AQP4 regarding its impact on water homeostasis in different types of edema, the results of experimental studies in which AQP4 function is blocked or enhanced need to be critically analyzed before being translated into clinical practice. Indeed, recently several compounds have been claimed to execute beneficial impact on the course of secondary brain damage, including brain edema via interference with AQP4 function and expression. Here, neuroprotective and antiedematous action of erythropoietin has been linked with the preservation of AQP4 function in trauma (257), hydrocephalus (258), and cerebral ischemia (259). Further, the neuroprotective action of several (food) antioxidants has been explained by the adjustment of AQP4 channel functions (260–264). Notably, the antiedematous effect of well-known osmolar drugs such as hypertonic saline and mannitol has been recently linked to modulation of AQP4-water channel permeability (265, 266). Finally, the idea of repurposing some of these well-known drugs like acetazolamide (267–269) or levetiracetam (270) was based on their presumed or proven effect on AQP4 channels. Even more promising is the therapeutic strategy, in which the AQP4 subcellular relocation as the main driver promoting brain or spinal cord edema is targeted. Here, the pharmacological inhibition of PKA and CaM as main regulators for AQP4 subcellular localization was efficient against spinal cord edema formation, breakdown of blood-spinal cord barrier, and improved functional outcome in a rat model of spinal cord injury (15). Since CaM inhibition was provided by trifluoperazine (TFP), a compound that is already approved as an antipsychotic drug, the perspective of swift clinical implementation of these experimental results emerges. Significantly, TFP has proven its neuroprotective and antiodematous effect also in experimental models of brain ischemia (271, 272). In the most recent study, implementing photothrombic stroke model, TFP has downregulated AQP4 expression, reduced the amount of brain edema, and improved the metabolic function (as demonstrated via increased glycogen level of astrocytes located in ischemic penumbra) (271).
Certainly, analyses of Kitchen et al. (15) and Sylvain et al. (271) clearly document the relationship between AQP4, its subcellular location, and the beneficial role of interfering AQP4 relocalization after an injury as the main mechanism for beneficial action of TFP. Nevertheless, for most of the other studies, the question emerges: are the antiedematous or neuroprotective properties truly mediated via impact on AQP4 activity, or is the shift in AQP4 expression/function only secondary and thus reflects rather an adapting reaction of the whole glymphatic system to the beneficial action of the given drug? This question should not hinder the research community in further search for treatment strategies, in which the pivotal position of AQP4 in cerebral edema management is utilized for the improvement of outcome and neuronal protection. A good example here is the use of decompressive craniectomy. This rapid change in physical properties of the skull and brain, including hydrostatic pressure change has been associated with increased AQP4 activity, at least in areas not affected by the abundant loss of neural and glial cells (250, 251). It is imaginable, that adding AQP4-targeted therapy [like acetazolamide (267, 273) or selective AQP4 channel blocker as TGN-020, being one of the most promising candidate drugs (274–277)] to the surgical decompression would allow reducing the risk of edema surplus, related with loss of hydrostatic resistance in the decompressed brain (267). Importantly, the list of structurally non-related compounds displaying the AQP4-inhibitory properties is long and includes ethoxzolamide, topiramate, lamotrigine, zonisamide, acetylsulfanilamide, phenytoin, bumetanide, furosemide, tetraethylammonium, and IMD0354 (273, 274, 278, 279). Obviously, this list encompasses several drugs that, similar to acetazolamide, have been already approved or tested for uses other than counteracting brain edema. Hence, the strategy of drug repurposing will open a fast track for the search for efficient AQP4-targeted treatment of brain edema. The importance of this approach is underlined by the fact, that despite several assays of AQP4 water transport function are available and has been abundantly used in basic research studies [for exhaustive review see (255, 256)], no single drug exists, that has yet been approved to successfully target AQP4 water channel function in a clinical setting (256). One of the possible obstacles is the toxicity and reduced selectivity of the compounds (including heavy metal derivates), which attempted to be used according to the traditional pore-blocking approach. It is difficult to circumvent this problem, even if modern pharmacodynamic forms of drug administration (e.g., liposome-encapsulated compounds) are used (256). Unfortunately, the strategy of virtually screening myriads of candidate inhibitors does not solve this problem but rather multiplies the number of putative AQP4 blockers that fail to exert their function in vivo. The possible reason here is the characteristic of AQP4 molecule, with the relatively small diameter of its pore and simple structure of its molecule, that, contrary to regular membrane receptors, lacks any complex intrinsic gating and transport mechanism (255). This makes AQP4 channels less prone to be targeted by the small inhibitory molecules, dramatically shortening the list of candidate drugs (255, 256). For this reason, the use of AQP4 targeted immunotherapy or AQP4-gene targeted treatment should be considered. Here, in the specific condition of NMO, the anti-AQP4 monoclonal antibody (aquaporumab), competitively binding to AQP4 has proven its efficacy in reducing lesions, at least in preclinical tests (280–283). It is noteworthy to consider an antibody-based approach in conditions where AQP4 function (as cytotoxic edema, ocular neovascularization, and astroglia proliferation including glial scarring and infiltration of glial tumors) is related to exacerbation or propagation of pathologic conditions. Limiting AQP4 expression by use of small interfering RNAs (siRNA) to suppress the translation process is another viable option (256), efficiently reducing the development of posttraumatic brain edema, at least in animal models (284, 285). Finally, the implementation of physical methods interfering with AQP4 function should be mentioned. For instance, global or focal brain hypothermia seems to exert their beneficial action not only by increasing AQP4 expression (286) but also partially via impacting the function of AQP4 channels (251, 287–289). Focusing on this aspect and enhancing the impact of cerebral hypothermia treatment with AQP4-active drugs would potentially allow the second renaissance of the latter treatment mode (currently abandoned due to clinical burden of side effects, including ionic disbalance) (290, 291). The key points of cellular AQP4 trafficking that are relevant for developing new treatment strategies are outlined in Figure 1.
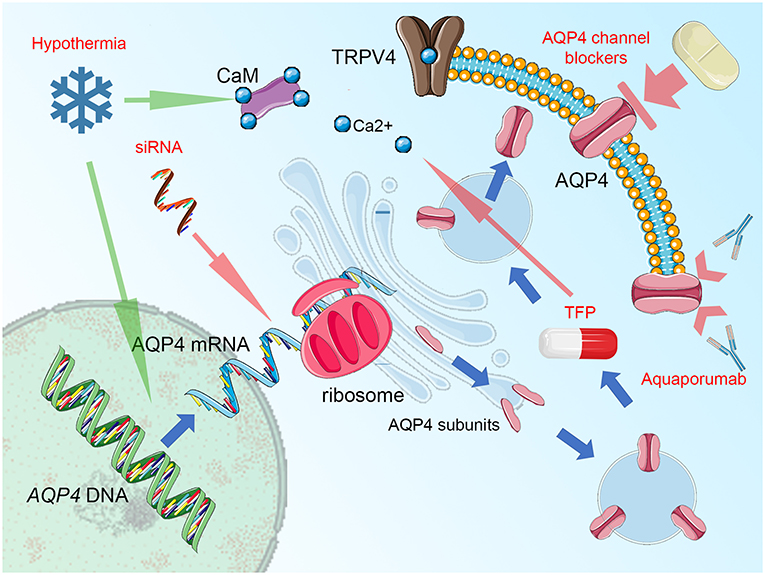
Figure 1. Summary figure, demonstrating aquaporin-4 (AQP4) cellular trafficking as a possible target for treatment. Blue arrows represent the process of AQP4 production and relocation, the groups of potential therapeutics are labeled by red text and their impact is marked by green (enhancing) or red arrows (blocking activity). AQP4 expression (transcription of the AQP4 gene and translation of AQP4 mRNA with ribosomal production of AQP single subunits may be disturbed by small interfering RNA (siRNA), attaching selectively to AQP4 mRNA domains and preventing the translational readout. The single subunits of AQP4 are organized into orthogonal arrays of particles (OAPs) and as tetramers are transferred by endosomal vesicles to the proximity of cell membrane (predominantly in astrocytic endfoot area). Here, the AQP4 translocation to the cell surface takes place. This process relies on the activity of vanilloid-receptor-related subfamily 4 calcium channel (TRPV4) and calmodulin (CaM), directly binding to the AQP4 particles. Importantly, blocking CaM activity by trifluoperazine (TFP) was efficient against AQP4 relocation and the formation of cytotoxic brain edema. Notably, hypothermia exerts opposite action enhancing AQP4 surface exposition and this effect may be counteracted by TRPV4 inhibitors, Ca2+ chelating compounds, or CaM blockers. This effect is more relevant than the impact of hypothermia on AQP4 expression, with increased transcription reported by some, but not all relevant studies. The AQP4 channel, while integrated into astrocytic surface membrane, may be simply blocked by a number of compounds, including acetazolamide, topiramate, lamotrigine, zonisamide, acetylsulfanilamide, phenytoin, bumetanide, furosemide, tetraethylammonium, and IMD0354 as well as by heavy metal derivates or—more selectively—by TGN-020. In conditions of autoimmune response that is driven against AQP4 channels, as seen in neuromyelitis optica (NMO), blocking of antigen epitopes by monoclonal antibodies (aquaporumab), has been demonstrated as an effective NMO treatment, at least in experimental conditions. Figure created with the use of Servier Medical Art images/content of smart.servier.com in compliance with the terms of the Creative Commons Attribution 3.0 Unported Licence.
7. Conclusion
There is growing interest in the structure and function of cerebral extracellular spaces described recently as the glymphatic system. Certainly, the glymphatic flow as well as water metabolismis dependent on numerous physical laws and molecular factors. However, evidence from recent years, regarding the role of cellular water channels in physiological conditions and diverse brain pathologies clearly point out AQP4 as the key component of cerebral fluid homeostasis, acting not only as a passive channel for water and small molecular substances but playing a key role in the proper functioning of blood-brain barrier and perivascular unit. Hereby adapting the glymphatic flow to the phases of neuronal activity with increased blood flow demand in an alternating manner. The knowledge about the role of AQP4 in cerebral fluid homeostasis is vast and continually growing, however, there is still a lot to discover in this field. For this reason, as well as the ambiguity of the impact of AQP4 on the neurological outcome of cerebral edema, attempts to translate somehow the positive results of in vivo studies into clinical practice should await more precise and more critical benefit-risk calculations for an inhomogeneous group of conditions, in which brain edema and/or neurovascular uncoupling play a major role.
Author Contributions
JS, MK, AW, and JO contributed the conception and design of the review. JS, MK, and AW wrote sections of the manuscript. All authors contributed to manuscript revision, read, and approved the submitted version.
Conflict of Interest
JO is a consultant to the Karl Storz Company and receives grants from the Erbe Company. These companies were not involved in the design and workflow during the preparation of the present review.
The remaining authors declare that the research was conducted in the absence of any commercial or financial relationships that could be construed as a potential conflict of interest.
Publisher's Note
All claims expressed in this article are solely those of the authors and do not necessarily represent those of their affiliated organizations, or those of the publisher, the editors and the reviewers. Any product that may be evaluated in this article, or claim that may be made by its manufacturer, is not guaranteed or endorsed by the publisher.
Acknowledgments
We thank Claude Lionel Ngassam Djeumen MD (Department of Neurosurgery Saarland University) for his comments on the manuscript and its linguistic corrections.
Abbreviations
AM, acetoxymethyl ester; APRE, acute phase response elements; AQP4, aquaporin-4; AQPs, aquaporins; ar/R, aromatic/arginine; BBB, blood-brain barrier; CaM, calmodulin; CBF, cerebral blood flow; CNS, central nervous system; CCI, controlled cortical impact; cGMP, cyclic guanosine monophosphate; cRNA, complementary ribonucleic acid; CSF, cerebrospinal fluid; Kir4.1, inwardly rectifying potassium channel 4.1; MAPK, mitogen-activated protein kinase; mRNA, messenger ribonucleic acid; NMO, neuromyelitis optica; NO, nitric oxide; NPA, asparagine–proline–arginine (motif); NPY, neuropeptide Y; nsSNPs, non-synonymous single nucleotide polymorphisms; NVU, neurovascular unit; OAPs, orthogonal arrays of particles; PKA, protein kinase A; siRNA, small interfering ribonucleic acid; Snta-1, syntrophin-1-alpha; TBI, traumatic brain injury; TFP, trifluoperazine; TRPV4, transient receptor potential cation channel subfamily V member 4; VIP, vasoactive intestinal peptide; VRS, Virchow-Robin space(s).
References
1. Schrier RW, Chen YC, Cadnapaphornchai MA. From finch to fish to man: role of aquaporins in body fluid and brain water regulation. Neuroscience. (2004) 129:897–904. doi: 10.1016/j.neuroscience.2004.06.043
2. Rasmussen MK, Mestre H, Nedergaard M. Fluid transport in the brain. Physiol Rev. (2021). doi: 10.1152/physrev.00031.2020 [Epub ahead of print].
3. Olsen ML, Khakh BS, Skatchkov SN, Zhou M, Lee CJ, Rouach N. New insights on astrocyte ion channels: critical for homeostasis and neuron-glia signaling. J Neurosci. (2015) 35:13827–35. doi: 10.1523/JNEUROSCI.2603-15.2015
4. Verkhratsky A, Nedergaard M. The homeostatic astroglia emerges from evolutionary specialization of neural cells. Philos Trans R Soc Lond B Biol Sci. (2016) 371:20150428. doi: 10.1098/rstb.2015.0428
5. Verkhratsky A, Nedergaard M, Hertz L. Why are astrocytes important? Neurochem Res. (2015) 40:389–401. doi: 10.1007/s11064-014-1403-2
6. Verkhratsky A, Nedergaard M. Astroglial cradle in the life of the synapse. Philos Trans R Soc Lond B Biol Sci. (2014) 369:20130595. doi: 10.1098/rstb.2013.0595
7. Natale G, Limanaqi F, Busceti CL, Mastroiacovo F, Nicoletti F, Puglisi-Allegra S, et al. Glymphatic system as a gateway to connect neurodegeneration from periphery to CNS. Front Neurosci. (2021) 15:639140. doi: 10.3389/fnins.2021.639140
8. Mestre H, Mori Y, Nedergaard M. The brain's glymphatic system: current controversies. Trends Neurosci. (2020) 43:458–466. doi: 10.1016/j.tins.2020.04.003
9. Jessen NA, Munk AS, Lundgaard I, Nedergaard M. The glymphatic system: a beginner's guide. Neurochem Res. (2015) 40:2583–99. doi: 10.1007/s11064-015-1581-6
10. Zhou X, Li Y, Lenahan C, Ou Y, Wang M, He Y. Glymphatic system in the central nervous system, a novel therapeutic direction against brain edema after stroke. Front Aging Neurosci. (2021) 13:698036. doi: 10.3389/fnagi.2021.698036
11. Xu M, Xiao M, Li S, Yang B. Aquaporins in nervous system. Adv Exp Med Biol. (2017) 969:81–103. doi: 10.1007/978-94-024-1057-0_5
12. Nakada T, Virchow-Robin Virchow-Robin space and aquaporin-4: new insights on an old friend. Croat Med J. (2014) 55:328–36. doi: 10.3325/cmj.2014.55.328
13. Verkman AS, Binder DK, Bloch O, Auguste K, Papadopoulos MC. Three distinct roles of aquaporin-4 in brain function revealed by knockout mice. Biochim Biophys Acta. (2006) 1758:1085–93. doi: 10.1016/j.bbamem.2006.02.018
14. Manley GT, Binder DK, Papadopoulos MC, Verkman AS. New insights into water transport and edema in the central nervous system from phenotype analysis of aquaporin-4 null mice. Neuroscience. (2004) 129:983–1. doi: 10.1016/j.neuroscience.2004.06.088
15. Kitchen P, Salman MM, Halsey AM, Clarke-Bland C, MacDonald JA, Ishida H, et al. Targeting aquaporin-4 subcellular localization to treat central nervous system edema. Cell. (2020) 181:784–99.e19. doi: 10.1016/j.cell.2020.03.037
16. Amorini AM, Dunbar JG, Marmarou A. Modulation of aquaporin-4 water transport in a model of TBI. Acta Neurochir Suppl. (2003) 86:261–3. doi: 10.1007/978-3-7091-0651-8_56
17. Xiong A, Xiong R, Yu J, Liu Y, Liu K, Jin G, et al. Aquaporin-4 is a potential drug target for traumatic brain injury via aggravating the severity of brain edema. Burns Trauma. (2021) 9:tkaa050. doi: 10.1093/burnst/tkaa050
18. Liu X, Xie Y, Wan X, Wu J, Fan Z, Yang L. Protective effects of aquaporin-4 deficiency on longer-term neurological outcomes in a mouse model. Neurochem Res. (2021) 46:1380–9. doi: 10.1007/s11064-021-03272-7
19. Gardner WJ. The brain's third circulation. Arch Neurol. (1977) 34:200. doi: 10.1001/archneur.1977.00500150086021
20. Proulx ST Cerebrospinal Cerebrospinal fluid outflow: a review of the historical and contemporary evidence for arachnoid villi perineural routes dural lymphatics. Cell Mol Life Sci. (2021) 78:2429–57. doi: 10.1007/s00018-020-03706-5
21. Kumar A, Ghosh SK, Faiq MA, Deshmukh VR, Kumari C, Pareek V. A brief review of recent discoveries in human anatomy. QJM. (2019) 112:567–73. doi: 10.1093/qjmed/hcy241
22. Rasmussen MK, Mestre H, Nedergaard M. The glymphatic pathway in neurological disorders. Lancet Neurol. (2018) 17:1016–24. doi: 10.1016/S1474-4422(18)30318-1
23. Nakada T, Kwee IL. Fluid dynamics inside the brain barrier: current concept of interstitial flow, glymphatic flow, and cerebrospinal fluid circulation in the brain. Neuroscientist. (2019) 25:155–66. doi: 10.1177/1073858418775027
24. Zhang Y, Song J, He XZ, Xiong J, Xue R, Ge JH, et al. Quantitative determination of glymphatic flow using spectrophotofluorometry. Neurosci Bull. (2020) 36:1524–37. doi: 10.1007/s12264-020-00548-w
25. Ramos M, Burdon Bechet N, Battistella R, Pavan C, Xavier ALR, Nedergaard M, et al. Cisterna magna injection in rats to study glymphatic function. Methods Mol Biol. (2019) 1938:97–104. doi: 10.1007/978-1-4939-9068-9_7
26. Albargothy NJ, Johnston DA, MacGregor-Sharp M, Weller RO, Verma A, Hawkes CA, et al. Convective influx/glymphatic system: tracers injected into the CSF enter and leave the brain along separate periarterial basement membrane pathways. Acta Neuropathol. (2018) 136:139–52. doi: 10.1007/s00401-018-1862-7
27. Zhang C, Lin J, Wei F, Song J, Chen W, Shan L, et al. Characterizing the glymphatic influx by utilizing intracisternal infusion of fluorescently conjugated cadaverine. Life Sci. (2018) 201:150–60. doi: 10.1016/j.lfs.2018.03.057
28. Yang L, Kress BT, Weber HJ, Thiyagarajan M, Wang B, Deane R, et al. Evaluating glymphatic pathway function utilizing clinically relevant intrathecal infusion of CSF tracer. J Transl Med. (2013) 11:107. doi: 10.1186/1479-5876-11-107
29. Taoka T, Naganawa S. Glymphatic imaging using MRI. J Magn Reson Imaging. (2020) 51:11–24. doi: 10.1002/jmri.26892
30. Benveniste H, Lee H, Ozturk B, Chen X, Koundal S, Vaska P, et al. Glymphatic cerebrospinal fluid and solute transport quantified by MRI and PET imaging. Neuroscience. (2020) 474:63–79. doi: 10.1016/j.neuroscience.2020.11.014
31. Bèchet NB, Shanbhag NC, Lundgaard I. Glymphatic pathways in the gyrencephalic brain. J Cereb Blood Flow Metab. (2021) 41:2264–79. doi: 10.1177/0271678X21996175
32. Raghunandan A, Ladron-de-Guevara A, Tithof J, Mestre H, Du T, Nedergaard M, et al. Bulk flow of cerebrospinal fluid observed in periarterial spaces is not an artifact of injection. eLife. (2021) 10:65958. doi: 10.7554/eLife.65958
33. Gutierrez J, DiTullio M, YK KC, Alperin N, Bagci A, R LS, et al. Brain arterial dilatation modifies the association between extracranial pulsatile hemodynamics and brain perivascular spaces: the Northern Manhattan Study. Hypertens Res. (2019) 42:1019–28. doi: 10.1038/s41440-019-0255-1
34. Mestre H, Tithof J, Du T, Song W, Peng W, Sweeney AM, et al. Flow of cerebrospinal fluid is driven by arterial pulsations and is reduced in hypertension. Nat Commun. (2018) 9:4878. doi: 10.1038/s41467-018-07318-3
35. Iliff JJ, Wang M, Zeppenfeld DM, Venkataraman A, Plog BA, Liao Y, et al. Cerebral arterial pulsation drives paravascular CSF-interstitial fluid exchange in the murine brain. J Neurosci. (2013) 33:18190–9. doi: 10.1523/JNEUROSCI.1592-13.2013
36. Vinje V, Bakker E, Rognes ME. Brain solute transport is more rapid in periarterial than perivenous spaces. Sci Rep. (2021) 11:16085. doi: 10.1038/s41598-021-95306-x
37. Cheng Y, Liu X, Ma X, Garcia R, Belfield K, Haorah J. Alcohol promotes waste clearance in the CNS via brain vascular reactivity. Free Radic Biol Med. (2019) 143:115–26. doi: 10.1016/j.freeradbiomed.2019.07.029
38. Venkat P, Chopp M, Chen J. New insights into coupling and uncoupling of cerebral blood flow and metabolism in the brain. Croat Med J. (2016) 57:223–8. doi: 10.3325/cmj.2016.57.223
39. Han F, Chen J, Belkin-Rosen A, Gu Y, Luo L, Buxton OM, et al. Reduced coupling between cerebrospinal fluid flow and global brain activity is linked to Alzheimer disease-related pathology. PLoS Biol. (2021) 19:e3001233. doi: 10.1371/journal.pbio.3001233
40. Han F, Brown GL, Zhu Y, Belkin-Rosen AE, Lewis MM, Du G, et al. Decoupling of global brain activity and cerebrospinal fluid flow in Parkinson's Disease cognitive decline. Mov Disord. (2021) doi: 10.1101/2021.01.08.425953
41. Ramirez J, Holmes MF, Berezuk C, Kwan D, Tan B, Beaton D, et al. MRI-visible perivascular space volumes, sleep duration and daytime dysfunction in adults with cerebrovascular disease. Sleep Med. (2021) 83:83–88. doi: 10.1016/j.sleep.2021.03.043
42. Manouchehrian O, Ramos M, Bachiller S, Lundgaard I, Deierborg T. Acute systemic LPS-exposure impairs perivascular CSF distribution in mice. J Neuroinflammation. (2021) 18:34. doi: 10.1186/s12974-021-02082-6
43. Mortensen KN, Sanggaard S, Mestre H, Lee H, Kostrikov S, Xavier ALR, et al. Impaired glymphatic transport in spontaneously hypertensive rats. J Neurosci. (2019) 39:6365–77. doi: 10.1523/JNEUROSCI.1974-18.2019
44. Chen W, Huang P, Zeng H, Lin J, Shi Z, Yao X. Cocaine-induced structural and functional impairments of the glymphatic pathway in mice. Brain Behav Immun. (2020) 88:97–104. doi: 10.1016/j.bbi.2020.04.057
45. Blair GW, Thrippleton MJ, Shi Y, Hamilton I, Stringer M, Chappell F, et al. Intracranial hemodynamic relationships in patients with cerebral small vessel disease. Neurology. (2020) 94:e2258–69. doi: 10.1212/WNL.0000000000009483
46. Hudetz AG, Regulation Regulation of oxygen supply in the cerebral circulation. Adv Exp Med Biol. (1997) 428:513–20. doi: 10.1007/978-1-4615-5399-1_73
47. McCarron RM, Chen Y, Tomori T, Strasser A, Mechoulam R, Shohami E, et al. Endothelial-mediated regulation of cerebral microcirculation. J Physiol Pharmacol. (2006) 57(Suppl. 11):133–44. doi: 10.26402/jpp
48. Duchemin S, Boily M, Sadekova N, Girouard H. The complex contribution of NOS interneurons in the physiology of cerebrovascular regulation. Front Neural Circuits. (2012) 6:51. doi: 10.3389/fncir.2012.00051
49. McConnell HL, Kersch CN, Woltjer RL, Neuwelt EA. The translational significance of the neurovascular unit. J Biol Chem. (2017) 292:762–70. doi: 10.1074/jbc.R116.760215
50. Quelhas P, Baltazar G, Cairrao E. The neurovascular unit: focus on the regulation of arterial smooth muscle cells. Curr Neurovasc Res. (2019) 16:502–15. doi: 10.2174/1567202616666191026122642
51. Zheng Z, Chopp M, Chen J. Multifaceted roles of pericytes in central nervous system homeostasis and disease. J Cereb Blood Flow Metab. (2020) 40:1381–401. doi: 10.1177/0271678X20911331
52. Gotoh J, Kuang TY, Nakao Y, Cohen DM, Melzer P, Itoh Y, et al. Regional differences in mechanisms of cerebral circulatory response to neuronal activation. Am J Physiol Heart Circ Physiol. (2001) 280:H821–9. doi: 10.1152/ajpheart.2001.280.2.H821
53. Dormanns K, Brown RG, David T. The role of nitric oxide in neurovascular coupling. J Theor Biol. (2016) 394:1–17. doi: 10.1016/j.jtbi.2016.01.009
54. Niwa K, Araki E, Morham SG, Ross ME, Iadecola C. Cyclooxygenase-2 contributes to functional hyperemia in whisker-barrel cortex. J Neurosci. (2000) 20:763–70. doi: 10.1523/JNEUROSCI.20-02-00763.2000
55. Lacroix A, Toussay X, Anenberg E, Lecrux C, Ferreirós N, Karagiannis A, et al. COX-2-derived prostaglandin E2 produced by pyramidal neurons contributes to neurovascular coupling in the rodent cerebral cortex. J Neurosci. (2015) 35:11791–810. doi: 10.1523/JNEUROSCI.0651-15.2015
56. Stobart JL, Lu L, Anderson HD, Mori H, Anderson CM. Astrocyte-induced cortical vasodilation is mediated by D-serine and endothelial nitric oxide synthase. Proc Natl Acad Sci USA. (2013) 110:3149–54. doi: 10.1073/pnas.1215929110
57. Du W, Stern JE, Filosa JA. Neuronal-derived nitric oxide and somatodendritically released vasopressin regulate neurovascular coupling in the rat hypothalamic supraoptic nucleus. J Neurosci. (2015) 35:5330–41. doi: 10.1523/JNEUROSCI.3674-14.2015
58. Cauli B, Tong X-K, Rancillac A, Serluca N, Lambolez B, Rossier J, et al. Cortical GABA interneurons in neurovascular coupling: relays for subcortical vasoactive pathways. J Neurosci. (2004) 24:8940–49. doi: 10.1523/JNEUROSCI.3065-04.2004
59. Perrenoud Q, Rossier J, Férézou I, Geoffroy H, Gallopin T, Vitalis T, et al. Activation of cortical 5-HT(3) receptor-expressing interneurons induces NO mediated vasodilatations and NPY mediated vasoconstrictions. Front Neural Circuits. (2012) 6:50. doi: 10.3389/fncir.2012.00050
60. Abounader R, Villemure JG, Hamel E. Characterization of neuropeptide Y (NPY) receptors in human cerebral arteries with selective agonists and the new Y1 antagonist BIBP 3226. Br J Pharmacol. (1995) 116:2245–50. doi: 10.1111/j.1476-5381.1995.tb15060.x
61. Yaksh TL, Wang JY, Go VL. Cortical vasodilatation produced by vasoactive intestinal polypeptide (VIP) and by physiological stimuli in the cat. J Cereb Blood Flow Metab. (1987) 7:315–26. doi: 10.1038/jcbfm.1987.69
62. Nakada T, Kwee IL, Igarashi H, Suzuki Y. Aquaporin-4 functionality and virchow-robin space water dynamics: physiological model for neurovascular coupling and glymphatic flow. Int J Mol Sci. (2017) 18:1798. doi: 10.3390/ijms18081798
63. Martinac AD, Fletcher DF, Bilston LE. Phase offset between arterial pulsations and subarachnoid space pressure fluctuations are unlikely to drive periarterial cerebrospinal fluid flow. Biomech Model Mechanobiol. (2021) 10:10102. doi: 10.1007/s10237-021-01474-0
64. Kedarasetti RT, Drew PJ, Costanzo F. Arterial pulsations drive oscillatory flow of CSF but not directional pumping. Sci Rep. (2020) 10:10102. doi: 10.1038/s41598-020-66887-w
65. Ichimura T, Fraser PA, Cserr HF. Distribution of extracellular tracers in perivascular spaces of the rat brain. Brain Res. (1991) 545:103–13. doi: 10.1016/0006-8993(91)91275-6
66. Segarra M, Aburto MR, Acker-Palmer A. Blood-brain barrier dynamics to maintain brain homeostasis. Trends Neurosci. (2021) 44:393–405. doi: 10.1016/j.tins.2020.12.002
67. Hamasaki S, Mukuda T, Koyama Y, Nakane H, Kaidoh T. Constitutive accessibility of circulating proteins to hippocampal neurons in physiologically normal rats. Brain Behav. (2020) 10:e01544. doi: 10.1002/brb3.1544
68. Nordström CH, Koskinen LO, Olivecrona M. Aspects on the physiological and biochemical foundations of neurocritical care. Front Neurol. (2017) 8:274. doi: 10.3389/fneur.2017.00274
69. Dickie BR, Parker GJM, Parkes LM. Measuring water exchange across the blood-brain barrier using MRI. Prog Nucl Magn Reson Spectrosc. (2020) 116:19–39. doi: 10.1016/j.pnmrs.2019.09.002
70. Olson JE, Banks M, Dimlich RV, Evers J. Blood-brain barrier water permeability and brain osmolyte content during edema development. Acad Emerg Med. (1997) 4:662–73. doi: 10.1111/j.1553-2712.1997.tb03757.x
71. Rapoport SI, Matthews K, Thompson HK, Pettigrew KD. Osmotic opening of the blood-brain barrier in the rhesus monkey without measurable brain edema. Brain Res. (1977) 136:23–9. doi: 10.1016/0006-8993(77)90128-7
72. Harik SI Blood–brain barrier sodium/potassium pump: modulation by central noradrenergic innervation. Proc Natl Acad Sci USA. (1986) 83:4067–70. doi: 10.1073/pnas.83.11.4067
73. Komura J, Tamai I, Senmaru M, Terasaki T, Sai Y, Tsuji A. Sodium and chloride ion-dependent transport of beta-alanine across the blood-brain barrier. J Neurochem. (1996) 67:330–5. doi: 10.1046/j.1471-4159.1996.67010330.x
74. Zhang Y, Liu GQ. Sodium and chloride-dependent high and low-affinity uptakes of GABA by brain capillary endothelial cells. Brain Res. (1998) 808:1–7. doi: 10.1016/S0006-8993(98)00767-7
75. Zaragozá R, Transport Transport of amino acids across the blood-brain barrier. Front Physiol. (2020) 11:973. doi: 10.3389/fphys.2020.00973
76. Shao X, Ma SJ, Casey M, D'Orazio L, Ringman JM, Wang DJJ. Mapping water exchange across the blood-brain barrier using 3D diffusion-prepared arterial spin labeled perfusion MRI. Magn Reson Med. (2019) 81:3065–079. doi: 10.1002/mrm.27632
77. Shao X, Jann K, Ma SJ, Yan L, Montagne A, Ringman JM, et al. Comparison between blood-brain barrier water exchange rate and permeability to gadolinium-based contrast agent in an elderly cohort. Front Neurosci. (2020) 14:571480. doi: 10.3389/fnins.2020.571480
78. Chassidim Y, Veksler R, Lublinsky S, Pell GS, Friedman A, Shelef I. Quantitative imaging assessment of blood-brain barrier permeability in humans. Fluids Barriers CNS. (2013) 10:9. doi: 10.1186/2045-8118-10-9
79. Prager O, Chassidim Y, Klein C, Levi H, Shelef I, Friedman A. Dynamic in vivo imaging of cerebral blood flow and blood-brain barrier permeability. Neuroimage. (2010) 49:337–44. doi: 10.1016/j.neuroimage.2009.08.009
80. Davoodi-Bojd E, Ding G, Zhang L, Li Q, Li L, Chopp M, et al. Modeling glymphatic system of the brain using MRI. Neuroimage. (2019) 188:616–27. doi: 10.1016/j.neuroimage.2018.12.039
81. Ringstad G, Valnes LM, Dale AM, Pripp AH, Vatnehol SS, Emblem KE, et al. Brain-wide glymphatic enhancement and clearance in humans assessed with MRI. JCI Insight. (2018) 3:121537. doi: 10.1172/jci.insight.121537
82. Lee H, Mortensen K, Sanggaard S, Koch P, Brunner H, Quistorff B, et al. Quantitative Gd-DOTA uptake from cerebrospinal fluid into rat brain using 3D VFA-SPGR at 9.4T. Magn Reson Med. (2018) 79:1568–78. doi: 10.1002/mrm.26779
83. Zong X, Lian C, Jimenez J, Yamashita K, Shen D, Lin W. Morphology of perivascular spaces and enclosed blood vessels in young to middle-aged healthy adults at 7T: dependences on age, brain region, breathing gas. Neuroimage. (2020) 218:116978. doi: 10.1016/j.neuroimage.2020.116978
84. Osipova ED, Semyachkina-Glushkovskaya OV, Morgun AV, Pisareva NV, Malinovskaya NA, Boitsova EB, et al. Gliotransmitters and cytokines in the control of blood-brain barrier permeability. Rev Neurosci. (2018) 29:567–91. doi: 10.1515/revneuro-2017-0092
85. Azad AK, Raihan T, Ahmed J, Hakim A, Emon TH, Chowdhury PA. Human aquaporins: functional diversity and potential roles in infectious and non-infectious diseases. Front Genet. (2021) 12:654865. doi: 10.3389/fgene.2021.654865
86. King LS, Kozono D, Agre P. From structure to disease: the evolving tale of aquaporin biology. Nat Rev Mol Cell Biol. (2004) 5:687–98. doi: 10.1038/nrm1469
87. Hasegawa H, Ma T, Skach W, Matthay MA, Verkman AS. Molecular cloning of a mercurial-insensitive water channel expressed in selected water-transporting tissues. J Biol Chem. (1994) 269:5497–500. doi: 10.1016/S0021-9258(17)37486-0
88. Frigeri A, Gropper MA, Turck CW, Verkman AS. Immunolocalization of the mercurial-insensitive water channel and glycerol intrinsic protein in epithelial cell plasma membranes. Proc Natl Acad Sci USA. (1995) 92:4328–31. doi: 10.1073/pnas.92.10.4328
89. Frigeri A, Gropper MA, Umenishi F, Kawashima M, Brown D, Verkman AS. Localization of MIWC and GLIP water channel homologs in neuromuscular, epithelial and glandular tissues. J Cell Sci. (1995) 108(Pt 9):2993–3002. doi: 10.1242/jcs.108.9.2993
90. Nielsen S, Nagelhus EA, Amiry-Moghaddam M, Bourque C, Agre P, Ottersen OP. Specialized membrane domains for water transport in glial cells: high-resolution immunogold cytochemistry of aquaporin-4 in rat brain. J Neurosci. (1997) 17:171–80. doi: 10.1523/JNEUROSCI.17-01-00171.1997
91. Takumi Y, Nagelhus EA, Eidet J, Matsubara A, Usami S, Shinkawa H, et al. Select types of supporting cell in the inner ear express aquaporin-4 water channel protein. Eur J Neurosci. (1998) 10:3584–95. doi: 10.1046/j.1460-9568.1998.00360.x
92. Agre P, Preston GM, Smith BL, Jung JS, Raina S, Moon C, et al. Aquaporin CHIP: the archetypal molecular water channel. Am J Physiol. (1993) 265:F463–76. doi: 10.1152/ajprenal.1993.265.4.F463
93. Jung JS, Bhat RV, Preston GM, Guggino WB, Baraban JM, Agre P. Molecular characterization of an aquaporin cDNA from brain: candidate osmoreceptor and regulator of water balance. Proc Natl Acad Sci USA. (1994) 91:13052–6. doi: 10.1073/pnas.91.26.13052
94. Fujiyoshi Y, Mitsuoka K., de Groot BL, Philippsen A, Grubmüller H, Agre P, Engel A. Structure and function of water channels. Curr Opin Struct Biol. (2002) 12:509–15. doi: 10.1016/S0959-440X(02)00355-X
95. Murata K, Mitsuoka K, Hirai T, Walz T, Agre P, Heymann JB, et al. Structural determinants of water permeation through aquaporin-1. Nature. (2000) 407:599–605. doi: 10.1038/35036519
96. Agre P, Kozono D. Aquaporin water channels: molecular mechanisms for human diseases. FEBS Lett. (2003) 555:72–8. doi: 10.1016/S0014-5793(03)01083-4
97. Lu M, Lee MD, Smith BL, Jung JS, Agre P, Verdijk MA, et al. The human AQP4 gene: definition of the locus encoding two water channel polypeptides in brain. Proc Natl Acad Sci USA. (1996) 93:10908–12. doi: 10.1073/pnas.93.20.10908
98. Palazzo C, Buccoliero C, Mola MG, Abbrescia P, Nicchia GP, Trojano M, et al. AQP4ex is crucial for the anchoring of AQP4 at the astrocyte end-feet and for neuromyelitis optica antibody binding. Acta Neuropathol Commun. (2019) 7:51. doi: 10.1186/s40478-019-0707-5
99. De Bellis M., Pisani F, Mola MG, Basco D, Catalano F, Nicchia GP, et al. A novel human aquaporin-4 splice variant exhibits a dominant-negative activity: a new mechanism to regulate water permeability. Mol Biol Cell. (2014) 25:470–80. doi: 10.1091/mbc.e13-06-0331
100. Jorgačevski J, Zorec R, Potokar M. Insights into cell surface expression, supramolecular organization, and functions of aquaporin 4 isoforms in astrocytes. Cells. (2020) 9:2622. doi: 10.3390/cells9122622
101. Moe SE, Sorbo JG, Sogaard R, Zeuthen T, Petter Ottersen O, Holen T. New isoforms of rat Aquaporin-4. Genomics. (2008) 91:367–77. doi: 10.1016/j.ygeno.2007.12.003
102. De Bellis M., Pisani F, Mola MG, Rosito S, Simone L, Buccoliero C, et al. Translational readthrough generates new astrocyte AQP4 isoforms that modulate supramolecular clustering, glial endfeet localization, water transport. Glia. (2017) 65:790–803. doi: 10.1002/glia.23126
103. Lisjak M, Potokar M, Zorec R, Jorgačevski J. Indirect role of AQP4b and AQP4d isoforms in dynamics of astrocyte volume and orthogonal arrays of particles. Cells. (2020) 9:735. doi: 10.3390/cells9030735
104. Sorbo JG, Moe SE, Ottersen OP, Holen T. The molecular composition of square arrays. Biochemistry. (2008) 47:2631–7. doi: 10.1021/bi702146k
105. Rossi A, Moritz TJ, Ratelade J, Verkman AS. Super-resolution imaging of aquaporin-4 orthogonal arrays of particles in cell membranes. J Cell Sci. (2012) 125:4405–12. doi: 10.1242/jcs.109603
106. Jin BJ, Rossi A, Verkman AS. Model of aquaporin-4 supramolecular assembly in orthogonal arrays based on heterotetrameric association of M1-M23 isoforms. Biophys J. (2011) 100:2936–45. doi: 10.1016/j.bpj.2011.05.012
107. Yang B, Ma T, Verkman AS. cDNA cloning, gene organization, and chromosomal localization of a human mercurial insensitive water channel. Evidence for distinct transcriptional units. J Biol Chem. (1995) 270:22907–13. doi: 10.1074/jbc.270.39.22907
108. National Center for Biotechnology Information. Database of single nucleotide polymorphisms (dbsnp): homo sapiens. Nucleic Acids Res. (2021) 28:352–55. Retreived from: https://www.ncbi.nlm.nih.gov/snp/ (accessed August 20, 2021).
109. Sorani MD, Zador Z, Hurowitz E, Yan D, Giacomini KM, Manley GT. Novel variants in human Aquaporin-4 reduce cellular water permeability. Hum Mol Genet. (2008) 17:2379–89. doi: 10.1093/hmg/ddn138
110. Crane JM, Van Hoek AN, Skach WR, Verkman AS. Aquaporin-4 dynamics in orthogonal arrays in live cells visualized by quantum dot single particle tracking. Mol Biol Cell. (2008) 19:3369–78. doi: 10.1091/mbc.e08-03-0322
111. Furman CS, Gorelick-Feldman DA, Davidson KG, Yasumura T, Neely JD, Agre P, et al. Aquaporin-4 square array assembly: opposing actions of M1 and M23 isoforms. Proc Natl Acad Sci USA. (2003) 100:13609–14. doi: 10.1073/pnas.2235843100
112. Kitchen P, Day RE, Taylor LH, Salman MM, Bill RM, Conner MT, et al. Identification and molecular mechanisms of the rapid tonicity-induced relocalization of the aquaporin 4 channel. J Biol Chem. (2015) 290:16873–81. doi: 10.1074/jbc.M115.646034
113. Rossi A, Pisani F, Nicchia GP, Svelto M, Frigeri A. Evidences for a leaky scanning mechanism for the synthesis of the shorter M23 protein isoform of aquaporin-4: implication in orthogonal array formation and neuromyelitis optica antibody interaction. J Biol Chem. (2010) 285:4562–9. doi: 10.1074/jbc.M109.069245
114. Salman MM, Kitchen P, Woodroofe MN, Brown JE, Bill RM, Conner AC, et al. Hypothermia increases aquaporin 4 (AQP4) plasma membrane abundance in human primary cortical astrocytes via a calcium/transient receptor potential vanilloid 4 (TRPV4)- and calmodulin-mediated mechanism. Eur J Neurosci. (2017) 46:2542–7. doi: 10.1111/ejn.13723
115. Ciappelloni S, Bouchet D, Dubourdieu N, Boué-Grabot E, Kellermayer B, Manso C, et al. Aquaporin-4 surface trafficking regulates astrocytic process motility and synaptic activity in health and autoimmune disease. Cell Rep. (2019) 27:3860–72.e4. doi: 10.1016/j.celrep.2019.05.097
116. Amiry-Moghaddam M, Frydenlund DS, Ottersen OP. Anchoring of aquaporin-4 in brain: molecular mechanisms and implications for the physiology and pathophysiology of water transport. Neuroscience. (2004) 129:999–1010. doi: 10.1016/j.neuroscience.2004.08.049
117. Nagelhus EA, Veruki ML, Torp R, Haug FM, Laake JH, Nielsen S, et al. Aquaporin-4 water channel protein in the rat retina and optic nerve: polarized expression in Müller cells and fibrous astrocytes. J Neurosci. (1998) 18:2506–19. doi: 10.1523/JNEUROSCI.18-07-02506.1998
118. Neely JD, Amiry-Moghaddam M, Ottersen OP, Froehner SC, Agre P, Adams ME. Syntrophin-dependent expression and localization of Aquaporin-4 water channel protein. Proc Natl Acad Sci USA. (2001) 98:14108–13. doi: 10.1073/pnas.241508198
119. Amiry-Moghaddam M, Otsuka T, Hurn PD, Traystman RJ, Haug FM, Froehner SC, et al. An alpha-syntrophin-dependent pool of AQP4 in astroglial end-feet confers bidirectional water flow between blood and brain. Proc Natl Acad Sci USA. (2003) 100:2106–11. doi: 10.1073/pnas.0437946100
120. Pitonzo D, Skach WR. Molecular mechanisms of aquaporin biogenesis by the endoplasmic reticulum Sec61 translocon. Biochim Biophys Acta. (2006) 1758:976–88. doi: 10.1016/j.bbamem.2006.04.021
121. Nagelhus EA, Horio Y, Inanobe A, Fujita A, Haug FM, Nielsen S, et al. Immunogold evidence suggests that coupling of K+ siphoning and water transport in rat retinal Müller cells is mediated by a coenrichment of Kir4.1 and AQP4 in specific membrane domains. Glia. (1999) 26:47–54. doi: 10.1002/(SICI)1098-1136(199903)26:1<47::AID-GLIA5>3.0.CO;2-5
122. Fallier-Becker P, Sperveslage J, Wolburg H, Noell S. The impact of agrin on the formation of orthogonal arrays of particles in cultured astrocytes from wild-type and agrin-null mice. Brain Res. (2011) 1367:2–12. doi: 10.1016/j.brainres.2010.09.092
123. Huang J, Li J, Feng C, Huang X, Wong L, Liu X, et al. Blood-brain barrier damage as the starting point of leukoaraiosis caused by cerebral chronic hypoperfusion and its involved mechanisms: effect of agrin and aquaporin-4. Biomed Res Int. (2018) 2018:2321797. doi: 10.1155/2018/2321797
124. Bobik M, Ellisman MH, Rudy B, Martone ME. Potassium channel subunit Kv3.2 and the water channel aquaporin-4 are selectively localized to cerebellar pinceau. Brain Res. (2004) 1026:168–78. doi: 10.1016/j.brainres.2004.07.088
125. Ozawa Y, Toda E, Kawashima H, Homma K, Osada H, Nagai N, et al. Aquaporin 4 suppresses neural hyperactivity and synaptic fatigue and fine-tunes neurotransmission to regulate visual function in the mouse retina. Mol Neurobiol. (2019) 56:8124–35. doi: 10.1007/s12035-019-01661-2
126. Hara-Chikuma M, Verkman AS. Physiological roles of glycerol-transporting aquaporins: the aquaglyceroporins. Cell Mol Life Sci. (2006) 63:1386–92. doi: 10.1007/s00018-006-6028-4
127. Kitchen P, Salman MM, Pickel SU, Jennings J, Törnroth-Horsefield S, Conner MT, et al. Water channel pore size determines exclusion properties but not solute selectivity. Sci Rep. (2019) 9:20369. doi: 10.1038/s41598-019-56814-z
128. McCoy E, Sontheimer H. Expression and function of water channels (aquaporins) in migrating malignant astrocytes. Glia. (2007) 55:1034–43. doi: 10.1002/glia.20524
129. Smith AJ, Jin BJ, Ratelade J, Verkman AS. Aggregation state determines the localization and function of M1- and M23-aquaporin-4 in astrocytes. J Cell Biol. (2014) 204:559–73. doi: 10.1083/jcb.201308118
130. Nagelhus EA, Ottersen OP. Physiological roles of aquaporin-4 in brain. Physiol Rev. (2013) 93:1543–62. doi: 10.1152/physrev.00011.2013
131. Kitchen P, Day RE, Salman MM, Conner MT, Bill RM, Conner AC. Beyond water homeostasis: Diverse functional roles of mammalian aquaporins. Biochim Biophys Acta. (2015) 1850:2410–21. doi: 10.1016/j.bbagen.2015.08.023
132. Yao X, Hrabetová S, Nicholson C, Manley GT. Aquaporin-4-deficient mice have increased extracellular space without tortuosity change. J Neurosci. (2008) 28:5460–4. doi: 10.1523/JNEUROSCI.0257-08.2008
133. Teng Z, Wang A, Wang P, Wang R, Wang W, Han H. The effect of aquaporin-4 knockout on interstitial fluid flow and the structure of the extracellular space in the deep brain. Aging Dis. (2018) 9:808–16. doi: 10.14336/AD.2017.1115
134. Haj-Yasein NN, Vindedal GF, Eilert-Olsen M, Gundersen GA, Skare Ø, Laake P, et al. Glial-conditional deletion of aquaporin-4 (Aqp4) reduces blood-brain water uptake and confers barrier function on perivascular astrocyte endfeet. Proc Natl Acad Sci USA. (2011) 108:17815–20. doi: 10.1073/pnas.1110655108
135. Bloch O, Auguste KI, Manley GT, Verkman AS. Accelerated progression of kaolin-induced hydrocephalus in aquaporin-4-deficient mice. J Cereb Blood Flow Metab. (2006) 26:1527–37. doi: 10.1038/sj.jcbfm.9600306
136. Papadopoulos MC, Manley GT, Krishna S, Verkman AS. Aquaporin-4 facilitates reabsorption of excess fluid in vasogenic brain edema. FASEB J. (2004) 18:1291–3. doi: 10.1096/fj.04-1723fje
137. Binder DK, Papadopoulos MC, Haggie PM, Verkman AS. In vivo measurement of brain extracellular space diffusion by cortical surface photobleaching. J Neurosci. (2004) 24:8049–56. doi: 10.1523/JNEUROSCI.2294-04.2004
138. Iliff JJ, Wang M, Liao Y, Plogg BA, Peng W, Gundersen GA, et al. A paravascular pathway facilitates CSF flow through the brain parenchyma and the clearance of interstitial solutes, including amyloid β. Sci Transl Med. (2012) 4:147ra111. doi: 10.1126/scitranslmed.3003748
139. Zhang R, Liu Y, Chen Y, Li Q, Marshall C, Wu T, et al. Aquaporin 4 deletion exacerbates brain impairments in a mouse model of chronic sleep disruption. CNS Neurosci Ther. (2020) 26:228–39. doi: 10.1111/cns.13194
140. Cao X, Xu H, Feng W, Su D, Xiao M. Deletion of aquaporin-4 aggravates brain pathology after blocking of the meningeal lymphatic drainage. Brain Res Bull. (2018) 143:83–96. doi: 10.1016/j.brainresbull.2018.10.007
141. Iliff JJ, Chen MJ, Plog BA, Zeppenfeld DM, Soltero M, Yang L, et al. Impairment of glymphatic pathway function promotes tau pathology after traumatic brain injury. J Neurosci. (2014) 34:16180–93. doi: 10.1523/JNEUROSCI.3020-14.2014
142. Rosu GC, Catalin B, Balseanu TA, Laurentiu M, Claudiu M, Kumar-Singh S, et al. Inhibition of aquaporin 4 decreases amyloid Aβ40 drainage around cerebral vessels. Mol Neurobiol. (2020) 57:4720–34. doi: 10.1007/s12035-020-02044-8
143. Xu Z, Xiao N, Chen Y, Huang H, Marshall C, Gao J, et al. Deletion of aquaporin-4 in APP/PS1 mice exacerbates brain Aβ accumulation and memory deficits. Mol Neurodegener. (2015) 10:58. doi: 10.1186/s13024-015-0056-1
144. Achariyar TM, Li B, Peng W, Verghese PB, Shi Y, McConnell E, et al. Glymphatic distribution of CSF-derived apoE into brain is isoform specific and suppressed during sleep deprivation. Mol Neurodegener. (2016) 11:74. doi: 10.1186/s13024-016-0138-8
145. Salman MM, Kitchen P, Halsey A, Wang MX, Tornroth-Horsefield S, Conner AC, et al. Emerging roles for dynamic aquaporin-4 subcellular relocalization in CNS water homeostasis. Brain. (2021). doi: 10.1093/brain/awab311 [Epub ahead of print].
146. Mestre H, Hablitz LM, Xavier AL, Feng W, Zou W, Pu T, et al. Aquaporin-4-dependent glymphatic solute transport in the rodent brain. eLife. (2018) 7:40070. doi: 10.7554/eLife.40070
147. Kress BT, Iliff JJ, Xia M, Wang M, Wei HS, Zeppenfeld D, et al. Impairment of paravascular clearance pathways in the aging brain. Ann Neurol. (2014) 76:845–61. doi: 10.1002/ana.24271
148. Ren Z, Iliff JJ, Yang L, Yang J, Chen X, Chen MJ, Giese RN, Wang B, Shi X, Nedergaard M. 'Hit & Run' model of closed-skull traumatic brain injury (TBI) reveals complex patterns of post-traumatic AQP4 dysregulation. J Cereb Blood Flow Metab. (2013) 33:834–45. doi: 10.1038/jcbfm.2013.30
149. Wang M, Iliff JJ, Liao Y, Chen MJ, Shinseki MS, Venkataraman A, et al. Cognitive deficits and delayed neuronal loss in a mouse model of multiple microinfarcts. J Neurosci. (2012) 32:17948–60. doi: 10.1523/JNEUROSCI.1860-12.2012
150. Smith AJ, Yao X, Dix JA, Jin BJ, Verkman AS. Test of the 'glymphatic' hypothesis demonstrates diffusive and aquaporin-4-independent solute transport in rodent brain parenchyma. eLife. (2017) 6:27679. doi: 10.7554/eLife.27679
151. Smith AJ, Akdemir G, Wadhwa M, Song D, Verkman AS. Application of fluorescent dextrans to the brain surface under constant pressure reveals AQP4-independent solute uptake. J Gen Physiol. (2021) 153:e202112898. doi: 10.1085/jgp.202112898
152. Rungta RL, Zuend M, Aydin AK, Martineau É, Boido D, Weber B, et al. Diversity of neurovascular coupling dynamics along vascular arbors in layer II/III somatosensory cortex. Commun Biol. (2021) 4:855. doi: 10.1038/s42003-021-02382-w
153. Csipo T, Lipecz A, Mukli P, Bahadli D, Abdulhussein O, Owens CD, et al. Increased cognitive workload evokes greater neurovascular coupling responses in healthy young adults. PLoS ONE. (2021) 16:e0250043. doi: 10.1371/journal.pone.0250043
154. Lecrux C, Hamel E. Neuronal networks and mediators of cortical neurovascular coupling responses in normal and altered brain states. Philos Trans R Soc Lond B Biol Sci. (2016) 371:20150350. doi: 10.1098/rstb.2015.0350
155. Uhlirova H, Kiliç K, Tian P, Thunemann M, Desjardins M, Saisan PA, et al. Cell type specificity of neurovascular coupling in cerebral cortex. eLife. (2016) 5:14315. doi: 10.7554/eLife.14315
156. Kleinfeld D, Blinder P, Drew PJ, Driscoll JD, Muller A, Tsai PS, et al. A guide to delineate the logic of neurovascular signaling in the brain. Front Neuroenerget. (2011) 3:1. doi: 10.3389/fnene.2011.00001
157. Cauli B, Hamel E. Revisiting the role of neurons in neurovascular coupling. Front Neuroenerget. (2010) 2:9. doi: 10.3389/fnene.2010.00009
158. Buxton RB, Griffeth VE, Simon AB, Moradi F, Shmuel A. Variability of the coupling of blood flow and oxygen metabolism responses in the brain: a problem for interpreting BOLD studies but potentially a new window on the underlying neural activity. Front Neurosci. (2014) 8:139. doi: 10.3389/fnins.2014.00241
159. Kiyatkin EA. Central and peripheral mechanisms underlying physiological and drug-induced fluctuations in brain oxygen in freely-moving rats. Front Integr Neurosci. (2018) 12:44. doi: 10.3389/fnint.2018.00044
160. Shi C, Lei Y, Han H, Zuo L, Yan J, He Q, et al. Transportation in the interstitial space of the brain can be regulated by neuronal excitation. Sci Rep. (2015) 5:17673. doi: 10.1038/srep17673
161. Tuura RO, Volk C, Callaghan F, Jaramillo V, Huber R. Sleep-related and diurnal effects on brain diffusivity and cerebrospinal fluid flow. Neuroimage. (2021) 241:118420. doi: 10.1016/j.neuroimage.2021.118420
162. Hablitz LM, Plá V, Giannetto M, Vinitsky HS, Stæger FF, Metcalfe T, et al. Circadian control of brain glymphatic and lymphatic fluid flow. Nat Commun. (2020) 11:4411. doi: 10.1038/s41467-020-18115-2
163. Xie L, Kang H, Xu Q, Chen MJ, Liao Y, Thiyagarajan M, et al. Sleep drives metabolite clearance from the adult brain. Science. (2013) 342:373–7. doi: 10.1126/science.1241224
164. Hablitz LM, Vinitsky HS, Sun Q, Stæger FF, Sigurdsson B, Mortensen KN, et al. Increased glymphatic influx is correlated with high EEG delta power and low heart rate in mice under anesthesia. Sci Adv. (2019) 5:eaav5447. doi: 10.1126/sciadv.aav5447
165. Benveniste H, Lee H, Ding F, Sun Q, Al-Bizri E, Makaryus R, et al. Anesthesia with dexmedetomidine and low-dose isoflurane increases solute transport via the glymphatic pathway in rat brain when compared with high-dose isoflurane. Anesthesiology. (2017) 127:976–88. doi: 10.1097/ALN.0000000000001888
166. Stanton EH, Persson N, Gomolka RS, Lilius T, Sigurð*sson B, Lee H, et al. Mapping of CSF transport using high spatiotemporal resolution dynamic contrast-enhanced MRI in mice: effect of anesthesia. Magn Reson Med. (2021) 85:3326–42. doi: 10.1002/mrm.28645
167. Bèchet NB, Kylkilahti TM, Mattsson B, Petrasova M, Shanbhag NC, Lundgaard I. Light sheet fluorescence microscopy of optically cleared brains for studying the glymphatic system. J Cereb Blood Flow Metab. (2020) 40:1975–86. doi: 10.1177/0271678X20924954
168. Gakuba C, Gaberel T, Goursaud S, Bourges J, Di Palma C, Quenault A, et al. Martinez de Lizarrondo, Vivien D, Gauberti M. General anesthesia inhibits the activity of the “glymphatic system”. Theranostics. (2018) 8:710–22. doi: 10.7150/thno.19154
169. Benveniste H, Heerdt PM, Fontes M, Rothman DL, Volkow ND. Glymphatic system function in relation to anesthesia and sleep states. Anesth Analg. (2019) 128:747–58. doi: 10.1213/ANE.0000000000004069
170. DiNuzzo M, Nedergaard M. Brain energetics during the sleep-wake cycle. Curr Opin Neurobiol. (2017) 47:65–72. doi: 10.1016/j.conb.2017.09.010
171. Li Y, Han H, Shi K, Cui D, Yang J, Alberts IL, et al. The mechanism of downregulated interstitial fluid drainage following neuronal excitation. Aging Dis. (2020) 11:1407–22. doi: 10.14336/AD.2020.0224
172. Liu DX, He X, Wu D, Zhang Q, Yang C, Liang FY, et al. Continuous theta burst stimulation facilitates the clearance efficiency of the glymphatic pathway in a mouse model of sleep deprivation. Neurosci Lett. (2017) 653:189–94. doi: 10.1016/j.neulet.2017.05.064
173. Oku H, Morishita S, Horie T, Kida T, Mimura M, Fukumoto M, et al. Nitric oxide increases the expression of aquaporin-4 protein in rat optic nerve astrocytes through the cyclic guanosine monophosphate/protein kinase G pathway. Ophthalmic Res. (2015) 54:212–21. doi: 10.1159/000440846
174. Rao KV, Jayakumar AR, Reddy PV, Tong X, Curtis KM, Norenberg MD. Aquaporin-4 in manganese-treated cultured astrocytes. Glia. (2010) 58:1490–9. doi: 10.1002/glia.21023
175. Mohammadi MT, Dehghani GA. Nitric oxide as a regulatory factor for aquaporin-1 and 4 gene expression following brain ischemia/reperfusion injury in rat. Pathol Res Pract. (2015) 211:43–9. doi: 10.1016/j.prp.2014.07.014
176. Lykke K, Assentoft M, Fenton RA, Rosenkilde MM, MacAulay N. Vasopressin receptors V1a and V2 are not osmosensors. Physiol Rep. (2015) 3:12519. doi: 10.14814/phy2.12519
177. Nakayama S, Amiry-Moghaddam M, Ottersen OP, Bhardwaj A. Conivaptan, a selective arginine vasopressin V1a and V2 receptor antagonist attenuates global cerebral edema following experimental cardiac arrest via perivascular pool of aquaporin-4. Neurocrit Care. (2016) 24:273–82. doi: 10.1007/s12028-015-0236-4
178. Rauen K, Pop V, Trabold R, Badaut J, Plesnila N. Vasopressin V(1a) receptors regulate cerebral aquaporin 1 after traumatic brain injury. J Neurotrauma. (2020) 37:665–74. doi: 10.1089/neu.2019.6653
179. Saito M, Tanaka H, Sasaki M, Kurose H, Nakahata N. Involvement of aquaporin in thromboxane A2 receptor-mediated, G 12/13/RhoA/NHE-sensitive cell swelling in 1321N1 human astrocytoma cells. Cell Signal. (2010) 22:41–6. doi: 10.1016/j.cellsig.2009.09.006
180. Larsen BR, MacAulay N. Activity-dependent astrocyte swelling is mediated by pH-regulating mechanisms. Glia. (2017) 65:1668–81. doi: 10.1002/glia.23187
181. Rosic B, Dukefoss DB, Åbjørsbråten KS, Tang W, Jensen V, Ottersen OP, Enger R, et al. Aquaporin-4-independent volume dynamics of astroglial endfeet during cortical spreading depression. Glia. (2019) 67:1113–21. doi: 10.1002/glia.23604
182. MacAulay N. Molecular mechanisms of K(+) clearance extracellular space shrinkage-Glia cells as the stars. Glia. (2020) 68:2192–211. doi: 10.1002/glia.23824
183. Amiry-Moghaddam M, Williamson A, Palomba M, Eid T, de Lanerolle NC, Nagelhus EA, et al. Delayed K+ clearance associated with aquaporin-4 mislocalization: phenotypic defects in brains of alpha-syntrophin-null mice. Proc Natl Acad Sci USA. (2003) 100:13615–20. doi: 10.1073/pnas.2336064100
184. Yao X, Smith AJ, Jin BJ, Zador Z, Manley GT, Verkman AS. Aquaporin-4 regulates the velocity and frequency of cortical spreading depression in mice. Glia. (2015) 63:1860–9. doi: 10.1002/glia.22853
185. Enger R, Dukefoss DB, Tang W, Pettersen KH, Bjørnstad DM, Helm PJ, et al. Deletion of aquaporin-4 curtails extracellular glutamate elevation in cortical spreading depression in awake mice. Cereb Cortex. (2017) 27:24–33. doi: 10.1093/cercor/bhw359
186. Binder DK, Yao X, Zador Z, Sick TJ, Verkman AS, Manley GT. Increased seizure duration and slowed potassium kinetics in mice lacking aquaporin-4 water channels. Glia. (2006) 53:631–6. doi: 10.1002/glia.20318
187. Binder DK, Oshio K, Ma T, Verkman AS, Manley GT. Increased seizure threshold in mice lacking aquaporin-4 water channels. Neuroreport. (2004) 15:259–62. doi: 10.1097/00001756-200402090-00009
188. Szu JI, Patel DD, Chaturvedi S, Lovelace JW, Binder DK. Modulation of posttraumatic epileptogenesis in aquaporin-4 knockout mice. Epilepsia. (2020) 61:1503–14. doi: 10.1111/epi.16551
189. Lei S, He Y, Zhu Z, Liu Z, Lin Y, He Y, et al. Inhibition of NMDA receptors downregulates astrocytic AQP4 to suppress seizures. Cell Mol Neurobiol. (2020) 40:1283–95. doi: 10.1007/s10571-020-00813-6
190. Szu JI, Chaturvedi S, Patel DD, Binder DK. Aquaporin-4 dysregulation in a controlled cortical impact injury model of posttraumatic epilepsy. Neuroscience. (2020) 428:140–53. doi: 10.1016/j.neuroscience.2019.12.006
191. Eid T, Lee TS, Thomas MJ, Amiry-Moghaddam M, Bjørnsen LP, Spencer DD, et al. C. de Lanerolle, Loss of perivascular aquaporin 4 may underlie deficient water and K+ homeostasis in the human epileptogenic hippocampus. Proc Natl Acad Sci USA. (2005) 102:1193–8. doi: 10.1073/pnas.0409308102
192. Lee TS, Eid T, Mane S, Kim JH, Spencer DD, Ottersen OP, et al.C. de Lanerolle, Aquaporin-4 is increased in the sclerotic hippocampus in human temporal lobe epilepsy. Acta Neuropathol. (2004) 108:493–502. doi: 10.1007/s00401-004-0910-7
193. Mhatre R, Anita M, Phillip M, Saini J, Arimappamagan A, Bharath RD, et al. Altered vascular permeability but not angiogenesis may play a role in the epileptogenesis of human hippocampal sclerosis. Epileptic Disord. (2021) 23:490–9. doi: 10.1684/epd.2021.1290
194. Chang JC, Shook LL, Biag J, Nguyen EN, Toga AW, Charles AC, et al. Biphasic direct current shift, haemoglobin desaturation and neurovascular uncoupling in cortical spreading depression. Brain. (2010) 133:996–1012. doi: 10.1093/brain/awp338
195. Oka F, Sadeghian H, Yaseen MA, Fu B, Kura S, Qin T, et al. Intracranial pressure spikes trigger spreading depolarizations. Brain. (2021). doi: 10.1093/brain/awab256 [Epub ahead of print].
196. Moshkforoush A, Ashenagar B, Harraz OF, Dabertrand F, Longden TA, Nelson MT, et al. The capillary Kir channel as sensor and amplifier of neuronal signals: modeling insights on K(+)-mediated neurovascular communication. Proc Natl Acad Sci USA. (2020) 117:16626–37. doi: 10.1073/pnas.2000151117
197. Koide M, Moshkforoush A, Tsoukias NM, Hill-Eubanks DC, Wellman GC, Nelson MT, et al. The yin and yang of K(V) channels in cerebral small vessel pathologies. Microcirculation. (2018) 25:12436. doi: 10.1111/micc.12436
198. Staehr C, Rajanathan R, Matchkov VV. Involvement of the Na(+),K(+) -ATPase isoforms in control of cerebral perfusion. Exp Physiol. (2019) 104:1023–8. doi: 10.1113/EP087519
199. Staehr C, Rajanathan R, Postnov DD, Hangaard L, Bouzinova EV, Lykke-Hartmann K, et al. Abnormal neurovascular coupling as a cause of excess cerebral vasodilation in familial migraine. Cardiovasc Res. (2020) 116:2009–20. doi: 10.1093/cvr/cvz306
200. Migliati ER, Amiry-Moghaddam M, Froehner SC, Adams ME, Ottersen OP, Bhardwaj A. Na(+)-K (+)-2Cl (-) cotransport inhibitor attenuates cerebral edema following experimental stroke via the perivascular pool of aquaporin-4. Neurocrit Care. (2010) 13:123–31. doi: 10.1007/s12028-010-9376-8
201. Monai H, Wang X, Yahagi K, Lou N, Mestre H, Xu Q, et al. Adrenergic receptor antagonism induces neuroprotection and facilitates recovery from acute ischemic stroke. Proc Natl Acad Sci USA. (2019) 116:11010–11019. doi: 10.1073/pnas.1817347116
202. Steiner E, Enzmann GU, Lin S, Ghavampour S, Hannocks MJ, Zuber B, et al. Loss of astrocyte polarization upon transient focal brain ischemia as a possible mechanism to counteract early edema formation. Glia. (2012) 60:1646–59. doi: 10.1002/glia.22383
203. Yan JH, Khatibi NH, Han HB, Hu Q, Chen CH, Li L, et al. p53-induced uncoupling expression of aquaporin-4 and inwardly rectifying K+ 4.1 channels in cytotoxic edema after subarachnoid hemorrhage. CNS Neurosci Ther. (2012) 18:334–42. doi: 10.1111/j.1755-5949.2012.00299.x
204. Anzabi M, Ardalan M, Iversen NK, Rafati AH, Hansen B, Østergaard L. Hippocampal atrophy following subarachnoid hemorrhage correlates with disruption of astrocyte morphology and capillary coverage by AQP4. Front Cell Neurosci. (2018) 12:19. doi: 10.3389/fncel.2018.00019
205. Rickels E, Zumkeller M. Vasospasm after experimentally induced subarachnoid haemorrhage and treatment with nimodipine. Neurochirurgia. (1992) 35:99–102. doi: 10.1055/s-2008-1052257
206. Young HA, Kolbeck RC, Schmidek H, Evans JN. Reactivity of rabbit basilar artery to alterations in extracellular potassium and calcium after subarachnoid hemorrhage. Neurosurgery. (1986) 19:346–9. doi: 10.1227/00006123-198609000-00002
207. Pu T, Zou W, Feng W, Zhang Y, Wang L, Wang H, et al. Persistent malfunction of glymphatic and meningeal lymphatic drainage in a mouse model of subarachnoid hemorrhage. Exp Neurobiol. (2019) 28:104–18. doi: 10.5607/en.2019.28.1.104
208. Bao H, Yang X, Huang Y, Qiu H, Huang G, Xiao H, et al. The neuroprotective effect of apelin-13 in a mouse model of intracerebral hemorrhage. Neurosci Lett. (2016) 628:219–24. doi: 10.1016/j.neulet.2016.06.046
209. Chu H, Tang Y, Dong Q. Protection of vascular endothelial growth factor to brain edema following intracerebral hemorrhage and its involved mechanisms: effect of aquaporin-4. PLoS ONE. (2013) 8:e66051. doi: 10.1371/journal.pone.0066051
210. Taya K, Gulsen S, Okuno K, Prieto R, Marmarou CR, Marmarou A. Modulation of AQP4 expression by the selective V1a receptor antagonist, SR49059, decreases trauma-induced brain edema. Acta Neurochir Suppl. (2008) 102:425–9. doi: 10.1007/978-3-211-85578-2_83
211. Reinert M, Khaldi A, Zauner A, Doppenberg E, Choi S, Bullock R. High extracellular potassium and its correlates after severe head injury: relationship to high intracranial pressure. Neurosurg Focus. (2000) 8:e10. doi: 10.3171/foc.2000.8.1.2027
212. Reeves TM, Kao CQ, Phillips LL, Bullock MR, Povlishock JT. Presynaptic excitability changes following traumatic brain injury in the rat. J Neurosci Res. (2000) 60:370–9. doi: 10.1002/(SICI)1097-4547(20000501)60:3<370::AID-JNR12>3.0.CO;2-B
213. Darbin O, Carre E, Naritoku D, Risso JJ, Lonjon M, Patrylo PR. Glucose metabolites in the striatum of freely behaving rats following infusion of elevated potassium. Brain Res. (2006) 1116:127–31. doi: 10.1016/j.brainres.2006.06.095
214. Klatzo I. Pathophysiological aspects of brain edema. Acta Neuropathol. (1987) 72:236–9. doi: 10.1007/BF00691095
216. Kimelberg HK, Current Current concepts of brain edema. review of laboratory investigations. J Neurosurg. (1995) 83:1051–9. doi: 10.3171/jns.1995.83.6.1051
217. Simard JM, Kent TA, Chen M, Tarasov KV, Gerzanich V. Brain oedema in focal ischaemia: molecular pathophysiology and theoretical implications. Lancet Neurol. (2007) 6:258–68. doi: 10.1016/S1474-4422(07)70055-8
218. Badaut J, Ashwal S, Obenaus A. Aquaporins in cerebrovascular disease: a target for treatment of brain edema? Cerebrovasc Dis. (2011) 31:521–31. doi: 10.1159/000324328
219. Stiefel MF, Tomita Y, Marmarou A. Secondary ischemia impairing the restoration of ion homeostasis following traumatic brain injury. J Neurosurg. (2005) 103:707–14. doi: 10.3171/jns.2005.103.4.0707
220. Barzó P, Marmarou A, Fatouros P, Hayasaki K, Corwin F. Contribution of vasogenic and cellular edema to traumatic brain swelling measured by diffusion-weighted imaging. J Neurosurg. (1997) 87:900–7. doi: 10.3171/jns.1997.87.6.0900
221. Marmarou A, Portella G, Barzo P, Signoretti S, Fatouros P, Beaumont A, et al. Distinguishing between cellular and vasogenic edema in head injured patients with focal lesions using magnetic resonance imaging. Acta Neurochir Suppl. (2000) 76:349–51. doi: 10.1007/978-3-7091-6346-7_72
222. Beaumont A, Marmarou A, Fatouros P, Corwin F. Secondary insults worsen blood brain barrier dysfunction assessed by MRI in cerebral contusion. Acta Neurochir Suppl. (2002) 81:217–9. doi: 10.1007/978-3-7091-6738-0_56
223. Marmarou A, Signoretti S, Aygok G, Fatouros P, Portella G. Traumatic brain edema in diffuse and focal injury: cellular or vasogenic? Acta Neurochir Suppl. (2006) 96:24–9. doi: 10.1007/3-211-30714-1_6
224. Marmarou A, Signoretti S, Fatouros PP, Portella G, Aygok GA, Bullock MR. Predominance of cellular edema in traumatic brain swelling in patients with severe head injuries. J Neurosurg. (2006) 104:720–30. doi: 10.3171/jns.2006.104.5.720
225. Young W, Rappaport ZH, Chalif DJ, Flamm ES. Regional brain sodium, potassium, and water changes in the rat middle cerebral artery occlusion model of ischemia. Stroke. (1987) 18:751–9. doi: 10.1161/01.STR.18.4.751
226. Marmarou A, A review of progress in understanding the pathophysiology and treatment of brain edema. Neurosurg Focus. (2007) 22:E1. doi: 10.3171/foc.2007.22.5.2
227. Ke C, Poon WS, Ng HK, Pang JC, Chan Y. Heterogeneous responses of aquaporin-4 in oedema formation in a replicated severe traumatic brain injury model in rats. Neurosci Lett. (2001) 301:21–4. doi: 10.1016/S0304-3940(01)01589-0
228. Kiening KL, van Landeghem FK, Schreiber S, Thomale UW, von Deimling A, Unterberg AW, et al. Decreased hemispheric Aquaporin-4 is linked to evolving brain edema following controlled cortical impact injury in rats. Neurosci Lett. (2002) 324:105–8. doi: 10.1016/S0304-3940(02)00180-5
229. Blixt J, Svensson M, Gunnarson E, Wanecek M. Aquaporins and blood-brain barrier permeability in early edema development after traumatic brain injury. Brain Res. (2015) 1611:18–28. doi: 10.1016/j.brainres.2015.03.004
230. Fukuda AM, Pop V, Spagnoli D, Ashwal S, Obenaus A, Badaut J. Delayed increase of astrocytic aquaporin 4 after juvenile traumatic brain injury: possible role in edema resolution? Neuroscience. (2012) 222:366–78. doi: 10.1016/j.neuroscience.2012.06.033
231. Taya K, Marmarou CR, Okuno K, Prieto R, Marmarou A. Effect of secondary insults upon aquaporin-4 water channels following experimental cortical contusion in rats. J Neurotrauma. (2010) 27:229–39. doi: 10.1089/neu.2009.0933
232. Zhang C, Chen J, Lu H. Expression of aquaporin-4 and pathological characteristics of brain injury in a rat model of traumatic brain injury. Mol Med Rep. (2015) 12:7351–7. doi: 10.3892/mmr.2015.4372
233. Chmelova M, Sucha P, Bochin M, Vorisek I, Pivonkova H, Hermanova Z, et al. The role of aquaporin-4 and transient receptor potential vaniloid isoform 4 channels in the development of cytotoxic edema and associated extracellular diffusion parameter changes. Eur J Neurosci. (2019) 50:1685–99. doi: 10.1111/ejn.14338
234. Yao X, Derugin N, Manley GT, Verkman AS. Reduced brain edema and infarct volume in aquaporin-4 deficient mice after transient focal cerebral ischemia. Neurosci Lett. (2015) 584:368–72. doi: 10.1016/j.neulet.2014.10.040
235. Liu X, Zhang W, Alkayed NJ, Froehner SC, Adams ME, Amiry-Moghaddam M, et al. Lack of sex-linked differences in cerebral edema and aquaporin-4 expression after experimental stroke. J Cereb Blood Flow Metab. (2008) 28:1898–906. doi: 10.1038/jcbfm.2008.83
236. Hirt L, Fukuda AM, Ambadipudi K, Rashid F, Binder D, Verkman A, et al. Improved long-term outcome after transient cerebral ischemia in aquaporin-4 knockout mice. J Cereb Blood Flow Metab. (2017) 37:277–90. doi: 10.1177/0271678X15623290
237. Akdemir G, Ratelade J, Asavapanumas N, Verkman AS. Neuroprotective effect of aquaporin-4 deficiency in a mouse model of severe global cerebral ischemia produced by transient 4-vessel occlusion. Neurosci Lett. (2014) 574:70–5. doi: 10.1016/j.neulet.2014.03.073
238. Katada R, Akdemir G, Asavapanumas N, Ratelade J, Zhang H, Verkman AS. Greatly improved survival and neuroprotection in aquaporin-4-knockout mice following global cerebral ischemia. FASEB J. (2014) 28:705–14. doi: 10.1096/fj.13-231274
239. Tang Y, Wu P, Su J, Xiang J, Cai D, Dong Q. Effects of Aquaporin-4 on edema formation following intracerebral hemorrhage. Exp Neurol. (2010) 223:485–95. doi: 10.1016/j.expneurol.2010.01.015
240. Chiu CD, Chen CC, Shen CC, Chin LT, Ma HI, Chuang HY, et al. Hyperglycemia exacerbates intracerebral hemorrhage via the downregulation of aquaporin-4: temporal assessment with magnetic resonance imaging. Stroke. (2013) 44:1682–9. doi: 10.1161/STROKEAHA.113.675983
241. Qiu GP, Xu J, Zhuo F, Sun SQ, Liu H, Yang M, et al. Loss of AQP4 polarized localization with loss of β-dystroglycan immunoreactivity may induce brain edema following intracerebral hemorrhage. Neurosci Lett. (2015) 588:42–8. doi: 10.1016/j.neulet.2014.12.053
242. Bloch O, Papadopoulos MC, Manley GT, Verkman AS. Aquaporin-4 gene deletion in mice increases focal edema associated with staphylococcal brain abscess. J Neurochem. (2005) 95:254–62. doi: 10.1111/j.1471-4159.2005.03362.x
243. Papadopoulos MC, Verkman AS. Aquaporin-4 gene disruption in mice reduces brain swelling and mortality in pneumococcal meningitis. J Biol Chem. (2005) 280:13906–12. doi: 10.1074/jbc.M413627200
244. Gao M, Lu W, Shu Y, Yang Z, Sun S, Xu J, et al. Poldip2 mediates blood-brain barrier disruption and cerebral edema by inducing AQP4 polarity loss in mouse bacterial meningitis model. CNS Neurosci Ther. (2020) 26:1288–302. doi: 10.1111/cns.13446
245. Huang J, Lu WT, Sun SQ, Yang ZB, Huang SQ, Gan SW, et al. Upregulation and lysosomal degradation of AQP4 in rat brains with bacterial meningitis. Neurosci Lett. (2014) 566:156–61. doi: 10.1016/j.neulet.2014.02.054
246. McCoy ES, Haas BR, Sontheimer H. Water permeability through aquaporin-4 is regulated by protein kinase C and becomes rate-limiting for glioma invasion. Neuroscience. (2010) 168:971–81. doi: 10.1016/j.neuroscience.2009.09.020
247. Osier ND, Dixon CE. The controlled cortical impact model: applications, considerations for researchers, future directions. Front Neurol. (2016) 7:134. doi: 10.3389/fneur.2016.00134
248. Fukuda AM, Adami A, Pop V, Bellone JA, Coats JS, Hartman RE, et al. Posttraumatic reduction of edema with aquaporin-4 RNA interference improves acute and chronic functional recovery. J Cereb Blood Flow Metab. (2013) 33:1621–32. doi: 10.1038/jcbfm.2013.118
249. Quintard H, Lorivel T, Gandin C, Lazdunski M, Heurteaux C. MLC901, a Traditional Chinese Medicine induces neuroprotective and neuroregenerative benefits after traumatic brain injury in rats. Neuroscience. (2014) 277:72–86. doi: 10.1016/j.neuroscience.2014.06.047
250. Tomura S, Nawashiro H, Otani N, Uozumi Y, Toyooka T, Ohsumi A, et al. Effect of decompressive craniectomy on aquaporin-4 expression after lateral fluid percussion injury in rats. J Neurotrauma. (2011) 28:237–43. doi: 10.1089/neu.2010.1443
251. Szczygielski J, Glameanu C, Müller A, Klotz M, Sippl C, Hubertus V, et al. Changes in posttraumatic brain edema in craniectomy-selective brain hypothermia model are associated with modulation of aquaporin-4 level. Front Neurol. (2018) 9:799. doi: 10.3389/fneur.2018.00799
252. Yao X, Uchida K, Papadopoulos MC, Zador Z, Manley GT, Verkman AS. Mildly reduced brain swelling and improved neurological outcome in aquaporin-4 knockout mice following controlled cortical impact brain injury. J Neurotrauma. (2015) 32:1458–64. doi: 10.1089/neu.2014.3675
253. Liang F, Luo C, Xu G, Su F, He X, Long S, et al. Deletion of aquaporin-4 is neuroprotective during the acute stage of micro traumatic brain injury in mice. Neurosci Lett. (2015) 598:29–35. doi: 10.1016/j.neulet.2015.05.006
254. Kitchen P, Salman MM, Abir-Awan M, Al-Jubair T, Törnroth-Horsefield S, Conner AC, et al. Calcein fluorescence quenching to measure plasma membrane water flux in live mammalian cells. STAR Protoc. (2020) 1:100157. doi: 10.1016/j.xpro.2020.100157
255. Verkman AS, Anderson MO, Papadopoulos MC. Aquaporins: important but elusive drug targets. Nat Rev Drug Discov. (2014) 13:259–77. doi: 10.1038/nrd4226
256. Abir-Awan M, Kitchen P, Salman MM, Conner MT, Conner AC, Bill RM. Inhibitors of mammalian aquaporin water channels. Int J Mol Sci. (2019) 20: doi: 10.3390/ijms20071589
257. Blixt J, Gunnarson E, Wanecek M. Erythropoietin attenuates the brain edema response after experimental traumatic brain injury. J Neurotrauma. (2018) 35:671–80. doi: 10.1089/neu.2017.5015
258. Rizwan Siddiqui M., Attar F, Mohanty V, Kim KS, Shekhar Mayanil C, Tomita T. Erythropoietin-mediated activation of aquaporin-4 channel for the treatment of experimental hydrocephalus. Childs Nerv Syst. (2018) 34:2195–2202. doi: 10.1007/s00381-018-3865-z
259. Wang R, Wu X, Zhao H, Min L, Tao Z, Ji X, et al. Effects of erythropoietin combined with tissue plasminogen activator on the rats following cerebral ischemia and reperfusion. Brain Circ. (2016) 2:54–60. doi: 10.4103/2394-8108.178552
260. Zhang M, Cui Z, Cui H, Cao Y, Zhong C, Wang Y. Astaxanthin alleviates cerebral edema by modulating NKCC1 and AQP4 expression after traumatic brain injury in mice. BMC Neurosci. (2016) 17:60. doi: 10.1186/s12868-016-0295-2
261. Liu L, Kelly MG, Wierzbicki EL, Escober-Nario IC, Vollmer MK, Doré S. Nrf2 plays an essential role in long-term brain damage and neuroprotection of korean red ginseng in a permanent cerebral ischemia model. Antioxidants. (2019) 8:8080273. doi: 10.3390/antiox8080273
262. Liu YL, Xu ZM, Yang GY, Yang DX, Ding J, Chen H, et al. Sesamin alleviates blood-brain barrier disruption in mice with experimental traumatic brain injury. Acta Pharmacol Sin. (2017) 38:1445–55. doi: 10.1038/aps.2017.103
263. Wang X, An F, Wang S, An Z, Wang S. Orientin attenuates cerebral ischemia/reperfusion injury in rat model through the AQP-4 and TLR4/NF-κB/TNF-α signaling pathway. J Stroke Cerebrovasc Dis. (2017) 26:2199–2214. doi: 10.1016/j.jstrokecerebrovasdis.2017.05.002
264. Xu LX, Lv Y, Li YH, Ding X, Wang Y, Han X, et al. Melatonin alleviates brain and peripheral tissue edema in a neonatal rat model of hypoxic-ischemic brain damage: the involvement of edema related proteins. BMC Pediatr. (2017) 17:90. doi: 10.1186/s12887-017-0824-x
265. Arima H, Yamamoto N, Sobue K, Umenishi F, Tada T, Katsuya H, et al. Hyperosmolar mannitol simulates expression of aquaporins 4 and 9 through a p38 mitogen-activated protein kinase-dependent pathway in rat astrocytes. J Biol Chem. (2003) 278:44525–34. doi: 10.1074/jbc.M304368200
266. Jiang W, Cao WJ, Zhang YK, Wei XY, Kuang F. Bolus injection of hypertonic solutions for cerebral edema in rats: challenge of homeostasis of healthy brain. Neurosci Lett. (2012) 509:44–9. doi: 10.1016/j.neulet.2011.12.045
267. Szczygielski J, Hubertus V, Kruchten E, Müller A, Albrecht LF, Mautes AE, et al. Brain edema formation and functional outcome after surgical decompression in murine closed head injury are modulated by acetazolamide administration. Front Neurol. (2019) 10:273. doi: 10.3389/fneur.2019.00273
268. Hao JQ, He XY, Yang X, Xiao YC, Duan SQ, Wang H, et al. Acetazolamide alleviate cerebral edema induced by ischemic stroke through inhibiting the expression of AQP4 mRNA. Neurocrit Care. (2021) doi: 10.1007/s12028-021-01261-w
269. Glober NK, Sprague S, Ahmad S, Mayfield KG, Fletcher LM, Digicaylioglu MH, et al. Acetazolamide treatment prevents redistribution of astrocyte aquaporin 4 after murine traumatic brain injury. Neurosci J. (2019) 2019:2831501. doi: 10.1155/2019/2831501
270. Jin H, Li W, Dong C, Ma L, Wu J, Zhao W. Effects of different doses of levetiracetam on aquaporin 4 expression in rats with brain edema following fluid percussion injury. Med Sci Monit. (2016) 22:678–86. doi: 10.12659/MSM.897201
271. Sylvain NJ, Salman MM, Pushie MJ, Hou H, Meher V, Herlo R, et al. The effects of trifluoperazine on brain edema, aquaporin-4 expression and metabolic markers during the acute phase of stroke using photothrombotic mouse model. Biochim Biophys Acta Biomembr. (2021) 1863:183573. doi: 10.1016/j.bbamem.2021.183573
272. Kuroda S, Nakai A, Kristían T, Siesjö BK. The calmodulin antagonist trifluoperazine in transient focal brain ischemia in rats. Anti-ischemic effect and therapeutic window. Stroke. (1997) 28:2539–44. doi: 10.1161/01.STR.28.12.2539
273. Huber VJ, Tsujita M, Yamazaki M, Sakimura K, Nakada T. Identification of arylsulfonamides as Aquaporin 4 inhibitors. Bioorg Med Chem Lett. (2007) 17:1270–3. doi: 10.1016/j.bmcl.2006.12.010
274. Salman MM, Kitchen P, Iliff JJ, Bill RM. Aquaporin 4 and glymphatic flow have central roles in brain fluid homeostasis. Nat Rev Neurosci. (2021) 22:650–1. doi: 10.1038/s41583-021-00514-z
275. MacAulay N, Reply to 'Aquaporin 4 and glymphatic flow have central roles in brain fluid homeostasis'. Nat Rev Neurosci. (2021) 22:651–2. doi: 10.1038/s41583-021-00515-y
276. Igarashi H, Huber VJ, Tsujita M, Nakada T. Pretreatment with a novel aquaporin 4 inhibitor, TGN-020, significantly reduces ischemic cerebral edema. Neurol Sci. (2011) 32:113–6. doi: 10.1007/s10072-010-0431-1
277. Toft-Bertelsen TL, Larsen BR, Christensen SK, Khandelia H, Waagepetersen HS, MacAulay N. Clearance of activity-evoked K(+) transients and associated glia cell swelling occur independently of AQP4: a study with an isoform-selective AQP4 inhibitor. Glia. (2021) 69:28–41. doi: 10.1002/glia.23851
278. Nakhoul NL, Davis BA, Romero MF, Boron WF. Effect of expressing the water channel aquaporin-1 on the CO2 permeability of Xenopus oocytes. Am J Physiol. (1998) 274:C543–8. doi: 10.1152/ajpcell.1998.274.2.C543
279. Huber VJ, Tsujita M, Kwee IL, Nakada T. Inhibition of aquaporin 4 by antiepileptic drugs. Bioorg Med Chem. (2009) 17:418–24. doi: 10.1016/j.bmc.2007.12.038
280. Jha RM, Kochanek PM, Simard JM. Pathophysiology and treatment of cerebral edema in traumatic brain injury. Neuropharmacology. (2019) 145:230–46. doi: 10.1016/j.neuropharm.2018.08.004
281. Papadopoulos MC, Bennett JL, Verkman AS. Treatment of neuromyelitis optica: state-of-the-art and emerging therapies. Nat Rev Neurol. (2014) 10:493–506. doi: 10.1038/nrneurol.2014.141
282. Tradtrantip L, Zhang H, Saadoun S, Phuan P-W, Lam C, Papadopoulos MC, et al. Anti-Aquaporin-4 monoclonal antibody blocker therapy for neuromyelitis optica. Ann Neurol. (2012) 71:314–22. doi: 10.1002/ana.22657
283. Huang P, Takai Y, Kusano-Arai O, Ramadhanti J, Iwanari H, Miyauchi T, et al. The binding property of a monoclonal antibody against the extracellular domains of aquaporin-4 directs aquaporin-4 toward endocytosis. Biochem Biophys Rep. (2016) 7:77–83. doi: 10.1016/j.bbrep.2016.05.017
284. Guan Y, Li L, Chen J, Lu H. Effect of AQP4-RNAi in treating traumatic brain edema: Multi-modal MRI and histopathological changes of early stage edema in a rat model. Exp Ther Med. (2020) 19:2029–36. doi: 10.3892/etm.2020.8456
285. Lu H, Zhan Y, Ai L, Chen H, Chen J. AQP4-siRNA alleviates traumatic brain edema by altering post-traumatic AQP4 polarity reversal in TBI rats. J Clin Neurosci. (2020) 81:113–9. doi: 10.1016/j.jocn.2020.09.015
286. Fujita Y, Yamamoto N, Sobue K, Inagaki M, Ito H, Arima H, et al. Effect of mild hypothermia on the expression of aquaporin family in cultured rat astrocytes under hypoxic condition. Neurosci Res. (2003) 47:437–44. doi: 10.1016/j.neures.2003.08.006
287. Kurisu K, Abumiya T, Nakamura H, Shimbo D, Shichinohe H, Nakayama N, et al. Transarterial regional brain hypothermia inhibits acute aquaporin-4 surge and sequential microvascular events in ischemia/reperfusion injury. Neurosurgery. (2016) 79:125–34. doi: 10.1227/NEU.0000000000001088
288. Gao D, Ding F, Lei G, Luan G, Zhang S, Li K, et al. Effects of focal mild hypothermia on thrombin-induced brain edema formation and the expression of protease activated receptor-1, matrix metalloproteinase-9 and aquaporin 4 in rats. Mol Med Rep. (2015) 11:3009–14. doi: 10.3892/mmr.2014.3111
289. Duan Y, Wu D, Huber M, Shi J, An H, Wei W, et al. New endovascular approach for hypothermia with intrajugular cooling and neuroprotective effect in ischemic stroke. Stroke. (2020) 51:628–36. doi: 10.1161/STROKEAHA.119.026523
290. Dietrich WD, Bramlett HM. Therapeutic hypothermia and targeted temperature management for traumatic brain injury: experimental and clinical experience. Brain Circ. (2017) 3:186–98. doi: 10.4103/bc.bc_28_17
Keywords: aquaporin-4, glymphatic system, brain edema, neruovascular unit, cerebral fluid homeostasis
Citation: Szczygielski J, Kopańska M, Wysocka A and Oertel J (2021) Cerebral Microcirculation, Perivascular Unit, and Glymphatic System: Role of Aquaporin-4 as the Gatekeeper for Water Homeostasis. Front. Neurol. 12:767470. doi: 10.3389/fneur.2021.767470
Received: 30 August 2021; Accepted: 12 November 2021;
Published: 13 December 2021.
Edited by:
Marek Czosnyka, University of Cambridge, United KingdomReviewed by:
Mootaz M. Salman, University of Oxford, United KingdomYoichiro Abe, Keio University, Japan
Copyright © 2021 Szczygielski, Kopańska, Wysocka and Oertel. This is an open-access article distributed under the terms of the Creative Commons Attribution License (CC BY). The use, distribution or reproduction in other forums is permitted, provided the original author(s) and the copyright owner(s) are credited and that the original publication in this journal is cited, in accordance with accepted academic practice. No use, distribution or reproduction is permitted which does not comply with these terms.
*Correspondence: Jacek Szczygielski, jacek.szczygielski@vp.pl