- 1Hotchkiss Brain Institute, Department of Clinical Neurosciences, Cumming School of Medicine, University of Calgary, Calgary, AB, Canada
- 2Centre for Heart and Lung Innovation, Department of Pathology and Laboratory Medicine, University of British Columbia, Vancouver, BC, Canada
- 3Division of Neurology, Department of Medicine, University of Saskatchewan, Saskatoon, SK, Canada
- 4Division of Neurodegenerative Disorders, Department of Biology, Albrechtsen St. Boniface Research Centre, University of Winnipeg, Winnipeg, MB, Canada
- 5Department of Medical Genetics, Alberta Child Health Research Institute, University of Calgary, Calgary, AB, Canada
In this article we review complications to the peripheral nervous system that occur as a consequence of viral infections, with a special focus on complications of severe acute respiratory syndrome coronavirus-2 (SARS-CoV-2). We discuss neuromuscular complications in three broad categories; the direct consequences of viral infection, autoimmune neuromuscular disorders provoked by viral infections, and chronic neurodegenerative conditions which have been associated with viral infections. We also include discussion of neuromuscular disorders that are treated by immunomodulatory therapies, and how this affects patient susceptibility in the current context of the coronavirus disease 2019 (COVID-19) pandemic. COVID-19 is associated with direct consequences to the peripheral nervous system via presumed direct viral injury (dysgeusia/anosmia, myalgias/rhabdomyolysis, and potentially mononeuritis multiplex) and autoimmunity (Guillain Barré syndrome and variants). It has important implications for people receiving immunomodulatory therapies who may be at greater risk of severe outcomes from COVID-19. Thus far, chronic post-COVID syndromes (a.k.a: long COVID) also include possible involvement of the neuromuscular system. Whether we may observe neuromuscular degenerative conditions in the longer term will be an important question to monitor in future studies.
Introduction
The emergence of the coronavirus disease 2019 (COVID-19) pandemic illness caused by severe acute respiratory syndrome coronavirus 2 (SARS-CoV-2) has led to the rapid publication of numerous reports outlining neuromuscular complications. Although many of the major complications of SARS-CoV-2 are related to the respiratory, cardiovascular, and host defense systems, the neuromuscular system can be affected in a large proportion of patients (1). In patients with critical illness or other comorbidities, neuromuscular complications may be difficult to recognize and thus be underdiagnosed (2).
Viral infections may affect the nervous system by a number of differing mechanisms. For the purposes of this review, we present three broad categories, (a) neuromuscular complications which are a direct consequence of viral infection (Table 1), (b) neuromuscular disorders which result from autoimmunity triggered by viral infection (Table 2), and (c) neuromuscular degenerative disorders which are associated with viral infections (Table 3) due to unclear mechanisms, or as part of a multifactorial etiology. This review discusses neuromuscular complications of viral infection broadly, with emphasis on SARS-CoV-2 because of its novelty.
Neuromuscular Disorders as a Direct Consequence of Viral Infection
Neuromuscular dysfunction may arise due to viral infections, from a variety of mechanisms which are not always well understood (Table 1). Viruses may directly infect cellular components of the central and peripheral nervous systems, causing injury in the process, or due to toxicity by viral proteins [ex.gp120 produced during HIV infection causing direct toxicity to neural cells (54)]. Neuronal retrograde viral dissemination occurs when infection of neurons occurs in the periphery and the virus co-opts cellular transport machinery to access the nervous system (55). Damage to the peripheral nervous system may also be a collateral injury from the innate immune response (56), although distinguishing direct viral injury from such collateral injury can be difficult. Certain viral infections (particularly herpesviruses) may remain dormant within neurons for years or decades (55).
Complications to muscle due to viral infection can occur indirectly as a downstream effect of peripheral nerve dysfunction, or as a direct effect of viral infection resulting in myositis (57). The precise mechanisms by which myositis develops are also unclear; certain viruses [paramyxoviruses, enteroviruses, and picornaviruses, among others (14, 58)] are known to be associated with myositis, presumably due to direct infection of muscle tissues (15). However, certain viruses are also thought to be involved in the pathogenesis of autoimmune myositis, without requiring direct or acute infection of muscle tissues (59).
It is also important to mention that severe systemic involvement due to viral infection may result in direct consequences to the neuromuscular system. When viruses result in critical illness and prolonged hospitalization, patients are at high risk of developing critical illness neuropathy and/or myopathy (60), and the longer-term consequences of muscle deconditioning and accelerated sarcopaenia (61). Organ dysfunction due to viral infection can also result in consequences to the nerves and/or muscles, such as when the renal (62), hepatic (63), or endocrine (64) systems become impaired.
Neuromuscular Dysfunction Caused by Autoimmunity Triggered by Viral Infection
Viruses are an important cause of autoimmunity which can result in a wide spectrum of human diseases (65). Generally speaking, the mechanisms by which viral infection results in autoimmunity can include the following: (a) molecular mimicry, in which viral antigens similar to nervous system antigens provoke an autoimmune response to nervous tissues (66), (b) bystander activation, in which autoreactive T-cells respond to self antigens released from nonspecific tissue damage (67), and (c) epitope spreading, in which persistent infection results in progressive release of additional self antigens and diversification of self-epitopes that provoke an autoimmune response (68).
The classic neuromuscular complication from viral infection is Guillain Barré syndrome (GBS), either acute inflammatory demyelinating polyneuropathy (AIDP) or axonal variants thereof. This is also the group of conditions for which molecular mechanisms are best understood. Molecular mimicry was initially implicated in GBS based on studies of lipopolysaccharides from Campylobacter jejuni (C jejuni) that have molecular similarity to nerve sheath gangliosides (69). Further research demonstrated a group of antibodies autoreactive to gangliosides, associated with differing phenotypes of GBS (70). However, non-ganglioside antibodies have also been associated with GBS indicating a diversity of peripheral nerve proteins susceptible to autoimmunity due to presumed molecular mimicry (71).
Clinically, GBS is characterized by progressive and symmetrical weakness, with reduced myotatic reflexes (72). Approximately 50–70% of cases are preceded by respiratory or gastrointestinal infection within 1–2 weeks (73). C jejuni, influenza A, influenza B and hepatitis A serve as common preceding infection of GBS development, although there is substantial regional variation (18). Molecular mimicry is believed to be the primary mechanism for autoimmunity; C. jejuni has a ganglioside-like lipo-oligosaccharides (LOS) that accounts for the pathogenesis of axonal GBS (18). These axonal variants of AIDP, particularly acute motor axonal neuropathy (AMAN), may present with severe weakness and poor prognosis compared to demyelinating variants (32). However, AMAN may also be difficult to distinguish from AIDP and can have rapid reversibility of neurophysiologic findings in some cases, which can correlate to favorable prognosis (74, 75). A lengthy list of viruses is associated with GBS and these, as well as other autoimmune complications from viral infection are summarized in Table 2.
Chronic inflammatory demyelinating polyneuropathy (CIDP) results in relapsing disease which can be progressive and result in proximal or distal weakness (31). In its initial presentation, CIDP may present acutely, resembling acute inflammatory demyelinating polyneuropathy (or GBS) (31). CIDP may also occur following gastrointestinal or upper-respiratory infections (31).
Other conditions that have a possible autoimmune etiology include idiopathic brachial neuritis (also known as brachial amyotrophy or Parsonage Turner syndrome) characterized by pain and monoplegia (with occasional bilateral involvement). This condition is typically of unclear etiology, but has been associated with a wide range of possible underlying conditions, including various viral infections or vaccination (76). Post-infectious reactive myositis (also known as post-viral myositis) is typically described in children following influenza, and usually involves mild, self-limiting gastrocnemius weakness and pain (77).
Neuromuscular Degenerative Conditions Associated with Viral Infection
Most of the discoveries connecting viral infections to chronic degenerative neuromuscular disorders is focused on ALS, a disease characterized by the degeneration of upper and lower motor neurons resulting in progressive paralysis (78). ALS is the most common motor neuron disease and is fatal, usually within 2–5 years of symptom onset (79). Half of patients (50%) diagnosed with ALS die within 30 months of symptom onset usually due to respiratory dysfunction, but 10% have been known to survive for more than a decade (79). Approximately 15% patients with ALS are diagnosed with frontotemporal dementia (FTD), and the two diseases can overlap pathologically and genetically (79). Based on European populations, up to 20% of patients with ALS were found to have a familial form (78). ALS incidence rates show regional variation for reasons that are not clear but could be related to environmental factors (78). There is no cure for ALS, nor has the pathogenesis been fully elucidated.
Neurodegenerative diseases like ALS can have a multifactorial component in their pathogenesis (80). The etiology of ALS may depend on several risk factors, including viruses. Viruses are considered environmental risk factors and therefore are also possible risk factors in ALS pathogenesis (79). Although precise causative mechanisms have not been defined, there are reported associations with several viral infections (Table 3), including enteroviruses and retroviruses.
Discussion of Specific Viruses and Their Neuromuscular Complications
Both RNA and DNA viruses are known to elicit neuromuscular deficits. Many of these viruses share common pathological strategies to manipulate cellular function and promote neurological changes, such as viral protein neurotoxicity, induction of inflammation, autoimmunity, and modulation of cell signaling pathways (81–83). Below, viruses within select viral family groupings are discussed in detail as to their clinical presentation and association with neuromuscular disturbances. These associations are also schematically represented in Figure 1.
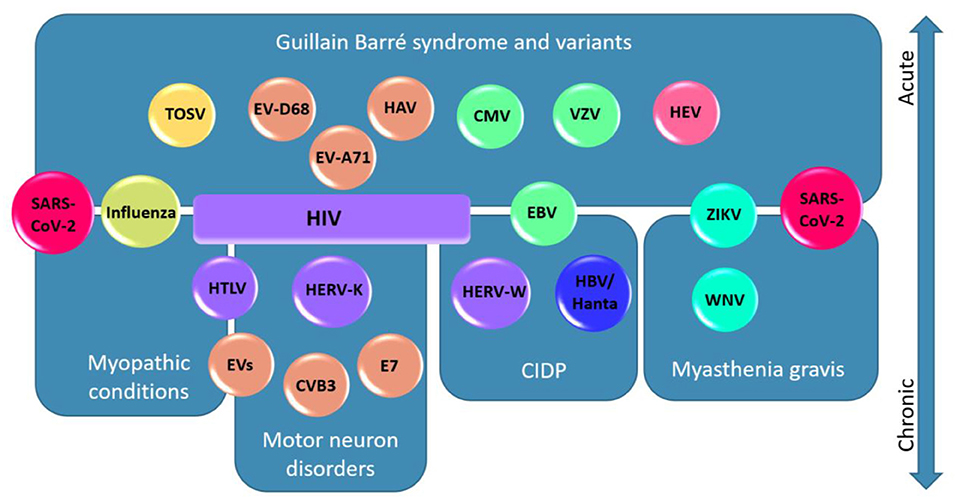
Figure 1. Viruses associated to their neuromuscular diseases and localisations. In this schematic diagram, viruses are represented according to the different diseases and neuromuscular localisations to which they are associated. Acute presentations are presented in the upper half of the figure and chronic disorders are presented in the lower half. Viruses are color-coded based on their family as follows: Coronaviridae (red), Orthomyxoviridae (yellow-green), Flaviviridae (cyan), Phenuiviridae (yellow), Picornaviridae (orange), Hantaviridae (blue), Hepeviridae (pink), Herpesviridae (green), and Retroviridae (purple). Note a general segregation of the viruses to the associated diseases/localisations. Note that SARS-CoV-2 is represented twice because of its association with GBS and variants, myasthenia gravis, and myopathic conditions. HIV is associated with a broad spectrum of neurological disorders and is therefore represented as a box spanning four disease categories. Abbreviations: Severe acute respiratory syndrome coronavirus 2 (SARS-CoV-2); Influenza A and B Virus (influenza); West Nile Virus (WNV); Zika Virus (ZIKV); Toscana virus (TOSV); Enterovirus (EV); Enterovirus D68 (EV-D68); Enterovirus A71 (EV-A71); Coxsackievirus B3 (CVB3); Echovirus-7 (E7); Hepatitis A Virus (HAV); Hepatitis E Virus (HEV); Hantavirus (Hanta); Hepatitis B virus (HBV); Varicella zoster virus (VZV); Epstein-Barr Virus (EBV); Cytomegalovirus (CMV); Human Immunodeficiency Virus (HIV), Human T-lymphotropic Virus (HTLV); Human Endogenous Retrovirus-W (HERV-W); Human Endogenous Retrovirus-K (HERV-K).
Coronaviridae
SARS-CoV-2
Direct Neuromuscular Complications
SARS-CoV-2 is associated with direct involvement of both peripheral nerves and muscles (Figure 2). One peripheral nerve complication that is widely discussed in popular media is anosmia and dysgeusia. The prevailing theory regarding the mechanism for this relates to infection and inflammation in the olfactory epithelium, which highly expresses ACE2 [the receptor for SARS-CoV-2 infection (84)]. In contrast, olfactory neurones do not appear to express ACE2 (85), although it has been proposed that olfactory sensory neurones are the route of entry to the central nervous system (86). It is important to clarify that anosmia and dysgeusia are general complications from upper respiratory tract infections and the uniqueness of this clinical association with COVID-19 is still debated (87). Nonetheless, the anosmia and dysgeusia from SARS-CoV-2 infection are considered to be unique given the abrupt onset and recovery, and the molecular mechanisms for this are an area of continued investigation [recently reviewed (88)].
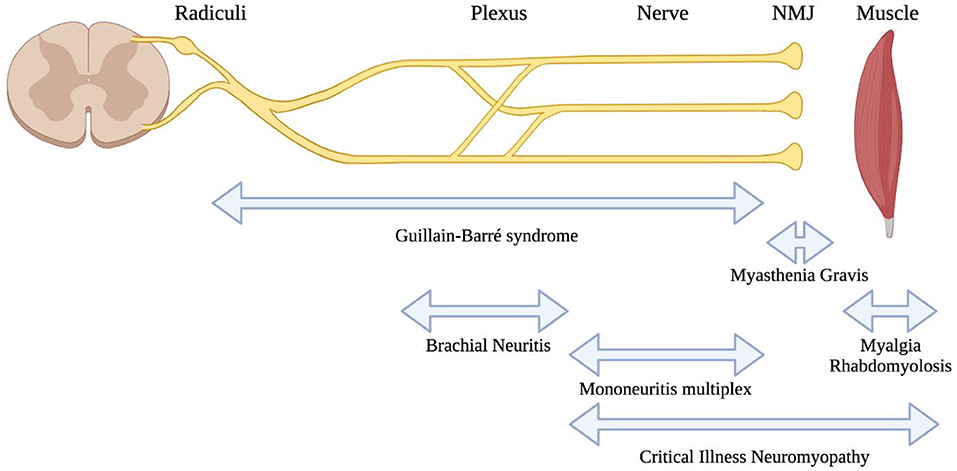
Figure 2. Localisations for neuromuscular consequences of SARS-CoV-2 infection. A highly schematic representation of the peripheral nervous system, showing major anatomic structures from the spinal cord through to muscle. Neuromuscular conditions associated with SARS-CoV-2 are listed below, with bidirectional arrows showing the approximate anatomic localisations of pathology.
Muscle involvement in SARS-CoV-2 is well-described and appears to be very common in COVID-19 patients. This usually manifests as myalgias with elevations in creatine kinase enzyme levels (89). Severe muscle involvement with rhabdomyolysis is rarely associated with SARS-CoV-2, but the mechanism by which this occurs (direct viral infection or a consequence of the systemic inflammatory response) is not clear (5, 90). One study of muscle tissues from patients who died from COVID-19 pointed toward an immune-mediated pathogenesis for myopathy and cardiomyopathy, rather than direct infection of muscle tissues (91). This also appears to be supported by clinical presentations similar to dermatomyositis in some cases (92). Occasionally, rhabdomyolysis was the presenting clinical feature in advance of pulmonary symptoms from COVID-19 (93, 94).
Because SARS-CoV-2 may cause acute and severe illness requiring intensive care, these severe cases are also vulnerable to above-mentioned systemic complications including critical illness neuromyopathy, or downstream neuromuscular effects due to other end-organ injury or deconditioning. The use of dexamethasone for treatment of patients with severe disease results in overall better outcomes (95), although there is ongoing debate about whether corticosteroids may influence the development of critical illness neuromyopathy (96, 97). To our knowledge this has not been studied in the COVID-19 context.
Autoimmune Neuromuscular Complications
Guillain-Barré Syndrome (GBS) and its variants appear to be the most commonly described autoimmune neuromuscular complication from SARS-CoV-2 infection (98). The first reported case of GBS associated with SARS-CoV-2 infection appeared to suggest a parainfectious development of the condition (99). Since then, numerous additional reports have been published and recently reviewed (100). In general, GBS associated with COVID-19 occurs as a post-infectious condition, usually featuring classic clinical presentations of GBS. There is a broad age range of affected individuals and it can also occur in patients with asymptomatic infection (101). Phenotypic variations from the typical GBS presentations are also described after SARS-CoV-2 infection, such as isolated facial diplegia (102–104). Despite the many reports, a recent large epidemiologic study showed no association between COVID-19 and GBS; in fact, GBS incidence appeared to have been reduced during the pandemic, perhaps due to public health measures that have reduced the transmission of other infections (105). There are several reasons that an association may be challenging to detect, such as the difficulties in identifying people who could have had GBS following asymptomatic or paucisymptomatic COVID-19, and on the other end of the disease spectrum, identifying GBS in patients who expired or had prolonged critical care admission for severe COVID-19 (106). Future study will hopefully clarify if, or to what extent an association exists.
A single study demonstrated the finding of mononeuritis multiplex (MNM) in patients with severe SARS-CoV-2 infection (2) requiring critical care. Their nerve injuries were suspected to develop during the height of their illness, due to the recognition of their neuropathies following withdrawal of sedation. Proning and/or patient handling injuries were thought to be unlikely (2), although a separate study suggested that prone positioning may be associated with more frequent and atypical compressive neuropathies (107). The postulated mechanism was parainfectious vasculitis as part of the cytokine storm that has been observed from COVID-19 (108). This still awaits confirmation from independent study, although as the authors point out, MNM is very difficult to recognize in patients with critical illness, when findings of weakness are likely to be attributed to critical illness neuro/myopathy. We are aware of additional isolated cases of MNM that have been reported (109, 110).
Brachial neuritis is also described in association with SARS-CoV-2, in isolated case reports (3, 111, 112).
Overall, the recognition of neurologic complications of COVID-19 is highly important because they are associated with poor prognosis (113). There may yet be additional neuromuscular complications from COVID-19 which are unrecognized; for example, case reports suggest the possible association of COVID-19 in three patients with a new diagnosis of myasthenia gravis (17, 114). Further study is required to confirm whether associations from case reports are truly related to SARS-CoV-2 or are coincidental.
Chronic Post-COVID Syndrome (Long COVID) and Neuromuscular Complications
Infection from SARS-CoV-2 is increasingly recognized to produce long-term consequences in some patients, referred to by several names including the chronic post-COVID syndrome, post-acute sequelae of COVID-19 (PASC) syndrome, or popularly, “long COVID”. Symptoms that potentially implicate the neuromuscular system are common in long COVID, such as muscle weakness and fatigue, sensory disturbances, and symptoms of autonomic dysfunction (115). Small fiber neuropathy has been demonstrated in long COVID syndrome, using clinical and neurophysiologic evaluation (116) as well as pathologic confirmation (117). Myopathic changes are apparent using quantitative EMG in long COVID patients having fatigue symptoms (118). Autonomic dysfunction, primarily orthostatic intolerance, has also been shown with autonomic testing (119). This may also manifest as the postural orthostatic tachycardia syndrome (POTS) in some patients (120).
The mechanisms causing long COVID are unknown, but autoimmune processes or persistent uncleared viral material have been postulated, among others (121). Recently, evidence suggests that reactivation of Epstein-Barr virus, among other factors, appears to be associated with long COVID (122). Additional research will be required to understand this possible interaction and may begin to show added complexity to viral infections' impacts on human health.
COVID-19 and Patients with Pre-existing Neuromuscular Conditions
The COVID-19 pandemic has also brought attention to the added risk that patients with pre-existing neuromuscular disease may experience if they are exposed to SARS-CoV-2. This can be due to characteristics of the disease itself [e.g., causing pre-existing respiratory muscle weakness (123)]. Other aspects associated with having a neuromuscular disease may increase risk of exposure, such as carers entering the home and lack of accessibility to public health services (124). Several neuromuscular diseases are treated with immunosuppression which appears to be associated with higher risk of hospitalization and mortality due to SARS-CoV-2 (125).
Evidence suggests that patients with pre-existing neurological conditions have more severe outcomes from COVID-19 (113). Patients with neuromuscular disease may experience respiratory muscle weakness resulting in reduced airway clearance (123) or bulbar dysfunction with potential aspiration (126) which can result in higher risk of pulmonary infections, and lower resilience in the case of a comorbid respiratory infection such as SARS-CoV-2. Cardiac disease is also a common comorbidity with neuromuscular disorders that increases the likelihood of poor outcome from COVID-19 (127). Although specific evidence to show the extent of the added risk is still not available, there is clearly expert consensus that SARS-CoV-2 infection is likely to pose substantial added risk to neuromuscular patients (128). This has resulted in advocacy efforts for the prioritization of neuromuscular disease patients for vaccination (129). Patients with chronic disabling conditions may also be deprioritised for life-saving care in situations where critical care resources become limited (130, 131).
Living with a chronic neuromuscular disease also increases the risk of exposure to pandemic illness if caregiver support is required in the home (124). Patients with neuromuscular conditions may have reduced mobility and limited transport options, which may result in reduced access to public health services (132). Conversely, the pandemic has provided an opportunity to build virtual care programs to improve access and ease of care for patients with debilitating neuromuscular conditions. Telemedicine was an active area of research in certain neuromuscular conditions such as amyotrophic lateral sclerosis (ALS) even prior to the COVID pandemic (133), and patients/caregivers had identified the need for travel as the major barrier to ALS clinic attendance (134). Recent guidelines advocate for consideration of telehealth options for the care of ALS (135) and during the pandemic virtual care has been shown to be effective and well received (136).
We are aware of two reports suggesting that CIDP may be exacerbated by SARS-CoV-2 infection (137, 138). In both cases, the exacerbations were uncharacteristically severe in comparison to the patients' prior disease course: the former case had cranial nerve involvement and tetraparesis (137), and the latter having respiratory failure and cytoalbuminologic disassociation in CSF (138).
Autoimmune neuromuscular conditions such as myasthenia gravis are often treated with immune-suppressive medications. Evidence is conflicting as to whether immunosuppressive drugs increase susceptibility to severe outcomes due to COVID-19, although another recent cohort of immunosuppressed patients had higher likelihood of in-hospital complications and death (139). There is still no substantial evidence to address this issue, specifically for neuromuscular patients. Preliminarily, there is some suggestion that patients with myasthenia gravis may have more severe outcomes (140); however, it is challenging to determine the extent to which the disease itself or its therapies may contribute to this. In principle, there could also be concern that immune-suppression may reduce the efficacy of vaccination, although the limited evidence thus far suggests antibodies are detectable post-vaccination in immunocompromised patients, which is indicative of some degree of immunity (141). Evidence from other autoimmune disorders has led to general recommendations regarding the timing of vaccinations related to various immune-suppressive agents (142). Immune-suppressive agents can result in reduced antibody titres from vaccination (143), which appears to correlate with reduced immunity in some populations (144).
Vaccination Against SARS-CoV-2 and Neuromuscular Complications
An additional question of recent interest relates to whether vaccines against SARS-CoV-2 are associated with a heightened risk of GBS. There is controversy regarding whether vaccines in general, such as the seasonal influenza shot, may be associated with GBS (145). There have been a few case reports indicating GBS occurring in temporal relationship to SARS-CoV-2 vaccination (146–148), with general emphasis on the fact that current evidence does not support a causal link (146, 148). Some of the reported cases include patients who may have predisposing factors, such as a prior history of GBS following vaccination (149), although a cohort study of patients with prior GBS who received mRNA vaccination did not show any major complications and argues strongly against any major risk (150). Another report of two patients with remission from diffuse large B-cell lymphoma who developed GBS after vaccination with tozinameran suggesting that aberrant B-cell function may be relevant to risk of GBS (151). This remains an evolving area of research and recently there appears to be an association possible for the adenovirus-based SARS-CoV-2 vaccinations with GBS (152).
CIDP is also reported as a possible consequence of vaccination, again with the majority of cases associated with adenovirus-based products (153–155). Brachial neuritis is also described as a post-vaccination complication in several reports (156–158), including patients receiving mRNA vaccines and usually ipsilateral to the side of vaccination (159). Inflammatory myopathy has also been reported in isolated cases as a side effect of vaccination, causing syndromes resembling poly/dermatomyositis (160) or localized myositis near the site of inoculation (161), There are also case reports of SARS-CoV-2 vaccine associated with varicella zoster reactivation (162), although at present a relationship is not clearly established (163).
Orthomyxoviridae
Influenza A and B Virus
The influenza pandemic (Spanish influenza), caused by a virulent strain of H1N1 of influenza A, affected 25–45% of the world's population, resulting in tens of millions of deaths (19). A study recruited 150 GBS patients and showed that 16% (24/150) of them displayed a positive serology for influenza B and 17% (26/150) had a positive serology for influenza A (18). Seven patients with preceding influenza B infection had a pure motor GBS without sensory deficits (18). This study (18) reported influenza B-related GBS as a pure motor phenotype. Molecular mimicry mechanism is a likely model in post-infectious GBS, however data that supports this hypothesis is sparse (19). Foreign and self-peptides share a common epitope; individuals carrying an MHC allele recognizing the target may activate autoreactive B cell or T cells through recognition of the foreign antigen, leading to autoimmunity (164).
According to one large retrospective study, 80% of cases of viral myositis were attributed to influenza, particularly influenza B (11). Influenza-associated myositis more commonly affects males aged 5–9, with calf muscle involvement and moderate elevations in creatine kinase. The condition is typically benign and resolves spontaneously, and it is likely to be under-recognized (165). Postulated mechanisms include the possibility of direct viral infection of myocytes (166), or perhaps as a post-infectious immune-mediated injury (167).
Flaviviridae
West Nile Virus (WNV)
WNV is a positive-stranded RNA virus of the Flaviridae family (168). WNV can spread to humans by mosquitoes. Over 80% of WNV infections are asymptomatic, however approximately 5% symptomatic cases have neurologic manifestations including meningitis, encephalitis, and acute flaccid myelitis (168). There have been 6 reports of patients with WNV infection that led to myasthenia gravis, which may also suggest that WNV disease is a trigger for developing autoimmunity (20).
Zika Virus (ZIKV)
A study in 2016 conducted during the Colombian outbreak of ZIKV identified a series of 68 GBS patients with symptoms of ZIKV infection (21). Seventeen (25%) of these patients remained positive for ZIKV by RT-PCR, including 3 of the patients with ZIKV in the CSF, and was compatible with prior observations in a case-control study (169). Both studies suggested that the mechanism for GBS in these patients may differ from classic descriptions of GBS in other infections; the time to onset of GBS from viral infections was shorter and presence of viraemia at time of neuromuscular symptoms suggests a parainfectious, rather than postinfectious etiology (21). The association of GBS with ZIKV was also supported by a population study showing significant elevation in hospitalisations for inflammatory neuropathies at the same time ZIKV became prevalent in Brazil (170).
Hepatitis C Virus (HCV)
HCV causes chronic infection resulting in ongoing liver inflammation with long term complications including fibrosis, cirrhosis and hepatocellular carcinoma (171). Cryoglobulinaemic vasculitis occurs due to immune complexes that are cold-precipitating and deposit on the vascular endothelium of end-organs, such as peripheral nerves (24). Cryoglobulins are associated with chronic HCV infection but may also occur in lymphoproliferative disorders or other chronic conditions (172, 173). The peripheral nerve complications of cryoglobulinaemic vasculitis most commonly cause painful sensory or sensorimotor polyneurophathy, but also includes mononeuropathy multiplex (23). There appears to be a female predominance in development of peripheral nerve complications (23), and the central nervous system may also become affected (173).
Phenuiviridae
Toscana Virus (TOSV)
TOSV originates in the Mediterranean area and is transmitted by sand flies (Phlebotomus perniciosus and Phlebotomus perfiliewi) in central Italy (174). While TOSV can affect the central nervous system (26, 174), TOSV IgG antibodies were found quite frequently in recently diagnosed GBS patients (26), suggesting that TOSV may trigger GBS. Similarly, a case report of a 40-year-old male had fit the diagnostic criteria for a subtype of GBS, acute motor and sensory axonal neuropathy (ASMAN) and tested positive for TOSV IgG antibodies as well (25).
Picornaviridae
Enterovirus D68 (EV-D68)
This positive-strand RNA virus primarily shares characteristics with rhinoviruses and is primarily associated with respiratory disease, most notably during outbreaks starting in 2014 (175). During these outbreaks it was observed that EV-D68 was rarely associated with acute flaccid myelitis (176). There is a possible reported association of EV-D68 with GBS from one series (27).
Enterovirus A71 (EV-A71)
EV-A71 infection is a cause of the childhood exanthem referred to as “hand foot mouth disease”. A small proportion of cases are associated with central nervous system involvement (177), but a few reported cases have additionally associated this virus with presentations of Guillain Barré Syndrome (178, 179).
Isolated reports also support the possibility that enteroviruses can be associated with myositis. One study identified enterovirus-specific genomic sequences in autoimmune polymyositis and dermatomyositis (29). A single case report identified myositis and rhabdomyolysis coincident with echovirus-9 infection (180). A possible etiologic link is supported by animal studies that have connected other enteroviruses with myositis (181). However the association remains unclear with other studies unable to show viral persistence (182).
Hepatitis A Virus (HAV)
GBS is rarely associated with HAV (28) but there have still been reports across the literature. In the study by Hao et al. (18), 7/150 (5%) GBS patients had a positive serology for hepatitis A. There was also a case report of as 12-year old boy who met the diagnostic criteria for GBS upon HAV infection (28). In this latter report, it was unclear whether GBS was post-infectious, or occurring in the context of ongoing liver inflammation (28). HAV could be a rare trigger for GBS that may occur simultaneously with progressive liver inflammation (28). It is possible that select pathogenic mechanisms are shared by both HAV and GBS (28).
Hepeviridae
Hepatitis E Virus (HEV)
The primary cause of hepatitis globally is HEV infection (30). There have been animal models where mice that were infected with HEV demonstrated the ability to infect neural tissue in the brain in vivo (30). HEV RNA has also been detected in CSF from some patients with HEV-associated GBS (30). Proposed mechanisms for GBS in HEV infection include either direct viral damage due to HEV replication in the neurological system and/or molecular mimicry (30). Although numerous case reports exist, only two prior studies report series of 10 or more cases (183, 184), suggesting HEV-associated GBS may be rare in endemic regions, or perhaps under-recognized and requires further study.
HEV has also rarely been associated with Parsonage Turner syndrome (PTS). Several of the reported cases have clear evidence of acute HEV infection (185–187). PTS associated with HEV appears to have a higher likelihood of bilateral presentation (13).
Hantaviridae
Hantavirus
In a single case report (31), a 44-year old male who was a hepatitis B virus (HBV) carrier, presented with acute quadriplegia, fever, malaise, anorexia, and subconjunctival hemorrhage. His serology suggested acute hantavirus infection. Nerve conduction studies (NCS) showed delayed latency in all 4 extremities (31). The patient showed polyclonal gammopathy with elevation of both IgG and IgA (71). The patient was diagnosed with GBS, however due to relapsing disease, the diagnosis was felt to be more consistent with CIDP (31). HBV and hantavirus co-infection was proposed to induce CIDP (31).
Herpesviridae
Herpes Simplex Virus 1 (HSV-1)
Bell palsy, which is the condition of unilateral paresis of cranial nerve VII, is a condition of unclear etiology. Certain Herpesviridae including HSV-1 (and HSV-2, below) are implicated in the pathogenesis of Bell palsy although various other causes are postulated (6).
Herpes Simplex Virus 2 (HSV-2)
HSV-2 infection is rarely associated with Elsberg syndrome, which is a presentation of acute lumbosacral plexitis, often accompanied by lower spinal cord myelitis (188). Elsberg syndrome may arise during either HSV-2 primary infection or during reactivation of latent infection (188). The condition may be under-recognized and may also be considered in unexplained cases of cauda equina syndrome (7). Criteria which may aid in recognition of this diagnostic entity have been proposed (7).
As mentioned above, HSV-2 is also considered to be associated with Bell palsy (6).
Varicella Zoster Virus (VZV, HHV-3)
VZV is also known as human alpha-herpesvirus 3 (HHV-3) and is most commonly associated with a febrile illness and macular-papular-vesicular rash during acute infection (varicella or “chicken pox”), as well as dermatomally-restricted eruptions during reactivation of dormant infection [zoster or “shingles (189)”]. Less commonly, varicella is associated with neurological complications which can include meningitis, encephalitis, stroke (due to vasculitis of cerebral vessels) and myelitis. Neuromuscular complications are common feature of VZV reactivation, in which patients may develop neuropathies of lower cranial nerves (10) [most commonly involving the facial nerve, referred to as Ramsay-Hunt syndrome (190, 191)]. VZV reactivation may also rarely resemble the clinical presentation of Elsberg syndrome (7). Post-herpetic neuralgia is a syndrome in which persistent neuropathic pain is present in the dermatome affected by a VZV reactivation (9). The spectrum of VZV reactivation syndromes may follow different patterns in the future as uptake of VZV vaccination continues (192).
GBS has also been associated with primary VZV infection. In one study, 1.6% of patients with varicella developed GBS within 4 weeks (32). It is speculated that VZV infection is associated with a pool of activated tissue-homing T-cells (32). CD4+ memory T cells are typically affected upon VZV infection (193). It is possible that this could lead to autoimmunity against peripheral nerves (32). However, it has also been suggested that VZV can directly infect the peripheral nerves which can result in peripheral nerve dysfunction (32).
Epstein-Barr Virus (EBV, HHV-4)
EBV is a highly prevalent DNA virus classically associated with mononucleosis, but is also associated with numerous human malignancies, and with non-malignant proliferative disorders in immune-compromised individuals (194). EBV is rarely associated with neuromuscular presentations including polyradiculitis [generally with coexistent central nervous system involvement (195)], as well as cranial neuropathy (196, 197), autonomic neuropathy (198), sensory neuropathy (199), and vasculitic neuropathy (200). These complications are generally only described in case reports and small series and proving their causation for such a widely prevalent infection is a challenge.
There may also be a connection between EBV infection and autoimmune neuromuscular disorders. Case reports connect EBV to GBS (201, 202) and variants such as Miller-Fisher syndrome (34, 203) and acute motor axonal neuropathy [AMAN (204)]. There is also a reported case of GBS with EBV and SARS-CoV-2 coinfection (205). There is also evidence suggesting that EBV is implicated in autoimmunity causing CIDP. A study including 66 CIDP participants showed that host-pathogen interactions were dysregulated for EBV, but not other herpesviruses, as evidenced by high seropositivity and threefold increased EBV cellular copy number compared with controls (33).
Cytomegalovirus (CMV, HHV-5)
CMV has high seroprevalence in the population, is well-adapted to the human immune system and typically does not produce symptoms, or occasionally results in mild illness resembling mononucleosis (206). Exceptions to this are in the case of congenital or neonatal infection, or in people who are immune-compromised due to HIV infection, organ or bone marrow transplants (207). In immunosuppressed adults, CMV is associated with the neurologic complication of polyradiculitis as an opportunistic infection (208).
Interestingly, immune-competent individuals with primary CMV infection may later develop GBS as a complication (209). A case-control study that enrolled 35 GBS cases found that in the 4 weeks preceding onset of GBS, 29 of the 35 patients experienced some form of infectious illness (35). Serologic testing identified 6 cases with IgM antibody against CMV, compared to 2 controls that showed the same result (35). Several other large studies confirm the strong association of primary CMV infection to GBS, including a 506 patient cohort study showing that CMV is a major cause of GBS comparable to C jejuni infection and present in 12.4% of cases (210). The pathogenesis may be explained by an association between anti-GM2 antibodies and previous CMV infection (211). IgM-type anti-GM2 antibodies, which are associated with motor neuron disease and acute and chronic polyneuropathies, are present in 30–50% of GBS patients who have had CMV infection (211).
Retroviridae
Human Immunodeficiency Virus (HIV)
Neurological diseases manifest during all stages of HIV infection, affecting the central and/or peripheral nervous systems (12). Neuromuscular complications from HIV may arise as a direct consequence of HIV infection, but can also be secondary to the acquired immunodeficiency syndrome (AIDS) (212), or as a consequence of antiretroviral therapy (ART) (213). HIV has been associated with complications at almost every possible neuromuscular localization, including lower motor neurons, polyradiculitis, polyneuropathy (including GBS and variants), autonomic neuropathy, mononeuropathy, mononeuritis multiplex, and myopathies (sporadic inclusion body myositis and sporadic late onset nemaline myopathy) (12, 42, 214).
Distal Symmetric Polyneuropathy (DSP) is the most common neurological complication resulting from HIV, affecting over 30% of HIV patients (215). DSP presents with symptoms including numbness, tightness, burning pain, paraesthesiae, and allodynia. The likelihood of developing DSP correlates with increased height, diabetes, substance abuse, hypertriglyceridemia, metabolic syndrome, increased age, and exposure to antiretroviral medications (12). The pathogenesis of this common complication may be related to dysregulation of macrophages and release of pro-inflammatory cytokines (TNF-α, IFN- α, IL-6), nitric oxide dysregulation, and free radical damage (12). Direct toxicity from the gp120 protein and an interaction with antiretrovirals may also be relevant to pathogenesis (216).
GBS or AIDP can rarely manifest in the initial stages (seroconversion) of HIV infection (12). Patients may present with symmetric acute ascending motor weakness, loss of reflexes, sensory symptoms and these symptoms usually precede with a flu or diarrheal illness (12). HIV patients with AIDP may have frequent episodes of AIDP and/or develop CIDP (12). Both AIDP and CIDP show elevated pleocytosis, meaning it is likely related to cerebrospinal fluid HIV viraemia (12). The immune response results in demyelinating the myelin sheath via autoantibodies and can affect multiple areas of the peripheral nervous system (12).
Mononeuritis multiplex (MNM) has been associated with HIV, in situations where AIDS occurs in conjunction with cytomegalovirus (CMV) reactivation (8). MNM is therefore unlikely to be present in patients who have access to ART. CMV reactivation can present with multi-organ involvement including retinitis, colitis, and oesophagitis (8). More extensive neurological involvement may also occur, manifesting as encephalitis, myelitis, and/or polyradiculopathy (8). Sensory ganglionopathy is also a possible presentation of HIV that has rarely also been associated with other viral etiologies (217).
Human Endogenous Retrovirus-W (HERV-W)
HERV-W encodes a pro-inflammatory protein named Multiple Sclerosis-associated RetroViral element Envelop protein (MSRV-Env) (36). One study investigated inflammatory neurological diseases for a study on Multiple Sclerosis and observed MSRV-Env expression (36). The study had 2 cohorts, part of which included 31 CIDP patients (36). In Study 1, 7/15 CIDP patients had significantly elevated levels, also known as a High Expression profile (HE), for MSRV transcript expression, majority (6/7) had a HE for MSRV-Env, and 1 had a HE for MSRV-pol (36). In Study 2, 6 CIDP patients presented with a HE for MSRV-Env and 8 CIDP patients presented a HE for MSRV-pol (36). There was also elevated Interleukin 6 (IL-6) and C-X-C Motif Chemokine Ligand 10 (CXCL10) levels in CIDP serum (36). Upregulation of IL-6 and CXCL10 is induced by MSRV-Env (36), which is consistent with the study's findings (165). CIDP autoimmune reaction may result from TLR4-driven activation of innate immunity by MSRV-Env (36). As observed in human Schwann cells (HSC), MSRV-Env expression within CIDP peripheral nerves may trigger inflammation along peripheral nerves mirrored by systemic immune dysregulation (36).
Discussion of Specific Viruses and Neuromuscular Degenerative Conditions
Picornaviridae
The picornavirus family has been investigated as a possible aetiologic agent in the development of ALS, given the presence of picornavirus RNA in central nervous system that is predominant in the anterior horns of the spinal cord (218).
Enteroviruses and ALS
Enteroviruses (EVs), including poliovirus, EV-D68, EV-A71, coxsackievirus B3 (CVB3), and echovirus-7 (Echo-7), tend to target several cell types in the CNS. They are able to infect motor neurons and cause the development of post-poliomyelitis-syndrome or non-polio flaccid paralysis (37). EV-D68 and EV-A71 are typically responsible for epidemics across the North American region and Asia-Pacific, respectively (219, 220). EV genomic material was detected in 60–88% of spinal cord/brain from ALS patients compared to 0–14% from controls (37). RT-PCR analysis of cerebrospinal fluid also showed detection of EV in 14.5% of 242 ALS patients vs. 7.6% in 354 controls, suggesting a link between EV infection and ALS development (37). EV infection is plausible to cause disease pathology via seeding of protein misfolding (37). An active viral infection is not necessarily required for disease progression because if EV infection occurs during this acute phase in childhood, it may still gradually propagate misfolded proteins in other regions of the CNS, eventually leading to ALS onset in adulthood (37).
Intriguingly, recent studies on CVB3 revealed that viral infection produces hallmark molecular and pathological phenotypes of ALS both in vitro and in vivo (38–40). CVB3 infection has been shown to cause translocation of TDP-43 to the cytoplasm, a pattern seen in other neurodegenerative diseases, through the action of virus-encoded protease that disrupts the nuclear pore complexes. CVB3 infection also results in the formation of stress granules (SGs), which co-localize with cytosolic TDP-43 aggregates (40). Cytoplasmic translocation and aggregation of TDP-43 is a hallmark for ALS (40, 41). In addition, CVB3 infection can cause direct neuronal damage and trigger robust inflammatory responses. Animal studies using SOD1-G85R ALS mice demonstrated that sublethal CVB3 infection that mimics chronic infection leads to early onset and accelerated ALS-like motor dysfunction, and shortened lifespan (39).
Echoviruses are part of the Enterovirus genus (41). In one study, they had positive results from a neutralization test for echo-7 of 40% among referents and 55% in ALS patients (221). The study chose to explore echo-7 because of the known ability of EVs to infect and destroy spinal and cortical motorneurons (41, 221). However the potential link to echoviruses should be studied further, with one prior study showing absence of echovirus RNA in CNS tissues of ALS patients (222).
Retroviridae
HIV and ALS-Like Syndromes
HIV appears to be capable of injuring the neuromuscular system with varying clinical presentations, localisations, and pathologic features. There have been over 20 case reports of HIV-associated motor neuron disease (12). Both HIV and human T-cell leukemia virus-1 (HTLV-1) have been associated with syndromes resembling ALS and respond to antiretroviral therapy (223, 224). Clinical observations have reported some ALS-like similarities and asymmetric patterns of extremity weakness, upper and lower motor neuron signs, fasciculations, atrophy, weight loss, easy fatigability, and brisk muscle jerk reflexes (12). HIV has also rarely been associated with motor neuron dysfunction causing bibrachial amyotrophic diplegia in case reports (42). HIV may lead to motor neuron disease by direct or indirect mechanisms of the virus (12). The virus can infiltrate the macrophages, microglia, and astrocytes, which affects neurons in the CNS (12).
Late-onset myopathy has also been associated with HIV infection, including cases of ocular myopathy presenting with ophthalmoplegia and/or ptosis (46, 225). Mitochondrial dyfunction was suggested as the underlying pathogenesis, based on the resemblance of this clinical presentation to chronic progressive external ophthalmoplegia (CPEO) (226). However, whether this relates to mitochondrial toxicity from HIV infection itself, and/or antiviral therapies is not known. Some of the reported cases had large-scale mtDNA deletions (46), so it is uncertain whether HIV and therapy unmasked or accelerated pre-existing disease, or was simply coincidental. Other rarely described late-onset myopathy presentations with HIV include sporadic inclusion body myositis (sIBM) and sporadic late-onset nemaline myopathy (SLONM) (42, 50). Described cases of sIBM suggest the age of onset may be earlier and with higher serum CK than HIV-negative sIBM (47). In the case of SLONM associated with HIV, cases appear to respond to immunosuppression emphasizing the importance of obtaining HIV serology in the workup of SLONM (45).
Human T-Lymphotropic Virus and ALS-Like Syndromes
In a study from 1995, 25 out of 50 sporadic ALS (sALS) patients showed immunoblot seroreactivity against HTLV-1/2 antigens (48). However, it is now recognized that most patients with sALS are HTLV-1 seronegative, although some HTLV-1 patients may have clinical presentations resembling motor neurone disease (49, 227), which probably is the reason for the finding in the above study. Mechanistically, HTLV has been associated with alterations in parathyroid hormone (PTH) regulation, which can result in motor neuron dysfunction (48). HTLV may also activate various human endogenous retroviruses (228) which may be implicated in neurodegenerative disease (see discussion below). Motor neuron disease can also be mimicked by lymphoma (a potential consequence of HTLV-1 infection) (48).
Sporadic inclusion body myositis has also been described from case reports in patients with evidence of HTLV-1 infection (50, 51). A series of patients from an endemic area in Japan appears to support sIBM as a true but uncommon association with chronic HTLV-1 infection (229).
Human Endogenous Retrovirus-K (HERV-K/ERVK) and ALS
Human endogenous retrovirus-K (HERV-K/ERVK) is a genomic symbiont in primates. Specifically, the HERV-K subgroup human mouse mammary tumor virus-like 2 (HML-2) is of particular interest because it is a recent integrant into the human genome and can become reactivated in several disease state, such as neurological disorders, autoimmune disorders and cancer (230).
For over a decade, HERV-K has been shown to have a neuropathological association with ALS (52). The reactivation of HERV-K is associated with upregulation of the RNA binding protein TDP-43, which is a defining neuropathologic feature in most cases of ALS (52, 53). This association has potential implications for biomarker development and therapy, and there are reports indirectly suggesting response to antiretroviral therapy in some ALS patients (223, 224, 231); further studies to confirm clinical significance will be required.
In comparison to healthy patients, HERV-K has been shown to have a significant elevated expression of its gag, pol, and env gene transcripts in the brain tissue of ALS patients (53). However, this is an evolving research area and recent studies indicate that experimentally assessing the relationship between HERV-K transcript levels in ALS may not be straightforward (232, 233). In contrast, the expression of HERV-K in brain and spinal cord is reliably measured at the protein level in approximately half of patients with sporadic ALS (52, 53, 224). A variety of HERV-K viral proteins can be measured at the protein level, including the viral envelope protein, conotoxin-like protein, reverse transcriptase, integrase and structural Gag proteins in specimens from patients with ALS. Moreover, a transgenic murine model of HERV-K driven motor neuron disease highlights that the production of viral proteins from the HERV-K env gene in neurons can lead to motor disturbances and reduced lifespan (53). It will be important for future studies to investigate how the neuropathological mechanisms behind HERV-K's association with ALS differ from that of other viruses, and whether appropriate antiviral drugs may be used therapeutically to treat motor disturbances in ALS.
Increased HERV-K protein maturation has also been observed in Spinal Bulbar Muscular Atrophy, which is an inherited form of motor neuron disease (234).
Discussion
This review presents a summary of neuromuscular complications associated with viral infections. The complications associated with SARS-CoV-2 infection have been emphasized because of its novelty. Of greatest interest is the possible relationship of GBS and variants to SARS-CoV-2. Anecdotally there are numerous reports of GBS in the literature temporally related to COVID-19, and it appears possible that atypical presentations such as facial diplegia may be more commonly associated with SARS-CoV-2. However, large epidemiologic studies have not clearly demonstrated an association, and paradoxically the incidence of GBS even appears to have been reduced by the pandemic (105). This reduction is likely because public health restrictions have been highly effective at reducing other infections that are strongly associated with GBS, such as C. jejuni and various respiratory viruses. A lack of association between SARS-CoV-2 and GBS is considered reasonable because SARS-CoV-2 epitopes are not known to have homology to tissues of the neuromuscular system (105).
Because SARS-CoV-2 is a novel illness, various unanswered questions remain. At time of writing there were new genetic variants with higher virulence that were increasing in prevalence (235). Whether this will affect the prevalence of direct or autoimmune neurologic complications from SARS-CoV-2 infection is unknown but vigilance by clinicians and health systems will be necessary.
As seen in Table 3, many viruses are associated with the longer-term development of neurodegenerative conditions. It is certainly too early to know whether any neurodegenerative long-term complications of COVID-19 exist, but it will be necessary to remain alert to this possibility. Already there is some evidence that SARS-CoV-2 has long-term effects on disparate organ systems (115), which is a general phenomenon also seen with other viral infections (236). The most common long-term symptoms (chronic post COVID syndrome, also known as “long COVID”) include muscle weakness and fatigue (115), although whether this is due to effects on the neuromuscular system, other organs, or other factors, is still unknown. Overall, the persistence of long-term symptoms appears to be associated with severe disease during acute infection requiring hospitalization (237, 238). In such patients, critical illness neuromyopathy may be present to varying degrees as a general consequence of critical care management (239). However, long-term symptoms are also described in patients without severe disease and understanding the full extent of this syndrome and its mechanisms will require extensive further study (240).
An important issue occurring in the context of the SARS-CoV-2 pandemic is the relationship of persons receiving immunosuppressive therapies and their risk of poor outcomes from COVID-19. As discussed in our article, several neuromuscular disorders are treated with immunosuppression (241, 242). Since such therapies can be associated with opportunistic infections and poor outcomes from other infectious agents (243) there was concern that severe outcomes would also occur from SARS-CoV-2. This question probably remains unanswered; neuromuscular patients were strongly encouraged to limit their risk of exposure (244), so case counts in neuromuscular patients may have remained disproportionately low as a result. Another possibility is that immunosuppression may not be a risk factor, or may even be protective, as has been suggested in systematic analysis (245).
Overall, the range of possible neuromuscular complications relating to viral infections is broad. Some viruses such as HIV have been broadly studied and have an array of possible consequences to the nervous system; others have been associated only with restricted phenotypes and may be limited solely to anecdotal observations. Our knowledge of neuromuscular or other organ system complications from viral infections is likely to be incomplete due to the challenges with connecting both common and uncommon infections with rare neuromuscular disorders. The interaction of viral infections with neuromuscular disorders is likely to be complex and influenced by environmental factors, host genetic factors, as well as the microbiome (246), among other factors that may not yet be discovered. The underlying molecular mechanisms are likely to be diverse and with complex inter-relationships. Future research in these areas will provide a better understanding of the pathogenesis of neuromuscular disorders and hopefully open new therapeutic targets.
Author Contributions
SJ was the primary author and performed the literature review. RK, TS, HL, KS, and RD revised the manuscript for intellectual content. TS, RD, and GP conceptualized and generated the Figures. GP authored the manuscript, conceptualized the project, and supervised the work. All authors approved the final version submitted for publication.
Funding
SJ is the recipient of an Alberta Innovates Health Solutions undergraduate studentship (2020).
Conflict of Interest
The authors declare that the research was conducted in the absence of any commercial or financial relationships that could be construed as a potential conflict of interest.
Publisher's Note
All claims expressed in this article are solely those of the authors and do not necessarily represent those of their affiliated organizations, or those of the publisher, the editors and the reviewers. Any product that may be evaluated in this article, or claim that may be made by its manufacturer, is not guaranteed or endorsed by the publisher.
Acknowledgments
Figure 2 was created using Biorender.com.
Abbreviations
CMV, cytomegalovirus; CVB3, Coxsackievirus B3; E7, echovirus-7; EBV, Epstein-Barr virus; EVs, enteroviruses; EV-A71, enterovirus 71; EV-D68, enterovirus 68; Hanta, Hantavirus; HAV, hepatitis A virus; HBV, hepatitis B virus; HEV, hepatitis E virus; HERV, human endogenous retrovirus; HIV, human immunodeficiency virus; SARS-CoV-2, Severe acute respiratory syndrome coronavirus 2; TOSV, Toscana virus; VZV, varicella zoster virus; WNV, West Nile virus; ZIKV, Zika virus.
References
1. Wu Y, Xu X, Chen Z, Duan J, Hashimoto K, Yang L, et al. Nervous system involvement after infection with COVID-19 and other coronaviruses. Brain Behav Immun. (2020) 87:18–22. doi: 10.1016/j.bbi.2020.03.031
2. Needham E, Newcombe V, Michell A, Thornton R, Grainger A, Anwar F, et al. Mononeuritis multiplex: an unexpectedly frequent feature of severe COVID-19. J Neurol. (2020) 268:2685–9. doi: 10.1007/s00415-020-10321-8
3. Mitry MA, Collins LK, Kazam JJ, Kaicker S, Kovanlikaya A. Parsonage-turner syndrome associated with SARS-CoV2 (COVID-19) infection. Clin Imaging. (2021) 72:8–10. doi: 10.1016/j.clinimag.2020.11.017
4. Zazzara MB, Modoni A, Bizzarro A, Lauria A, Ciciarello F, Pais C, et al. COVID-19 atypical Parsonage-Turner syndrome: a case report. BMC Neurol. (2022) 22:96.
5. Khosla SG, Nylen ES, Khosla R. Rhabdomyolysis in Patients Hospitalized With COVID-19 Infection: Five Case Series. J Investi Med High Impact Case Rep. (2020) 8:2324709620984603. doi: 10.1177/2324709620984603
6. Zhang W, Xu L, Luo T, Wu F, Zhao B, Li X. The etiology of Bell's palsy: a review. J Neurol. (2020) 267:1896–905. doi: 10.1007/s00415-019-09282-4
7. Savoldi F, Kaufmann TJ, Flanagan EP, Toledano M, Weinshenker BG. Elsberg syndrome: A rarely recognized cause of cauda equina syndrome and lower thoracic myelitis. Neurol Neuroimmunol Neuroinflam. (2017) 4:e355. doi: 10.1212/NXI.0000000000000355
8. Palma P, Costa A, Duro R, Neves N, Abreu C, Sarmento A. Mononeuritis multiplex: an uncommon neurological manifestation of cytomegalovirus reactivation in an HIV-infected patient. BMC Infect Dis. (2018) 18:554. doi: 10.1186/s12879-018-3501-2
9. Hadley GR, Gayle JA, Ripoll J, Jones MR, Argoff CE, Kaye RJ, et al. Post-herpetic Neuralgia: a Review. Curr Pain Headache Rep. (2016) 20:17. doi: 10.1007/s11916-016-0548-x
10. Niesvizky-Kogan I, Greca I, Gambhir HS. Varicella zoster presenting as cranial polyneuropathy. Am J Emerg Med. (2019) 37(3):564.e5–564.e6. doi: 10.1016/j.ajem.2018.12.025
11. Kerr J, Macartney K, Britton PN. Influenza-associated myositis: a single-centre, 5-year retrospective study. Eur J Pediatr. (2021) 180:577–84. doi: 10.1007/s00431-020-03835-w
12. Prior DE, Song N, Cohen JA. Neuromuscular diseases associated with human immunodeficiency virus infection. J Neurol Sci. (2018) 387:27–36. doi: 10.1016/j.jns.2018.01.016
13. Dartevel A, Colombe B, Bosseray A, Larrat S, Sarrot-Reynauld F, Belbezier A, et al. Hepatitis E and neuralgic amyotrophy: Five cases and review of literature. J Clin Virol. (2015) 69:156–64. doi: 10.1016/j.jcv.2015.06.091
14. Singh U, Scheld WM. Infectious etiologies of rhabdomyolysis: three case reports and review. Clin Infect Dis. (1996) 22:642–9. doi: 10.1093/clinids/22.4.642
15. Poels PJ, Ewals JA, Joosten EM, van Loon AM. Rhabdomyolysis associated with simultaneous Epstein-Barr virus infection and isolation of echovirus 6 from muscle: a dual infection. J Neurol Neurosurg Psychiatry. (1989) 52:412–4. doi: 10.1136/jnnp.52.3.412
16. Agosti E, Giorgianni A, D'Amore F, Vinacci G, Balbi S, Locatelli D. Is Guillain-Barrè syndrome triggered by SARS-CoV-2? Case report and literature review. Neurol Sci. (2021) 42:607–12. doi: 10.1007/s10072-020-04553-9
17. Muhammed L, Baheerathan A, Cao M, Leite MI, Viegas S. MuSK antibody–associated myasthenia gravis with SARS-CoV-2 infection: a case report. Ann Intern Med. (2021) 174:872–3. doi: 10.7326/L20-1298
18. Hao Y, Wang W, Jacobs BC, Qiao B, Chen M, Liu D, et al. Antecedent infections in Guillain-Barré syndrome: a single-center, prospective study. Ann Clin Transl Neurol. (2019) 6:2510–7. doi: 10.1002/acn3.50946
19. Lehmann HC, Hartung HP, Kieseier BC, Hughes RAC. Guillain-Barré syndrome after exposure to influenza virus. Lancet Infect Dis. (2010) 10:643–51. doi: 10.1016/S1473-3099(10)70140-7
20. Leis AA, Szatmary G, Ross MA, Stokic DS. West nile virus infection and myasthenia gravis. Muscle Nerve. (2014) 49:26–9. doi: 10.1002/mus.23869
21. Parra B, Lizarazo J, Jiménez-Arango JA, Zea-Vera AF, González-Manrique G, Vargas J, et al. Guillain-Barré syndrome associated with Zika virus infection in Colombia. New England J Med. (2016) 375:1513–23. doi: 10.1056/NEJMoa1605564
22. Molko N, Simon O, Guyon D, Biron A, Dupont-Rouzeyrol M, Gourinat AC. Zika virus infection and myasthenia gravis: report of 2 cases. Neurology. (2017) 88:1097–8. doi: 10.1212/WNL.0000000000003697
23. Gemignani F. Clinical spectrum of cryoglobulinaemic neuropathy. J Neurol, Neurosurg Psychiatry. (2005) 76:1410–4. doi: 10.1136/jnnp.2004.057620
24. Charles ED, Dustin LB. Hepatitis C virus-induced cryoglobulinemia. Kidney Int. (2009) 76:818–24. doi: 10.1038/ki.2009.247
25. Rota E, Morelli N, Immovilli P, de Mitri P, Guidetti D. Guillain-Barré-like axonal polyneuropathy associated with Toscana virus infection. Medicine. (2017) 96:e8081. doi: 10.1097/MD.0000000000008081
26. Okar SV, Bekircan-Kurt CE, Hacioglu S, Erdem-Özdamar S, Özkul A, Ergünay K. Toscana virus associated with Guillain–Barré syndrome: a case–control study. Acta Neurol Belg. (2021) 121:661–8. doi: 10.1007/s13760-020-01279-5
27. Williams CJ, Thomas RH, Pickersgill TP, Lyons M, Lowe G, Stiff RE, et al. Cluster of atypical adult Guillain-Barré syndrome temporally associated with neurological illness due to EV-D68 in children, South Wales, United Kingdom, October 2015 to January 2016. Eurosurveillance. (2016) 21:30119. doi: 10.2807/1560-7917.ES.2016.21.4.30119
28. Baltadzhiev I, Geneva I, Popivanova N. Guillain-barré syndrome in a child with ongoing viral hepatitis a. Iranian J Child Neurol. (2018) 12:133–8.
29. Yousef GE, Isenberg DA, Mowbray JF. Detection of enterovirus specific RNA sequences in muscle biopsy specimens from patients with adult onset myositis. Ann Rheum Dis. (1990) 49:310–5. doi: 10.1136/ard.49.5.310
30. Liu H, Ma Y. Hepatitis E virus-associated Guillain–Barre syndrome: Revision of the literature. Brain Behav. (2020) 10:e01496. doi: 10.1002/brb3.1496
31. Lim JY, Lim YH, Choi EH. Acute-onset chronic inflammatory demyelinating polyneuropathy in hantavirus and hepatitis B virus coinfection: a case report. Medicine (United States). (2016) 95:e5580. doi: 10.1097/MD.0000000000005580
32. Islam B, Islam Z, Geurtsvankessel CH, Jahan I, Endtz HP, Mohammad QD, et al. Guillain-Barré syndrome following varicella-zoster virus infection. Eur J Clin Microbiol Infect Dis. (2018) 37:511–8. doi: 10.1007/s10096-018-3199-5
33. Lünemann JD, Tackenberg B, Stein A, Wandinger KP, Oertel WH, Wagner HJ, et al. Dysregulated Epstein-Barr virus infection in patients with CIDP. J Neuroimmunol. (2010) 218:107–11. doi: 10.1016/j.jneuroim.2009.11.003
34. Communal C, Filleron A, Baron-Joly S, Salet R, Tran TA. Pediatric Miller Fisher Syndrome Complicating an Epstein–Barr Virus Infection. Pediatr Neurol. (2016) 63:73–5. doi: 10.1016/j.pediatrneurol.2016.06.018
35. Nafissi S, Vahabi Z, Ghahar MS, Amirzargar AA, Naderi S. The role of Cytomegalovirus, Haemophilus Influenzae and Epstein Barr Virus in Guillain Barre syndrome. Acta Med Iran. (2013) 51:372–6. doi: 10.1002/pmrj.12619
36. Faucard R, Madeira A, Gehin N, Authier FJ, Panaite PA, Lesage C, et al. Human endogenous retrovirus and neuroinflammation in chronic inflammatory demyelinating polyradiculoneuropathy. EBioMedicine. (2016) 6:190–8. doi: 10.1016/j.ebiom.2016.03.001
37. Xue YC, Feuer R, Cashman N, Luo H. Enteroviral infection: The forgotten link to amyotrophic lateral sclerosis? Front Mol Neurosci. (2018) 11:63. doi: 10.3389/fnmol.2018.00063
38. Xue YC, Ruller CM, Fung G, Mohamud Y, Deng H, Liu H, et al. Enteroviral Infection Leads to Transactive Response DNA-Binding Protein 43 Pathology in Vivo. Am J Pathol. (2018) 188:2853–62. doi: 10.1016/j.ajpath.2018.08.013
39. Xue YC, Liu H, Mohamud Y, Bahreyni A, Zhang J, Cashman NR, et al. Sublethal enteroviral infection exacerbates disease progression in an ALS mouse model. J Neuroinflammation. (2022) 19:16. doi: 10.1186/s12974-022-02380-7
40. Fung G, Shi J, Deng H, Hou J, Wang C, Hong A, et al. Cytoplasmic translocation, aggregation, and cleavage of TDP-43 by enteroviral proteases modulate viral pathogenesis. Cell Death Differ. (2015) 22:2087–97. doi: 10.1038/cdd.2015.58
41. Castanedo-Vazquez D, Bosque-Varela P, Sainz-Pelayo A, Riancho J. Infectious agents and amyotrophic lateral sclerosis: another piece of the puzzle of motor neuron degeneration. J Neurol. (2019) 266:27–36. doi: 10.1007/s00415-018-8919-3
42. Rowland LP. HIV-related neuromuscular diseases: nemaline myopathy, amyotrophic lateral sclerosis and bibrachial amyotrophic diplegia. Acta Myol. (2011) 30:29–31. Available online at: https://www.aafp.org/pubs/afp/issues/2003/0201/p519.html
43. Satin ZA, Bayat E. ALS-Like Disorder in Three HIV-positive patients: case series. Case Rep Neurol. (2021). 13:59–64. doi: 10.1159/000511203
44. Contreras-Galindo R, López P, Vélez R, Yamamura Y. HIV-1 infection increases the expression of human endogenous retroviruses type K (HERV-K) in vitro. AIDS Res Hum Retroviruses. (2007) 23:116–22. doi: 10.1089/aid.2006.0117
45. Schnitzler LJ, Schreckenbach T, Nadaj-Pakleza A, Stenzel W, Rushing EJ, van Damme P, et al. Sporadic late-onset nemaline myopathy: clinico-pathological characteristics and review of 76 cases. Orphanet J Rare Dis. (2017) 12:86. doi: 10.1186/s13023-017-0640-2
46. Pfeffer G, Cote HCF, Montaner JS Li CC, Jitratkosol M, Mezei MM. Ophthalmoplegia and ptosis. mitochondrial toxicity in patients receiving hiv therapy. Neurology. (2009) 73:71–2. doi: 10.1212/WNL.0b013e3181aae814
47. Couture P, Malfatti E, Morau G, Mathian A, Cohen-Aubart F, Nielly H, et al. Inclusion body myositis and human immunodeficiency virus type 1: A new case report and literature review. Neuromusc Diso. (2018) 28:334–8. doi: 10.1016/j.nmd.2018.01.005
48. Westarp ME, Ferrante P, Perron H, Bartmann P, Kornhuber HH. Sporadic ALS/MND: a global neurodegeneration with retroviral involvement? J Neurol Sci. (1995) 129:145–7. doi: 10.1016/0022-510X(95)00087-I
49. Gonzalez Trujillo F, Parra Cortes K, Álvarez Pareja Y, Onate J. Human T-cell lymphotropic virus type I associated with amyotrophic lateral sclerosis syndrome: immunopathological aspects and treatment options. Cureus. (2020) 12:e7531. doi: 10.7759/cureus.7531
50. Cupler EJ, Leon-Monzon M, Miller J, Semino-Mora C, Anderson TL, Dalakas MC. Inclusion body myositis in HIV-1 and HTLV-1 infected patients. Brain. (1996) 119:1887–93. doi: 10.1093/brain/119.6.1887
51. Ozden S, Gessain A, Gout O, Mikol J. Sporadic Inclusion Body Myositis in a Patient with Human T Cell Leukemia Virus Type 1-Associated Myelopathy. Clin Infect Dis. (2001) 32:510–4. doi: 10.1086/318506
52. Douville R, Liu J, Rothstein J, Nath A. Identification of active loci of a human endogenous retrovirus in neurons of patients with amyotrophic lateral sclerosis. Ann Neurol. (2011) 69:141–51. doi: 10.1002/ana.22149
53. Li W, Lee MH, Henderson L, Tyagi R, Bachani M, Steiner J, et al. Human endogenous retrovirus-K contributes to motor neuron disease. Sci Transl Med. (2015) 7:307ra153. doi: 10.1126/scitranslmed.aac8201
54. Kanmogne GD, Kennedy RC, Grammas P. HIV-1 gp120 proteins and gp160 peptides are toxic to brain endothelial cells and neurons: possible pathway for HIV entry into the brain and HIV-associated dementia. J Neuropathol Exper Neurol. (2002) 61:992–1000. doi: 10.1093/jnen/61.11.992
55. Kramer MF, Cook WJ, Roth FP, Zhu J, Holman H, Knipe DM, et al. Latent herpes simplex virus infection of sensory neurons alters neuronal gene expression. J Virol. (2003) 77:9533–41. doi: 10.1128/JVI.77.17.9533-9541.2003
56. Koyuncu OO, Hogue IB, Enquist LW. Virus infections in the nervous system. Cell Host Microbe. (2013) 13:379–93. doi: 10.1016/j.chom.2013.03.010
57. Crum-Cianflone NF. Bacterial, fungal, parasitic, and viral myositis. Clin Microbiol Rev. (2008) 21:473–94. doi: 10.1128/CMR.00001-08
59. Miller FW, Lamb JA, Schmidt J, Nagaraju K. Risk factors and disease mechanisms in myositis. Nat. Rev. Rheumatol. (2018) 14:255–68. doi: 10.1038/nrrheum.2018.48
60. Goodman BP, Boon AJ. Critical Illness Neuromyopathy. Phys Med Rehabil Clin N Am. (2008) 19:97–110. doi: 10.1016/j.pmr.2007.10.012
61. Welch CK, Hassan-Smith Z, A Greig C, M Lord J, A Jackson T. Acute sarcopenia secondary to hospitalisation - an emerging condition affecting older adults. Aging and Disease. (2018) 9:151–64. doi: 10.14336/AD.2017.0315
62. Arnold R, Issar T, Krishnan A, Pussell BA. Neurological complications in chronic kidney disease. JRSM Cardiov Dis. (2016) 5:2048004016677687. doi: 10.1177/2048004016677687
63. McDougall AJ, Davies L, McCaughan GW. Autonomic and peripheral neuropathy in endstage liver disease and following liver transplantation. Muscle Nerve. (2003) 28:595–600. doi: 10.1002/mus.10477
64. Douglas M. Neurology of endocrine disease. Clin Med. (2010) 10:387–90. doi: 10.7861/clinmedicine.10-4-387
65. Smatti MK, Cyprian FS, Nasrallah GK. al Thani AA, Almishal RO, Yassine HM. Viruses and autoimmunity: a review on the potential interaction and molecular mechanisms. Viruses. (2019) 11:762. doi: 10.3390/v11080762
66. Kim B, Kaistha SD, Rouse BT. Viruses and autoimmunity. Autoimmunity. (2006) 39:71–7. doi: 10.1080/08916930500484708
67. Pacheco Y, Acosta-Ampudia Y, Monsalve DM, Chang C, Gershwin ME, Anaya JM. Bystander activation and autoimmunity. J Autoimmun. (2019) 103:102301. doi: 10.1016/j.jaut.2019.06.012
68. Venkatesha SH, Durai M, Moudgil KD. Epitope spreading in autoimmune diseases. In: Infection and Autoimmunity. Baltimore, MD: Elsevier (2015). p. 45–68. doi: 10.1016/B978-0-444-63269-2.00003-9
69. Moran AP, Annuk H, Prendergast MM. Antibodies induced by ganglioside-mimicking Campylobacter jejuni lipooligosaccharides recognise epitopes at the nodes of Ranvier. J Neuroimmunol. (2005) 165:179–85. doi: 10.1016/j.jneuroim.2005.04.013
70. Yu RK, Usuki S, Ariga T. Ganglioside molecular mimicry and its pathological roles in guillain-barré syndrome and related diseases. Infect Immun. (2006) 74:6517–27. doi: 10.1128/IAI.00967-06
71. Yuki N, Tagawa Y, Handa S. Autoantibodies to peripheral nerve glycosphingolipids SPG, SLPG, and SGPG in Guillain–Barré syndrome and chronic inflammatory demyelinating polyneuropathy. J Neuroimmunol. (1996) 70:1–6. doi: 10.1016/S0165-5728(96)00042-2
72. Walling A, Dickson G. Guillain-Barré syndrome. Am Fam Physician. (2013) 87:191–7. doi: 10.1186/s12883-022-02622-4
73. Esposito S, Longo MR. Guillain–Barré syndrome. Autoimmun Rev. (2017) 16:96–101. doi: 10.1016/j.autrev.2016.09.022
74. Kokubun N, Nishibayashi M, Uncini A, Odaka M, Hirata K, Yuki N. Conduction block in acute motor axonal neuropathy. Brain. (2010) 133:2897–908. doi: 10.1093/brain/awq260
75. Peediackal S, Jose J, Gafoor VA, Smitha B. Reversible conduction failure in acute motor axonal neuropathy. Ann Indian Acad Neurol. (2014) 17:142–3. doi: 10.4103/0972-2327.128601
76. Feinberg JH, Radecki J. Parsonage-turner syndrome. HSS J. (2010) 6:199–205. doi: 10.1007/s11420-010-9176-x
77. Magee H, Goldman RD. Viral myositis in children. Can Fam Physician. (2017) 63:365–8. Available online at: https://acta.tums.ac.ir/index.php/acta/article/view/4428
78. Hardiman O, Al-Chalabi A, Chio A, Corr EM, Logroscino G, Robberecht W, et al. Amyotrophic lateral sclerosis. Nat Rev Dis Primers. (2017) 3:17071. doi: 10.1038/nrdp.2017.71
79. Ingre C, Roos PM, Piehl F, Kamel F, Fang F. Risk factors for amyotrophic lateral sclerosis. Clin Epidemiol. (2015) 7:181–93. doi: 10.2147/CLEP.S37505
80. Al-Chalabi A, Calvo A, Chio A, Colville S, Ellis CM, Hardiman O, et al. Analysis of amyotrophic lateral sclerosis as a multistep process: a population-based modelling study. Lancet Neurol. (2014) 13:1108–13. doi: 10.1016/S1474-4422(14)70219-4
81. Ayala-Nunez NV, Gaudin R, A. viral journey to the brain: current considerations and future developments. Evans MJ, editor PLOS Pathogens. (2020) 16:e1008434. doi: 10.1371/journal.ppat.1008434
82. Bohmwald K, Gálvez NMS, Ríos M, Kalergis AM. Neurologic alterations due to respiratory virus infections. Front Cell Neurosci. (2018) 12:386. doi: 10.3389/fncel.2018.00386
83. Wiley CA. Emergent Viral Infections of the CNS. J Neuropathol Exper Neurol. (2020) 79:823–42. doi: 10.1093/jnen/nlaa054
84. Davidson AM, Wysocki J, Batlle D. Interaction of SARS-CoV-2 and other coronavirus with ACE (Angiotensin-Converting Enzyme)-2 as their main receptor. Hypertension. (2020) 76:1339–49. doi: 10.1161/HYPERTENSIONAHA.120.15256
85. Brann DH, Tsukahara T, Weinreb C, Lipovsek M, van den Berge K, Gong B, et al. Non-neuronal expression of SARS-CoV-2 entry genes in the olfactory system suggests mechanisms underlying COVID-19-associated anosmia. Sci Adv. (2020) 6:eabc5801. doi: 10.1126/sciadv.abc5801
86. Meinhardt J, Radke J, Dittmayer C, Franz J, Thomas C, Mothes R, et al. Olfactory transmucosal SARS-CoV-2 invasion as a port of central nervous system entry in individuals with COVID-19. Nat Neurosci. (2021) 24:168–75. doi: 10.1101/2020.06.04.135012
87. Carrillo-Larco RM, Altez-Fernandez C. Anosmia and dysgeusia in COVID-19: a systematic review. Wellcome Open Res. (2020) 5:94. doi: 10.12688/wellcomeopenres.15917.1
88. Butowt R, von Bartheld CS. Anosmia in COVID-19: underlying mechanisms and assessment of an olfactory route to brain infection. Neuroscientist. (2021) 27:582–603. doi: 10.1177/1073858420956905
89. Pitscheider L, Karolyi M, Burkert FR, Helbok R, Wanschitz J, Horlings C, et al. Muscle involvement in SARS-CoV-2 infection. Eur J Neurol. (2020) 28:3411–7. doi: 10.1111/ene.14564
90. Singh B, Kaur P, Mechineni A, Maroules M. Rhabdomyolysis in COVID-19: report of four cases. Cureus. (2020) 12:e10686. doi: 10.7759/cureus.10686
91. Aschman T, Schneider J, Greuel S, Meinhardt J, Streit S, Goebel HH, et al. Association between SARS-CoV-2 infection and immune-mediated myopathy in patients who have died. JAMA Neurol. (2021) 78:948. doi: 10.1001/jamaneurol.2021.2004
92. Saud A, Naveen R, Aggarwal R, Gupta L. COVID-19 and myositis: what we know so far. Curr Rheumatol Rep. (2021) 23:63. doi: 10.1007/s11926-021-01023-9
93. Meegada S, Muppidi V, Wilkinson DC, Siddamreddy S, Katta SK. Coronavirus disease 2019-induced rhabdomyolysis. Cureus. (2020) 12:e10123. doi: 10.7759/cureus.10123
94. Chedid NR, Udit S, Solhjou Z, Patanwala MY, Sheridan AM, Barkoudah E. COVID-19 and rhabdomyolysis. J Gen Intern Med. (2020) 35:3087–90. doi: 10.1007/s11606-020-06039-y
95. RECOVERY Collaborative Group, Horby P, Lim WS, Emberson JR, Mafham M, Bell JL, et al. Dexamethasone in Hospitalized Patients with Covid-19. N Engl J Med. (2021) 384:693–704. doi: 10.1056/NEJMoa2021436
96. Yang T, Li Z, Jiang L, Xi X. Corticosteroid use and intensive care unit-acquired weakness: a systematic review and meta-analysis. Crit Care. (2018) 22:187. doi: 10.1186/s13054-018-2111-0
97. Hermans G, de Jonghe B, Bruyninckx F, van den Berghe G. Interventions for preventing critical illness polyneuropathy and critical illness myopathy. Cochrane Datab System Rev. (2014) 2014:CD006832. doi: 10.1002/14651858.CD006832.pub3
98. Jardim Vaz de. Mello L, Guimaraes Silva E, Oliveira Correa Rabelo G, Evaristo Leite M, Vieira NR, Bahadori M, et al. Neurologic compromise in COVID-19: a literature review. J Neurol Res. (2020) 10:164–72. doi: 10.14740/jnr619
99. Zhao Y, Zhao Z, Wang Y, Zhou Y, Ma Y, Zuo W. Single-cell RNA expression profiling of ACE2, the putative receptor of Wuhan 2019-nCov. bioRxiv. (2020). doi: 10.1101/2020.01.26.919985
100. Abu-Rumeileh S, Abdelhak A, Foschi M, Tumani H, Otto M. Guillain–Barré syndrome spectrum associated with COVID-19: an up-to-date systematic review of 73 cases. J Neurol. (2021) 268:1133–70. doi: 10.1007/s00415-020-10124-x
101. d'Orsi G, Sica S, Maiorano A, Melchionda D, Lalla A, Montemurro L, et al. Guillain-Barré syndrome as only manifestation of COVID-19 infection. Clin Neurol Neurosur. (2021) 207:106775. doi: 10.1016/j.clineuro.2021.106775
102. Chan JL, Ebadi H, Sarna JR. Guillain-Barré syndrome with facial diplegia related to SARS-CoV-2 infection. Canadian J Neurol Sci. (2020) 47:852–4. doi: 10.1017/cjn.2020.106
103. Juliao Caamaño DS, Alonso Beato R. Facial diplegia, a possible atypical variant of Guillain-Barré Syndrome as a rare neurological complication of SARS-CoV-2. J Clin Neurosci. (2020) 77:230–2. doi: 10.1016/j.jocn.2020.05.016
104. Hutchins KL, Jansen JH, Comer AD, Scheer RV, Zahn GS, Capps AE, et al. COVID-19–Associated Bifacial Weakness with Paresthesia Subtype of Guillain-Barré Syndrome. Am J Neuroradiol. (2020) 41:1707–11. doi: 10.3174/ajnr.A6654
105. Keddie S, Pakpoor J, Mousele C, Pipis M, Machado PM, Foster M, et al. Epidemiological and cohort study finds no association between COVID-19 and Guillain-Barré syndrome. Brain. (2021) 144:682–93.
106. Foschi M, D'Anna L, Abdelhak A, Mayer B, Tumani H, Otto M, et al. Ongoing challenges in unravelling the association between COVID-19 and Guillain-Barré syndrome. Brain. (2021) 144:e44–e44. doi: 10.1093/brain/awab069
107. Malik GR, Wolfe AR, Soriano R, Rydberg L, Wolfe LF, Deshmukh S, et al. Injury-prone: peripheral nerve injuries associated with prone positioning for COVID-19-related acute respiratory distress syndrome. Br J Anaesth. (2020) 125:e478–80. doi: 10.1016/j.bja.2020.08.045
108. Thepmankorn P, Bach J, Lasfar A, Zhao X, Souayah S, Chong ZZ, et al. Cytokine storm induced by SARS-CoV-2 infection: The spectrum of its neurological manifestations. Cytokine. (2021) 138:155404. doi: 10.1016/j.cyto.2020.155404
109. Enrique ER, Javier BH, Hernando R, Herney Andrés GP. Mononeuritis Multiplex Associated with Sars-Cov2-Covid-19 Infection: Case Report. Int J Neurol Neurotherapy. (2020) 7:102. doi: 10.23937/2378-3001/1410102
110. Assar S, Pournazari M, Soufivand P, Mohamadzadeh D, Sanaee S. Microscopic polyangiitis associated with coronavirus disease-2019 (COVID-19) infection in an elderly male. Egyptian Rheumatol. (2021) 43:225–8. doi: 10.1016/j.ejr.2021.03.001
111. Coll C, Tessier M, Vandendries C, Seror P. Neuralgic amyotrophy and COVID-19 infection: 2 cases of spinal accessory nerve palsy. Joint Bone Spine. (2021) 88:105196. doi: 10.1016/j.jbspin.2021.105196
112. Viatgé T, Noel-Savina E, Prévot G, Faviez G, Plat G, de Boissezon X, et al. [Parsonage-Turner syndrome following severe SARS-CoV-2 infection]. Rev Mal Respir. (2021) 38:853–8. doi: 10.1016/j.rmr.2021.06.004
113. Manji H, Carr AS, Brownlee WJ, Lunn MP. Neurology in the time of COVID-19. J Neurol Neurosurg Psychiatry. (2020) 91:568–70. doi: 10.1136/jnnp-2020-323414
114. Restivo DA, Centonze D, Alesina A, Marchese-Ragona R. Myasthenia Gravis Associated With SARS-CoV-2 Infection. Ann Intern Med. (2020) 173:1027–8. doi: 10.7326/L20-0845
115. Huang C, Huang L, Wang Y, Li X, Ren L, Gu X, et al. 6-month consequences of COVID-19 in patients discharged from hospital: a cohort study. Lancet. (2021) 397:220–32. doi: 10.1016/S0140-6736(20)32656-8
116. Oaklander AL, Mills AJ, Kelley M, Toran LS, Smith B, Dalakas MC, et al. Peripheral Neuropathy Evaluations of Patients With Prolonged Long COVID. Neurol Neuroimmunol Neuroinflam. (2022) 9:e1146. doi: 10.1212/NXI.0000000000001146
117. Abrams RMC, Simpson DM, Navis A, Jette N, Zhou L, Shin SC. Small fiber neuropathy associated with SARS-CoV-2 infection. Muscle Nerve. (2022) 65:440–3. doi: 10.1002/mus.27458
118. Agergaard J, Leth S, Pedersen TH, Harbo T, Blicher JU, Karlsson P, et al. Myopathic changes in patients with long-term fatigue after COVID-19. Clin Neurophysiol. (2021) 132:1974–81. doi: 10.1016/j.clinph.2021.04.009
119. Shouman K, Vanichkachorn G, Cheshire WP, Suarez MD, Shelly S, Lamotte GJ, et al. Autonomic dysfunction following COVID-19 infection: an early experience. Clin Auton Res. (2021) 31:385–94. doi: 10.1007/s10286-021-00803-8
120. Chadda KR, Blakey EE, Huang CLH, Jeevaratnam K. Long COVID-19 and postural orthostatic tachycardia syndrome- is dysautonomia to be blamed? Front Cardiovasc Med. (2022) 9:860198. doi: 10.3389/fcvm.2022.860198
121. Proal AD, VanElzakker MB. Long COVID or Post-acute Sequelae of COVID-19 (PASC): an overview of biological factors that may contribute to persistent symptoms. Front Microbiol. (2021) 12:698169. doi: 10.3389/fmicb.2021.698169
122. Su Y, Yuan D, Chen DG, Ng RH, Wang K, Choi J, et al. Multiple early factors anticipate post-acute COVID-19 sequelae. Cell. (2022) 185:881–895.e20. doi: 10.1016/j.cell.2022.01.014
123. Pfeffer G, Povitz M. Respiratory management of patients with neuromuscular disease: current perspectives. Degener Neurol Neuromuscul Dis. (2016) 6:111–8. doi: 10.2147/DNND.S87323
124. Kuper H, Banks LM, Bright T, Davey C, Shakespeare T. Disability-inclusive COVID-19 response: What it is, why it is important and what we can learn from the United Kingdom's response. Wellcome Open Res. (2020) 5:79. doi: 10.12688/wellcomeopenres.15833.1
125. Suárez-García I, Perales-Fraile I, González-García A, Muñoz-Blanco A, Manzano L, Fabregate M, et al. In-hospital mortality among immunosuppressed patients with COVID-19: analysis from a national cohort in Spain. PLoS ONE. (2021) 16:e0255524. doi: 10.1371/journal.pone.0255524
126. Jaradeh S. Muscle Disorders Affecting Oral and Pharyngeal Swallowing. GI Motility online. (2006).
127. Phelps M, Christensen DM, Gerds T, Fosbøl E, Torp-Pedersen C, Schou M, et al. Cardiovascular comorbidities as predictors for severe COVID-19 infection or death. Eur Heart J. (2021) 7:172–80. doi: 10.1093/ehjqcco/qcaa081
128. Neurologists A of B. Association of British Neurologists Guidance on COVID-19 for people with neurological conditions, their doctors and carers. (2020). Available online at: https://cdn.ymaws.com/www.theabn.org/resource/collection/65C334C7-30FA-45DB-93AA-74B3A3A20293/ABN_Neurology_COVID-19_Guidance_v5_26.3.20.pdf (accessed June 15, 2021).
129. Pfeffer G, Jacob S, Preston J. COVID-19 vaccine priority for people with neurologic and rare diseases. J Neurol Res. (2021) 11:1–4. doi: 10.14740/jnr665
130. Iezzoni LI, Rao SR, Ressalam J, Bolcic-Jankovic D, Agaronnik ND, Donelan K, et al. Physicians' perceptions of people with disability and their health care. Health Aff. (2021) 40:297–306. doi: 10.1377/hlthaff.2020.01452
131. Wilson C. Ontario patients to be ranked for life-saving care should ICUs become full, documents show. (2021). Available online at: https://toronto.ctvnews.ca/ontario-patients-to-be-ranked-for-life-saving-care-should-icus-become-full-documents-show-1.5271774 (accessed June 15, 2021).
132. Armitage R, Nellums LB. The COVID-19 response must be disability inclusive. Lancet Public Health. (2020) 5:e257. doi: 10.1016/S2468-2667(20)30076-1
133. Helleman J, Kruitwagen ET, van den Berg LH, Visser-Meily JMA, Beelen A. The current use of telehealth in ALS care and the barriers to and facilitators of implementation: a systematic review. Amyotrophic Lateral Sclerosis Frontotemporal Degeneration. (2020) 21:167–82. doi: 10.1080/21678421.2019.1706581
134. Schellenberg KL, Hansen G. Patient perspectives on transitioning to amyotrophic lateral sclerosis multidisciplinary clinics. J Multidiscip Healthc. (2018) 11:519–24. doi: 10.2147/JMDH.S177563
135. Shoesmith C, Abrahao A, Benstead T, Chum M, Dupre N, Izenberg A, et al. Canadian best practice recommendations for the management of amyotrophic lateral sclerosis. Can Med Assoc J. (2020) 192:E1453–68. doi: 10.1503/cmaj.191721
136. Vasta R, Moglia C, D'Ovidio F, di Pede F, de Mattei F, Cabras S, et al. Telemedicine for patients with amyotrophic lateral sclerosis during COVID-19 pandemic: an Italian ALS referral center experience. Amyotroph Lateral Scler Frontotemporal Degener. (2021) 22:308–11. doi: 10.1080/21678421.2020.1820043
137. Abu-Rumeileh S, Garibashvili T, Ruf W, Fangerau T, Kassubek J, Althaus K, et al. Exacerbation of chronic inflammatory demyelinating polyneuropathy in concomitance with COVID-19. J Neurol Sci. (2020) 418:117106. doi: 10.1016/j.jns.2020.117106
138. van Looy E, Veenker L, Steyaert A, Leenders J, Malfroid G, de Cauwer H. COVID-19-induced exacerbation of chronic inflammatory demyelinating polyneuropathy. J Neurol. (2021) 268:3129–31. doi: 10.1007/s00415-021-10417-9
139. Andersen KM, Mehta HB, Palamuttam N, Ford D, Garibaldi BT, Auwaerter PG, et al. Association between chronic use of immunosuppresive drugs and clinical outcomes from coronavirus disease 2019 (COVID-19) hospitalization: a retrospective cohort study in a large US health system. Clin Infect Dis. (2021) 73:e4124–30. doi: 10.1093/cid/ciaa1488
140. Muppidi S, Guptill JT, Jacob S, Li Y, Farrugia ME, Guidon AC, et al. COVID-19-associated risks and effects in myasthenia gravis (CARE-MG). Lancet Neurol. (2020) 19:970–1. doi: 10.1016/S1474-4422(20)30413-0
141. Squire J, Joshi A. Seroconversion after coronavirus disease 2019 vaccination in patients with immune deficiency. Ann Allergy Asthma Immunol. (2021) 127:383–4. doi: 10.1016/j.anai.2021.05.015
142. Morel J, Czitrom SG, Mallick A, Sellam J, Sibilia J. Vaccinations in adults with chronic inflammatory joint disease: Immunization schedule and recommendations for patients taking synthetic or biological disease-modifying antirheumatic drugs. Joint Bone Spine. (2016) 83:135–41. doi: 10.1016/j.jbspin.2015.08.008
143. Mahil SK, Bechman K, Raharja A, Domingo-Vila C, Baudry D, Brown MA, et al. The effect of methotrexate and targeted immunosuppression on humoral and cellular immune responses to the COVID-19 vaccine BNT162b2: a cohort study. Lancet Rheumatol. (2021) 3:e627–37. doi: 10.1016/S2665-9913(21)00212-5
144. Caillard S, Chavarot N, Bertrand D, Kamar N, Thaunat O, Moal V, et al. Occurrence of severe COVID-19 in vaccinated transplant patients. Kidney Int. (2021) 100:477–9. doi: 10.1016/j.kint.2021.05.011
145. Grave C, Boucheron P, Rudant J, Mikaeloff Y, Tubert-Bitter P, Escolano S, et al. Seasonal influenza vaccine and Guillain-Barré syndrome. Neurology. (2020) 94:e2168–79. doi: 10.1212/WNL.0000000000009180
146. Márquez Loza AM, Holroyd KB, Johnson SA, Pilgrim DM, Amato AA. Guillain-Barré syndrome in the placebo and active arms of a COVID-19 vaccine clinical trial. Neurology. (2021) 96:1052–4. doi: 10.1212/WNL.0000000000011881
147. Finsterer J. Exacerbating Guillain–Barré syndrome eight days after vector-based COVID-19 vaccination. Case Rep Infect Dis. (2021) 2021:3619131. doi: 10.1155/2021/3619131
148. Ogbebor O, Seth H, Min Z, Bhanot N. Guillain-Barré syndrome following the first dose of SARS-CoV-2 vaccine: a temporal occurrence, not a causal association. IDCases. (2021) 24:e01143. doi: 10.1016/j.idcr.2021.e01143
149. Ling L, Bagshaw SM, Villeneuve PM. Guillain–Barré syndrome after SARS-CoV-2 vaccination in a patient with previous vaccine-associated Guillain–Barré syndrome. Can Med Assoc J. (2021) 193:E1766–9. doi: 10.1503/cmaj.210947
150. Shapiro Ben David S, Potasman I, Rahamim-Cohen D. Rate of Recurrent Guillain-Barré Syndrome After mRNA COVID-19 Vaccine BNT162b2. JAMA Neurol. (2021) 78:1409. doi: 10.1001/jamaneurol.2021.3287
151. Chun JY, Park S, Jung J, Kim SH, Kim TS, Choi YJ, et al. Guillain-Barré syndrome after vaccination against COVID-19. Lancet Neurol. (2022) 21:117–9. doi: 10.1016/S1474-4422(21)00416-6
152. WHO. Statement of the WHO Global Advisory Committee on Vaccine Safety (GACVS) COVID-19 Subcommittee on Reports of Guillain-Barré Syndrome (GBS) Following Adenovirus Vector COVID-19 Vaccines. Geneva: WHO (2021). p. 26–8.
153. de Souza A, Oo WM, Giri P. Inflammatory demyelinating polyneuropathy after the ChAdOx1 nCoV-19 vaccine may follow a chronic course. J Neurol Sci. (2022) 436:120231. doi: 10.1016/j.jns.2022.120231
154. Bagella CF, Corda DG, Zara P, Elia AE, Ruiu E, Sechi E, et al. Chronic Inflammatory Demyelinating Polyneuropathy after ChAdOx1 nCoV-19 Vaccination. Vaccines (Basel). (2021) 9:1502. doi: 10.3390/vaccines9121502
155. Suri V, Pandey S, Singh J, Jena A. Acute-onset chronic inflammatory demyelinating polyneuropathy after COVID-19 infection and subsequent ChAdOx1 nCoV-19 vaccination. BMJ Case Rep. (2021) 14:e245816. doi: 10.1136/bcr-2021-245816
156. Sharma A, Gupta A. A Rare case of brachial plexus neuropraxia after COVID-19 vaccination. Cureus. (2022) 14:e21244. doi: 10.7759/cureus.21244
157. Queler SC, Towbin AJ, Milani C, Whang J, Sneag DB. Parsonage-turner syndrome following COVID-19 vaccination: MR neurography. Radiology. (2022) 302:84–7. doi: 10.1148/radiol.2021211374
158. Diaz-Segarra N, Edmond A, Gilbert C, Mckay O, Kloepping C, Yonclas P. Painless idiopathic neuralgic amyotrophy after COVID-19 vaccination: A case report. PM&R. (2021). Available online at: https://www.aafp.org/pubs/afp/issues/2013/0201/p191.html?utm_medium=email&utm_source=transaction. [Epub ahead of print].
159. Shields LBE, Iyer VG, Zhang YP, Burger JT, Shields CB. Parsonage-turner syndrome following COVID-19 vaccination: clinical and electromyographic findings in 6 patients. Case Rep Neurol. (2022) 14:58–67. doi: 10.1159/000521462
160. Vutipongsatorn K, Isaacs A, Farah Z. Inflammatory myopathy occurring shortly after severe acute respiratory syndrome coronavirus 2 vaccination: two case reports. J Med Case Rep. (2022) 16:57. doi: 10.1186/s13256-022-03266-1
161. Theodorou DJ, Theodorou SJ, Axiotis A, Gianniki M, Tsifetaki N. COVID-19 vaccine-related myositis. QJM Int J Med. (2021) 114:424–5. doi: 10.1093/qjmed/hcab043
162. Psichogiou M, Samarkos M, Mikos N, Hatzakis A. Reactivation of varicella zoster virus after vaccination for SARS-CoV-2. Vaccines (Basel). (2021) 9:572. doi: 10.3390/vaccines9060572
163. Diez-Domingo J, Parikh R, Bhavsar AB, Cisneros E, McCormick N, Lecrenier N. Can COVID-19 increase the risk of herpes zoster? A Narrative Review. Dermatol Ther (Heidelb). (2021) 11:1119–26. doi: 10.1007/s13555-021-00549-1
164. Rojas M, Restrepo-Jiménez P, Monsalve DM, Pacheco Y, Acosta-Ampudia Y, Ramírez-Santana C, et al. Molecular mimicry and autoimmunity. J Autoimmun. (2018) 95:100–23. doi: 10.1016/j.jaut.2018.10.012
165. Zafeiriou D, Katzos G, Gombakis N, Kontopoulos E, Tsantali C. Clinical features, laboratory findings and differential diagnosis of benign acute childhood myositis. Acta Paediatr. (2000) 89:1493–4. doi: 10.1111/j.1651-2227.2000.tb02783.x
166. Davis LE, Kornfeld M. Experimental influenza B viral myositis. J Neurol Sci. (2001) 187:61–7. doi: 10.1016/S0022-510X(01)00526-3
167. Davis LE. Neurologic and Muscular Complications of the 2009 Influenza A (H1N1) Pandemic. Curr Neurol Neurosci Rep. (2010) 10:476–83. doi: 10.1007/s11910-010-0135-1
168. Rossi SL, Ross TM, Evans JD. West Nile Virus. Clin Lab Med. (2010) 30:47–65. doi: 10.1016/j.cll.2009.10.006
169. Cao-Lormeau VM, Blake A, Mons S, Lastère S, Roche C, Vanhomwegen J, et al. Guillain-Barré Syndrome outbreak associated with Zika virus infection in French Polynesia: a case-control study. Lancet. (2016) 387:1531–9. doi: 10.1016/S0140-6736(16)00562-6
170. Angelo JR, Fuller TL, Leandro BBS, Praça HLF, Marques RD, Ferreira JMC, et al. Neurological complications associated with emerging viruses in Brazil. Int J Gynecol Obstetrics. (2020) 148:70–5. doi: 10.1002/ijgo.13050
171. Khatun M, Ray RB. Mechanisms underlying hepatitis C virus-associated hepatic fibrosis. Cells. (2019) 8:1249. doi: 10.3390/cells8101249
172. Cacoub P, Comarmond C, Domont F, Savey L, Saadoun D. Cryoglobulinemia Vasculitis. Am J Med. (2015) 128:950–5. doi: 10.1016/j.amjmed.2015.02.017
173. Feldman L, Dhamne M, Li Y. Neurologic manifestations associated with cryoglobulinemia: A single center experience. J Neurol Sci. (2019) 398:121–7. doi: 10.1016/j.jns.2019.01.041
174. Charrel RN. Emergence of Toscana virus in the mediterranean area. World J Virology. (2012) 1:135–41. doi: 10.5501/wjv.v1.i5.135
175. Messacar K, Abzug MJ, Dominguez SR. 2014 outbreak of enterovirus D68 in North America. J Med Virol. (2016) 88:739–45. doi: 10.1002/jmv.24410
176. Greninger AL, Naccache SN, Messacar K, Clayton A, Yu G, Somasekar S, et al. A novel outbreak enterovirus D68 strain associated with acute flaccid myelitis cases in the USA (2012-14): a retrospective cohort study. Lancet Infect Dis. (2015) 15:671–82. doi: 10.1016/S1473-3099(15)70093-9
177. Ooi MH, Wong SC, Lewthwaite P, Cardosa MJ, Solomon T. Clinical features, diagnosis, and management of enterovirus 71. Lancet Neurol. (2010) 9:1097–105. doi: 10.1016/S1474-4422(10)70209-X
178. MORI M, TAKAGI K, KUWABARA S, HATTORI T, KOJIMA S. Guillain-Barre Syndrome Following Hand-foot-and-mouth Disease. Internal Med. (2000) 39:503–5. doi: 10.2169/internalmedicine.39.503
179. McMinn P, Stratov I, Nagarajan L, Davis S. Neurological manifestations of enterovirus 71 infection in children during an outbreak of hand, foot, and mouth disease in Western Australia. Clin Infect Dis. (2001) 32:236–42. doi: 10.1086/318454
180. Jehn UW, Fink MK. Myositis, myoglobinemia, and myoglobinuria associated with enterovirus echo 9 infection. Arch Neurol. (1980) 37:457–8. doi: 10.1001/archneur.1980.00500560087016
181. Morrey J, Wang H, Hurst B, Zukor K, Siddharthan V, van Wettere A, et al. Causation of acute flaccid paralysis by myelitis and myositis in enterovirus-D68 infected mice deficient in interferon αβ/γ receptor deficient mice. Viruses. (2018) 10:33. doi: 10.3390/v10010033
182. Jongen PJ, Zoll GJ, Beaumont M, Melchers WJ, van de Putte LB, Galama JM. Polymyositis and dermatomyositis: no persistence of enterovirus or encephalomyocarditis virus RNA in muscle. Ann Rheum Dis. (1993) 52:575–8. doi: 10.1136/ard.52.8.575
183. van den Berg B, van der Eijk AA, Pas SD, Hunter JG, Madden RG, Tio-Gillen AP, et al. Guillain-Barré syndrome associated with preceding hepatitis E virus infection. Neurology. (2014) 82:491–7. doi: 10.1212/WNL.0000000000000111
184. Geurtsvankessel CH, Islam Z, Mohammad QD, Jacobs BC, Endtz HP, Osterhaus ADME. Hepatitis E and Guillain-Barre syndrome. Clin Infect Dis. (2013) 57:1369–70. doi: 10.1093/cid/cit512
185. Avila JD, Lacomis D, Lam EM. Neuralgic amyotrophy associated with hepatitis E virus infection: first case in the United States. J Clin Neuromuscul Dis. (2016) 18:96–100. doi: 10.1097/CND.0000000000000137
186. Bisciglia M, van den Bergh P, Duprez T, Kabamba BM, Ivanoiu A. Neuralgic amyotrophy associated with hepatitis E virus (HEV) infection: a case report. Acta Neurol Belg. (2017) 117:555–7. doi: 10.1007/s13760-016-0642-1
187. Njabom CN, Gilbert A, Brasseur E, Zandona R, Ghuysen A, D'Orio V. Parsonage-turner syndrome as a rare extrahepatic complication of hepatitis E infection. Eur J Case Rep Intern Med. (2019) 6:001208. doi: 10.12890/2019_001208
188. Eberhardt O, Kuker W, Dichgans J, Weller M. HSV-2 sacral radiculitis (Elsberg syndrome). Neurology. (2004) 63:758–9. doi: 10.1212/01.WNL.0000134652.51657.10
189. Gershon AA, Breuer J, Cohen JI, Cohrs RJ, Gershon MD, Gilden D, et al. Varicella zoster virus infection. Nat Rev Dis Primers. (2015) 1:15016. doi: 10.1038/nrdp.2015.16
190. Mishell JH, Applebaum EL. Ramsay-hunt syndrome in a patient with HIV infection. Otolaryngol–Head Neck Surg. (1990) 102:177–9. doi: 10.1177/019459989010200215
191. Adour KK. Otological complications of herpes zoster. Ann Neurol. (1994) 35:S62–4. doi: 10.1002/ana.410350718
192. Moodley A, Swanson J, Grose C, Bonthius DJ. Severe herpes zoster following varicella vaccination in immunocompetent young children. J Child Neurol. (2019) 34:184–8. doi: 10.1177/0883073818821498
193. Zerboni L, Sen N, Oliver SL, Arvin AM. Molecular mechanisms of varicella zoster virus pathogenesis. Nat Rev Microbiol. (2014) 12:197–210. doi: 10.1038/nrmicro3215
195. Majid A, Galetta SL, Sweeney CJ, Robinson C, Mahalingam R, Smith J, et al. Epstein–Barr virus myeloradiculitis and encephalomyeloradiculitis. Brain. (2002) 125:159–65. doi: 10.1093/brain/awf010
196. Gavin C, Langan Y, Hutchinson M. Cranial and peripheral neuropathy due to Epstein-Barr virus infection. Postgrad Med J. (1997) 73:419–20. doi: 10.1136/pgmj.73.861.419
197. Dorris CB, Gallagher D, Black M. Glandular fever and Epstein-Barr virus-related polyneuropathy: a life-threatening complication. BMJ Case Rep. (2020) 13:e235678. doi: 10.1136/bcr-2020-235678
198. Bennett JL, Mahalingam R, Wellish MC, Gilden DH. Epstein-barr virus-associated acute autonomic neuropathy. Ann Neurol. (1996) 40:453–5. doi: 10.1002/ana.410400316
199. Rubin DI, Daube JR. Subacute sensory neuropathy associated with Epstein-Barr virus. Muscle Nerve. (1999) 22:1607–10. doi: 10.1002/(SICI)1097-4598(199911)22:11<1607::AID-MUS21>3.0.CO;2-J
200. Kanai K, Kuwabara S, Mori M, Arai K, Yamamoto T, Hattori T. Leukocytoclastic-vasculitic neuropathy associated with chronic Epstein-Barr virus infection. Muscle Nerve. (2003) 27:113–6. doi: 10.1002/mus.10287
201. Lee JY, Sunwoo JS, Kwon KY, Lee KB, Ahn MY, Roh H. Guillain-Barré syndrome supervening on meningitis in primary Epstein-Barr virus infection. Ann Clin Neurophysiol. (2019) 21:48–52. doi: 10.14253/acn.2019.21.1.48
202. Ferraro D, Galli V, Simone AM, Bedin R, Vitetta F, Merelli E, et al. Cerebrospinal fluid anti-Epstein-Barr virus specific oligoclonal IgM and IgG bands in patients with clinically isolated and Guillain-Barré syndrome. J Neurovirol. (2017) 23:329–34. doi: 10.1007/s13365-016-0493-9
203. Chang L, Xiong J, Xue Y, Wang J, Zhu X, Zheng X, et al. An incomplete form of anti-ganglioside antibody-positive Miller Fisher syndrome after an Epstein-Barr virus infection. Medicine. (2021) 100:e24451. doi: 10.1097/MD.0000000000024451
204. Kanna A, Nasseri M, Kakara M, Elsayed M. Epstein-Barr virus driven acute motor axonal neuropathy with central demyelination following stem-cell transplant (1740). Neurology. (2020) 94:S1740.
205. Cabrera Muras A, Carmona-Abellán MM, Collía Fernández A, Uterga Valiente JM, Antón Méndez L, García-Moncó JC. Bilateral facial nerve palsy associated with COVID-19 and Epstein–Barr virus co-infection. Eur J Neurol. (2021) 28:358–60. doi: 10.1111/ene.14561
206. Taylor GH. Cytomegalovirus. Am Fam Physician. (2003) 67:519–24. Available Online At: https://www.cfp.ca/content/63/5/365.long
207. Griffiths PD, Whitley RJ. Cytomegalovirus. In: Practical Guidelines in Antiviral Therapy. Elsevier. (2002). doi: 10.1016/B978-044450884-3/50007-6
208. Meier PA, Stephan KT, Blatt SP. Cytomegalovirus polyradiculopathy in HIV-infected patients. J Gen Intern Med. (1996) 11:47–9. doi: 10.1007/BF02603486
209. Schmitz H, Enders G. Cytomegalovirus as a frequent cause of Guillain-Barré syndrome. J Med Virol. (1977) 1:21–7. doi: 10.1002/jmv.1890010105
210. Orlikowski D, Porcher R, Sivadon-Tardy V, Quincampoix JC, Raphael JC, Durand MC, et al. Guillain-Barre syndrome following primary cytomegalovirus infection: a prospective cohort study. Clin Infect Dis. (2011) 52:837–44. doi: 10.1093/cid/cir074
211. Silva CT, Silva S, Silva MJ, Almeida AF, Fonseca J, Melo C, et al. Guillain-Barré Syndrome in a Teenage Girl: A Severe Case With Anti-GM2 Antibodies Associated With Acute CMV Infection and Literature Review. Clin Pediatr. (2020) 59:300–4. doi: 10.1177/0009922819898186
212. Cassis-Ghavami F, Curlin M, Geise R, Duerr A. Human immunodeficiency virus. In: Vaccines for Biodefense and Emerging and Neglected Diseases. Amsterdam. (2009). doi: 10.1016/B978-0-12-369408-9.00025-1
213. Kranick SM, Nath A. Neurologic Complications of HIV-1 infection and its treatment in the era of antiretroviral therapy. CONTINUUM: Lifelong Learn Neurol. (2012) 18:1319–37. doi: 10.1212/01.CON.0000423849.24900.ec
214. Gawde AK, Emmady PD. HIV-1 Associated Progressive Polyradiculopathy. Tampa, FL: StatPearls Publishing (2022).
215. Geraci AP, Simpson DM. Neurological manifestations of HIV-1 infection in the HAART era. Compreh Ther. (2001) 27:232–41. doi: 10.1007/s12019-001-0020-6
216. Keswani SC, Jack C, Zhou C, Hoke A. Establishment of a rodent model of HIV-associated sensory neuropathy. J Neurosci. (2006) 26:10299–304. doi: 10.1523/JNEUROSCI.3135-06.2006
217. Amato AA, Ropper AH. Sensory ganglionopathy. N Engl J Med. (2020) 383:1657–62. doi: 10.1056/NEJMra2023935
218. Brahic M, Smith RA, Gibbs CJ, Garruto RM, Tourtellotte WW, Cash E. Detection of picornavirus sequences in nervous tissue of amyotrophic lateral sclerosis and control patients. Ann Neurol. (1985) 18:337–43. doi: 10.1002/ana.410180311
219. Huang HI, Shih SR. Neurotropic enterovirus infections in the central nervous system. Viruses. (2015) 7:6051–66. doi: 10.3390/v7112920
220. Anastasina M, Domanska A, Palm K, Butcher S. Human picornaviruses associated with neurological diseases and their neutralization by antibodies. J General Virology. (2017) 98:1145–58. doi: 10.1099/jgv.0.000780
221. Cermelli C, Vinceti M, Beretti F, Pietrini V, Nacci G, Pietrosemoli P, et al. Risk of sporadic amyotrophic lateral sclerosis associated with seropositivity for herpesviruses and echovirus-7. Eur J Epidemiol. (2003) 18:123–7. doi: 10.1023/A:1023067728557
222. Walker MP, Schlaberg R, Hays AP, Bowser R, Lipkin WI. Absence of echovirus sequences in brain and spinal cord of amyotrophic lateral sclerosis patients. Ann Neurol. (2001) 49:249–53. doi: 10.1002/1531-8249(20010201)49:2<249::AID-ANA46>3.0.CO;2-3
223. MacGowan DJL, Scelsa SN, Waldron M. An ALS-like syndrome with new HIV infection and complete response to antiretroviral therapy. Neurology. (2001) 57:1094–7. doi: 10.1212/WNL.57.6.1094
224. Alfahad T, Nath A. Retroviruses and amyotrophic lateral sclerosis. Antiviral Res. (2013) 99:180–7. doi: 10.1016/j.antiviral.2013.05.006
225. Dinges WL, Witherspoon SR, Itani KM, Garg A, Peterson DM. Blepharoptosis and External Ophthalmoplegia Associated with Long-Term Antiretroviral Therapy. Clin Infect Dis. (2008) 47:845–52. doi: 10.1086/591202
226. Pfeffer G, Sirrs S, Wade NK, Mezei MM. Multisystem disorder in late-onset chronic progressive external ophthalmoplegia. Canad J Neurol Sci. (2011) 38:119–23. doi: 10.1017/S031716710001115X
227. Matsuzaki T, Nakagawa M, Nagai M, Nobuhara Y, Usuku K, Higuchi I, et al. HTLV-I-associated myelopathy (HAM)/tropical spastic paraparesis (TSP) with amyotrophic lateral sclerosis-like manifestations. J Neurovirol. (2000) 6:544–8. doi: 10.3109/13550280009091955
228. Toufaily C, Landry S, Leib-Mosch C, Rassart E, Barbeau B. Activation of LTRs from different human endogenous retrovirus (HERV) families by the HTLV-1 tax protein and T-cell activators. Viruses. (2011) 3:2146–59. doi: 10.3390/v3112146
229. Matsuura E, Umehara F, Nose H, Higuchi I, Matsuoka E, Izumi K, et al. Inclusion Body Myositis Associated With Human T-Lymphotropic Virus-Type I Infection. J Neuropathol Exper Neurol. (2008) 67:41–9. doi: 10.1097/nen.0b013e31815f38b7
230. Dervan E, Bhattacharyya DD, McAuliffe JD, Khan FH, Glynn SA. Ancient Adversary – HERV-K (HML-2) in Cancer. Front Oncol. (2021) 11:658489. doi: 10.3389/fonc.2021.658489
231. Garcia-Montojo M, Fathi S, Norato G, Smith BR, Rowe DB, Kiernan MC, et al. Inhibition of HERV-K (HML-2) in amyotrophic lateral sclerosis patients on antiretroviral therapy. J Neurol Sci. (2021) 423:117358. doi: 10.1016/j.jns.2021.117358
232. Mayer J, Harz C, Sanchez L, Pereira GC, Maldener E, Heras SR, et al. Transcriptional profiling of HERV-K(HML-2) in amyotrophic lateral sclerosis and potential implications for expression of HML-2 proteins. Mol Neurodegener. (2018) 13:39. doi: 10.1186/s13024-018-0275-3
233. Garson JA, Usher L, Al-Chalabi A, Huggett J, Day EF, McCormick AL. Quantitative analysis of human endogenous retrovirus-K transcripts in postmortem premotor cortex fails to confirm elevated expression of HERV-K RNA in amyotrophic lateral sclerosis. Acta Neuropathol Commun. (2019) 7:45. doi: 10.1186/s40478-019-0698-2
234. Rex C, Nadeau MJ, Douville R, Schellenberg K. Expression of human endogenous retrovirus-k in spinal and bulbar muscular atrophy. Front Neurol. (2019) 10:968. doi: 10.3389/fneur.2019.00968
235. van Oosterhout C, Hall N, Ly H, Tyler KM. COVID-19 evolution during the pandemic – Implications of new SARS-CoV-2 variants on disease control and public health policies. Virulence. (2021) 12:507–8. doi: 10.1080/21505594.2021.1877066
236. Aucott JN, Rebman AW. Long-haul COVID: heed the lessons from other infection-triggered illnesses. Lancet. (2021) 397:967–8. doi: 10.1016/S0140-6736(21)00446-3
237. Arnold DT, Hamilton FW, Milne A, Morley AJ, Viner J, Attwood M, et al. Patient outcomes after hospitalisation with COVID-19 and implications for follow-up: results from a prospective UK cohort. Thorax. (2021) 76:399–401. doi: 10.1136/thoraxjnl-2020-216086
238. Moreno-Pérez O, Merino E, Leon-Ramirez JM, Andres M, Ramos JM, Arenas-Jiménez J, et al. Post-acute COVID-19 syndrome. Incidence and risk factors: A Mediterranean cohort study. J Infection. (2021) 82:378–83. doi: 10.1016/j.jinf.2021.01.004
239. Tankisi H, Tankisi A, Harbo T, Markvardsen LK, Andersen H, Pedersen TH. Critical illness myopathy as a consequence of Covid-19 infection. Clin Neurophysiol. (2020) 131:1931–2. doi: 10.1016/j.clinph.2020.06.003
240. Nalbandian A, Sehgal K, Gupta A, Madhavan M v, McGroder C, Stevens JS, et al. Post-acute COVID-19 syndrome. Nat Med. (2021) 27:601–15. doi: 10.1038/s41591-021-01283-z
241. Farmakidis C, Dimachkie MM, Pasnoor M, Barohn RJ. Immunosuppressive and immunomodulatory therapies for neuromuscular diseases. Part I: Tradit Agents Muscle Nerve. (2020) 61:5–16. doi: 10.1002/mus.26708
242. Farmakidis C, Dimachkie MM, Pasnoor M, Barohn RJ. Immunosuppressive and immunomodulatory therapies for neuromuscular diseases. Part II: New Novel Agents Muscle Nerve. (2020) 61:17–25. doi: 10.1002/mus.26711
243. Qiu S, Jacobson R. Structured review of opportunistic infections in neuromuscular disease (1252). Neurology. (2021) 96:1252.
244. Canada MD. What you need to know about Covid-19. (2020). Available online at: https://muscle.ca/covid-19/what-you-need-to-know-about-covid-19/ (accessed June 15, 2021).
245. Minotti C, Tirelli F, Barbieri E, Giaquinto C, Donà D. How is immunosuppressive status affecting children and adults in SARS-CoV-2 infection? A systematic review. J Infect. (2020) 81:e61–6. doi: 10.1016/j.jinf.2020.04.026
Keywords: COVID-19, SARS-CoV-2, neuromuscular disease (NMD), Guillain-Barre syndrome, viral disease, autoimmune disease
Citation: Jacob S, Kapadia R, Soule T, Luo H, Schellenberg KL, Douville RN and Pfeffer G (2022) Neuromuscular Complications of SARS-CoV-2 and Other Viral Infections. Front. Neurol. 13:914411. doi: 10.3389/fneur.2022.914411
Received: 06 April 2022; Accepted: 25 May 2022;
Published: 24 June 2022.
Edited by:
Angelo Schenone, University of Genoa, ItalyReviewed by:
Georgios E. Manousakis, University of Minnesota, United StatesSamir Abu Rumeileh, Martin Luther University of Halle-Wittenberg, Germany
Copyright © 2022 Jacob, Kapadia, Soule, Luo, Schellenberg, Douville and Pfeffer. This is an open-access article distributed under the terms of the Creative Commons Attribution License (CC BY). The use, distribution or reproduction in other forums is permitted, provided the original author(s) and the copyright owner(s) are credited and that the original publication in this journal is cited, in accordance with accepted academic practice. No use, distribution or reproduction is permitted which does not comply with these terms.
*Correspondence: Gerald Pfeffer, gerald.pfeffer@ucalgary.ca