Noradrenergic Hypothesis Linking Neurodegeneration-Based Cognitive Decline and Astroglia
- 1Department of Drug Sciences, University of Catania, Catania, Italy
- 2Department of Biomedical and Biotechnological Sciences, University of Catania, Catania, Italy
- 3Laboratory of Neuroendocrinology-Molecular Cell Physiology, Faculty of Medicine, Institute of Pathophysiology, University of Ljubljana, Ljubljana, Slovenia
- 4Laboratory of Cell Engineering, Celica Biomedical, Ljubljana, Slovenia
In the past, manipulation of the cholinergic system was seen as the most likely therapeutic for neurodegeneration-based cognitive decline in Alzheimer's disease (AD) (Whitehouse et al., 1982). However, targeting the noradrenergic system also seems a promising strategy, since more recent studies revealed that in post-mortem tissue from patients with AD and other neurodegenerative disorders there is a robust correlation between cognitive decline and loss of neurons from the Locus coeruleus (LC), a system with diffuse noradrenaline (NA) innervation in the central nervous system (CNS). Therefore, the hypothesis has been considered that increasing NA signaling in the CNS will prevent, or at least halt the progression of neurodegeneration and cognitive decline. A hallmark of the age- and neurodegeneration-related cognitive decline is reduced neurogenesis. We here discuss noradrenergic dysfunction in AD-related cognitive decline in humans and its potential involvement in AD pathology and disease progression. We also focus on animal models to allow the validation of the noradrenergic hypothesis of AD, including those based upon the immunotoxin-mediated ablation of LC based on saporin, a protein synthesis interfering agent, which offers selective and graded demise of LC neurons, Finally, we address how astrocytes, an abundant and functionally heterogeneous cell type of neuroglia maintaining homeostasis, may participate in the regulation of neurogenesis, a new strategy for preventing LC neuron loss.
Introduction
With its widespread efferent projections, the small brainstem nucleus Locus coeruleus (LC) represents the main source of noradrenergic innervation to the entire CNS, and plays a pivotal regulatory role in a variety of physiological processes, including attention, arousal, sleep/wakefulness, consciousness as well as in specific aspects of learning and memory (Amaral and Sinnamon, 1977; Aston-Jones and Cohen, 2005; Benarroch, 2009; Sara, 2009). Notably, increasing clinical and imaging evidences (Peterson and Li, 2018) indicate that LC degeneration constitutes a crucial early event in the pathogenesis of Alzheimer's (AD) and Parkinson's disease and most of the LC-regulated functions have been shown to be severely affected during its progression (Chan-Palay and Asan, 1989; Rub et al., 2001; Wilson et al., 2013; Arendt et al., 2015). Taken together, these observations have pointed at the noradrenergic system as a viable therapeutic target for the treatment of diseases characterized by memory loss and cognitive decline. However, a feasible animal model recapitulating noradrenergic neuronal and terminal fiber loss and its histopathological and cognitive sequelae has not yet been achieved, mainly due to the objective difficulty to selectively and efficiently target LC neurons. This review seeks to briefly summarize noradrenergic dysfunction in AD-related memory loss and its potential involvement in AD pathology and progression. Also, recent findings emerging from our own studies addressing selective immunotoxic ablation of LC neurons and its effects upon cognitive performance, tissue pathology and hippocampal neurogenesis will be briefly outlined. Then we focus into impaired neurogenesis in neurodegeneration and point to the possible contribution of astroglia to this process. Invariably, much relevant literature on the aforementioned topics will not be mentioned here, and we apologize for this.
Locus Coeruleus Dysfunction in Alzheimer's Disease
The existence of an association between noradrenergic depletion and neurodegeneration including AD has long been known (Ishii, 1966; Forno and Alvord, 1971; Mann et al., 1980, 1982; Tomlinson et al., 1981; Iversen et al., 1983), however only in relatively recent years has noradrenergic neuron loss in LC been widely acknowledged as a prominent feature of neurodegeneration and AD, being present often decades prior to the appearance of clinical symptoms, and having been related to neurofibrillary pathology and the severity of cognitive deficits, when overtly present (Haglund et al., 2006; Grudzien et al., 2007; Braak and Del Tredici, 2011a,b; Wilson et al., 2013; Andres-Benito et al., 2017; Peterson and Li, 2018).
There have also been conflicting reports regarding the NA levels in the brain of AD patients. In fact, while some studies have reported marked decline in regional NA brain tissue content, whose magnitude correlated with the severity of cognitive impairments (Martignoni et al., 1992; Nazarali and Reynolds, 1992; Matthews et al., 2002; Chen et al., 2014), others reported no changes (Sparks et al., 1988; Herregodts et al., 1989; Tohgi et al., 1992) or even increased NA levels (Tohgi et al., 1992; Elrod et al., 1997).
Similar conflicting observations in post-mortem brain specimens from AD patients have been reported for adrenergic receptors (Shimohama et al., 1986; Kalaria et al., 1989; Pascual et al., 1992; Leverenz et al., 2001), known to be key mediators of noradrenergic activity, and recently considered relevant candidates as novel therapeutic targets for AD (Chen et al., 2014). These discrepancies, likely reflecting compensatory responses, or their lack, at more advanced stages of the disease (Szot et al., 2006, 2007), have thus driven the need to conclusively dissect the exact role played by the noradrenergic system in the cognitive sequelae and pathogenesis that characterize AD and neurodegeneration in general. During the last decades, numerous animal studies and reviews (Mather and Harley, 2016; Borodovitsyna et al., 2017; Gannon and Wang, 2018) have provided valuable insights into the factors underlying the disease. It has been shown, for example, that - possibly via their direct connections to the prefrontal cortex and hippocampus - LC neurons have a fundamental role in sustaining behavioral responsiveness upon exposure to relevant, reward-predicting, stimuli (Bouret and Sara, 2004; Hagena et al., 2016), including those related to working memory (Milstein et al., 2007; Coradazzi et al., 2016). Furthermore, LC neuron degeneration offers a major contribution to AD pathogenesis and progression (Braak et al., 2011; Iba et al., 2015). In fact, a prevailing hypothesis for noradrenergic neuron and fiber depletion in AD holds that LC neurons are uniquely susceptible to tau toxicity (Chandler et al., 2014) and are especially vulnerable to oxidative stress, possibly owing to their high bioenergetic needs (Sanchez-Padilla et al., 2014). In such scenario, accumulation of abnormally phosphorylated tau in LC neurons and its spreading to most of the brain due to the extremely diffuse efferent projections, would account for the progression of the disease (Braak and Del Tredici, 2015), and the resulting neuronal degeneration and cognitive impairments.
Experimental Animal Paradigms to Study Noradrenergic Dysfunction
Animal studies have so far been extremely helpful to dissect the importance of NA in cognition and in the pathological events associated to its loss. Experimental manipulation of the noradrenergic system by e.g., pharmacological blockade (Mair et al., 2005; Khakpour-Taleghani et al., 2009), lesioning with N-(2-chloro-ethyl)-N-ethyl-2- bromobenzylamine (DSP4), reportedly an LC-selective neurotoxin (Lapiz et al., 2001; Sontag et al., 2008) or the knockout of the dopamine-β-hydroxylase (DBH) gene (Thomas and Palmiter, 1997; Marino et al., 2005; Hammerschmidt et al., 2013) have all resulted in impaired performance in several learning and memory tasks, demonstrating the existence of an association between NA loss and disturbances in various aspects of cognition. However, in many cases, the impairments observed in these studies have appeared rather inconsistent, both in efficiency and selectivity, thus highlighting the potential limitations inherent to each method (Sontag et al., 2008; Khakpour-Taleghani et al., 2009; Szot et al., 2010; Gannon et al., 2015). In fact, pharmacological agents lack anatomical and neurochemical resolution, acting on most monoaminergic neurons and cells throughout the central and peripheral nervous system. Likewise, DSP4 does not seem to be specific for noradrenergic neurons and has been shown to produce only a modest noradrenergic neuron loss, at best. Finally, although DBH (–/–) knockout mice have provided the unique opportunity to precisely assess the effects of NA loss per se, with respect to the various modulators produced and released by the very same LC neurons, they do not seem to offer the possibility to obtain partial or graded neurotransmitter depletions. In light of these limitations, we have chosen an alternative lesioning approach based on the use of the immunotoxin anti-DBH-saporin (Picklo et al., 1994), able to target noradrenergic neurons in the LC with unprecedented selectivity and efficiency. This immunotoxin results from the conjugation of saporin, a powerful ribosome-inactivating plant lectin extracted from Saponaria officinalis (Caryophyllaceae) (Lappi et al., 1985; Barthelemy et al., 1993) to a monoclonal antibody raised against DBH (the enzyme converting dopamine to NA) that, in addition to its main localization in the cytosol, is also expressed at the plasma membrane surface of noradrenergic neurons (Weinshilboum, 1978; Studelska and Brimijoin, 1989). Due to its structure, saporin cannot enter the cell (Contestabile and Stirpe, 1993), but when coupled to a carrier molecule (e.g., an antibody), is able to specifically bind a surface antigen protein (such as DBH, in this case), the toxin gains access to the cytosol and binds to the ribosomal 60S subunit, interfering with protein synthesis, and soon leading to cell death (Wiley and Kline, 2000). In initial anatomical investigations, the immunotoxin, infused into the lateral ventricles of either adults (Wrenn et al., 1996) or developing rats (Coradazzi et al., 2010) has been observed to produce highly specific and dose-dependent depletions of LC neurons, with no effects on other cholinergic, dopaminergic or serotonergic neuronal populations (Figure 1). Notably, the possibility to induce a partial or total noradrenergic loss (by varying the injected dose) makes this immunotoxic approach extremely suitable to address compensatory events within the noradrenergic projection system, in addition to providing an excellent tissue environment for the survival and integration of implanted NA-rich progenitors (Coradazzi et al., 2010).
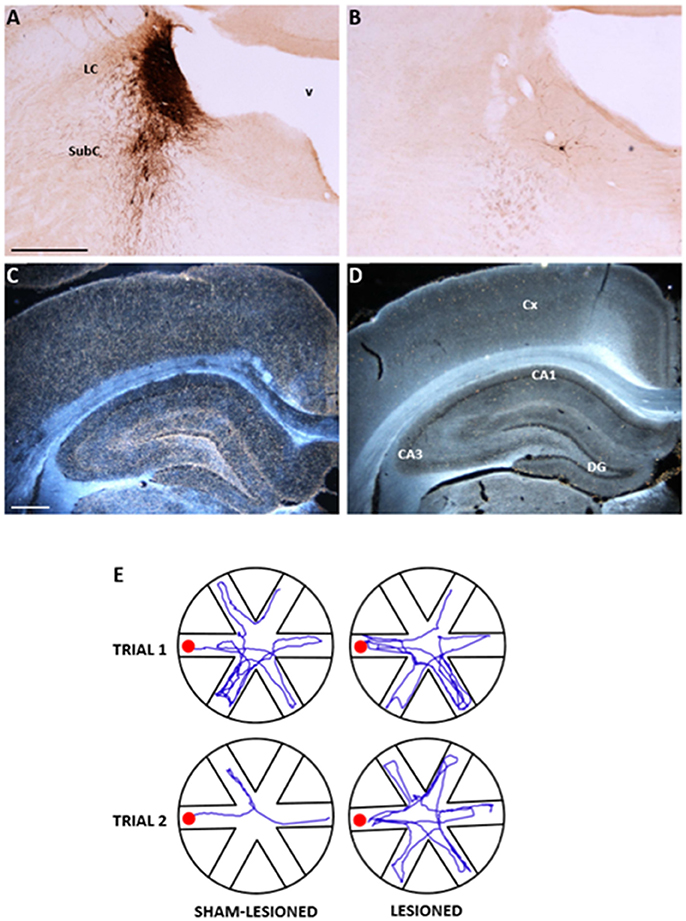
Figure 1. Anatomical and functional effects of the selective noradrenergic lesion in young adult Sprague-Dawley rats. (A–D): Photomicrographs showing the effects of the anti-DBH immunotoxin, injected bilaterally into the LC, on noradrenergic neurons in the LC/SubC (A,B) and on the DBH-immunoreactive terminal innervation in the parietal cortex and hippocampus (C,D, in dark field). Note in (B,D) the nearly complete loss of immunoreactive neurons and fibers induced by the lesion, compared to the normal patterns in the specimens from a sham-lesioned animal (A,C). In (E), the actual swim paths taken by representative sham-lesioned and lesioned animals undergoing the Radial Arm Water Maze (RAWM) task for working memory are illustrated. The sham-lesioned animal rapidly learns the task and significantly improves its performance from the first to the second trial, whereas the lesioned animal does not. LC, locus coeruleus; SubC, subcoeruleus, v fourth ventricle, CA, cornu ammonis of the hippocampus; DG, dentate gyrus. Scale bars in (A,C): 500 μm. Adapted from Coradazzi et al. (2016).
Noradrenaline and Adult Hippocampal Neurogenesis
The subgranular zone of the hippocampal dentate gyrus is one of the brain regions where generation of neural progenitor cells occurs thoughout life in various species, including humans (Altman and Das, 1965; Cameron et al., 1993; Eriksson et al., 1998; Gould et al., 1998). From here, proliferating newborn cells migrate to the granule cell layer, where they differentiate into neurons and glia and functionally integrate into the local tissue environment (Markakis and Gage, 1999; Carlen et al., 2002; van Praag et al., 2002). Many factors have been observed to affect hippocampal neurogenesis, including environmental or stressful stimuli (Dranovsky and Hen, 2006), various kinds of hormones and drugs (Duman et al., 2001), as well as neurotransmitter activity (Brezun and Daszuta, 1999; Mohapel et al., 2005, 2006; Aztiria et al., 2007; Walker et al., 2008). Notably, the hippocampus is the region where explicit memories are apparently acquired and consolidated (Murchison et al., 2004), and one of the brain areas receiving the densest LC-derived noradrenergic innervation (Swanson and Hartman, 1975). Moreover, hippocampal neurogenesis has been proposed to underlie some of the behavioral effects of antidepressant drugs (Santarelli et al., 2003; Warner-Schmidt and Duman, 2006; Airan et al., 2007), whose actions are mainly exerted by increasing extracellular levels of serotonin and/or noradrenaline (Fuller et al., 1994; Sacchetti et al., 1999). It is therefore not surprising that several studies have begun to investigate the contribution of LC neurons to the regulation of hippocampal neurogenesis, and have generally reported a permissive role for NA upon hippocampal neurogenesis (Kulkarni et al., 2002; Jhaveri et al., 2010, 2014; Masuda et al., 2012). Again, however, none of the lesion or pharmacological manipulations adopted in these investigations proved to be region-or transmitter specific, nor were any of the observed effects analyzed also in terms of impact upon cognitive performance. In our study using selective and discrete immunolesioning of LC neurons, associated with a series of hippocampus-dependent spatial navigation tasks (Coradazzi et al., 2016), we found severe deficits in working memory, which correlated with the magnitude of hippocampal noradrenergic depletion and the lesion-induced reduction in the numbers of proliferating cells within the dentate gyrus. Notably, no changes were detected in reference memory abilities, nor did the lesion affect long-term survival or differentiation of granule cell progenitors (Coradazzi et al., 2016). Thus, the noradrenergic regulation of complex aspects of cognitive function (e.g., those related to working memory) may take place via the proliferation of progenitor cells in the hippocampal dentate gyrus.
Noradrenaline, Astroglia and Neurogenesis
It appears that noradrenergic receptors are densely present in astroglia (Aoki, 1992), therefore it is likely that NA may affect neuronal circuits via astroglia (Ding et al., 2013; Paukert et al., 2014; Pankratov and Lalo, 2015; Gao et al., 2016; Dong et al., 2017) (Figure 2. graphical abstract). Current view holds that neurodegeneration in AD is a consequence of neuron-specific deficits. However, it is more likely that preceding or concomitant changes in neuroglia may also contribute to this process (Heneka et al., 2015; Verkhratsky and Parpura, 2015; De Strooper and Karran, 2016; Rodriguez-Vieitez et al., 2016; Stenovec et al., 2016; Verkhratsky et al., 2016). Astroglia, a type of neuroglia, consisting also of oligodendroglia, microglia and NG2 cells, are functionally and morphologically heterogeneous, and are involved in sustaining brain homeostasis at cellular and whole organ levels, by regulating extracellular levels of ions and neurotransmitters, by controlling vascular and metabolic functions, the integrity of blood-brain barrier (BBB) (Terry, 2000; Giaume et al., 2007; Kano and Hashimoto, 2009; Heneka et al., 2010; Nedergaard et al., 2010; Parpura and Zorec, 2010; Verkhratsky and Nedergaard, 2014, 2018; Zorec et al., 2018). Importantly, astrocytes are essential in orchestrating defense in the CNS as well; pathological states in the CNS lead to reactive astrogliosis, a process that contains and isolates events taking place in the damaged brain regions. Moreover, reactive astrogliosis is also augmenting post-damage regeneration and repair of brain tissue (Parpura et al., 2012; Pekny et al., 2016; Verkhratsky et al., 2016); however, under certain conditions reactive astrogliosis can be neurotoxic (Liddelow et al., 2017). Astrocytes were also termed gliocrine cells (Vardjan and Zorec, 2015), since they secrete gliosignalling molecules into the extracellular space and are then convectively distributed thoroughout the brain by the glymphatic system (at least in mice), responsible for waste removal (Thrane et al., 2014). Changes in these complex functions of astroglia may lead to a homeostatic failure, leading to disease (Verkhratsky and Parpura, 2015). Hence, primary defect in homeostatic astroglia may lead to a secondary defect in neurons. Interestingly, the role of neuroglial cells in dementia and AD was noted already at least a century ago by A. Alzheimer, who observed glial cells in the proximity of damaged neurons (Alzheimer, 1907, 1910; Strassnig and Ganguli, 2005).
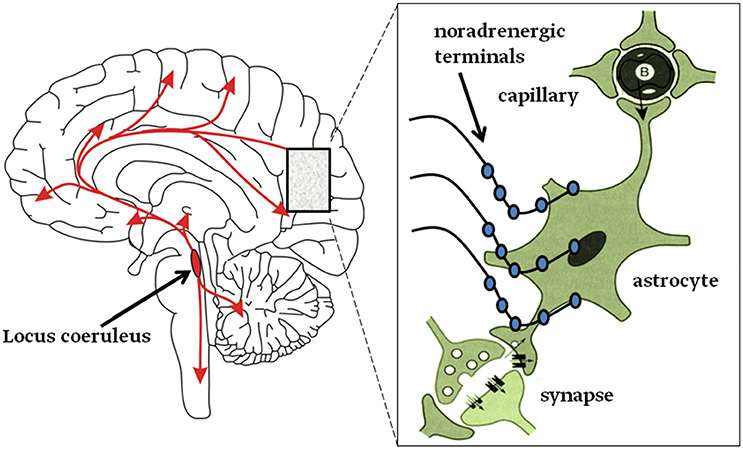
Figure 2. Graphical abstract. Left: neurons from the Locus coeruleus (LC) project axons to most, if not all, areas of the brain and into the spinal cord as denoted by the arrows. Right: inset magnifies the LC nerve endings with varicosities (swellings on noradrenergic nerve terminals) from which NA is released and mainly activates astroglia (Aoki, 1992; Sherpa et al., 2016). About half of these terminals do not form tight contacts with target cells, resembling synapses (Feinstein et al., 2016).
In tissue from post-mortem patients with AD, several morphological observations were made, including astroglial hypertrophy, associated with reactive astrogliosis, the hallmark of which is increased expression of cytoskeletal glial fibrillary acidic protein (GFAP) and S100, a Ca2+-binding protein; these changes in astrocytes were seen associated with senile plaques (Beach and McGeer, 1988; Griffin et al., 1989; Nagele et al., 2004; Mrak and Griffin, 2005; Verkhratsky et al., 2014). Further, studying patients with AD by imaging methods revealed distinct time- and brain-specific morphological alterations (Rodriguez-Vieitez et al., 2016). Similar changes occurred in an animal mouse model of familial AD as well, including astroglial atrophy in addition to hypertrophy in certain areas of the CNS (Olabarria et al., 2010, 2011; Yeh et al., 2011; Kulijewicz-Nawrot et al., 2012). The appearance of asthenic astrocytes appeared to preced the presence of senile plaques; asthenic astrocytes were at first observed in the entorhinal cortex, an area affected early in AD pathology (Yeh et al., 2011). Ideally, studies of pathological developments associated with neurodegeneration and AD would be best in humans, but these experiments are challenging. Recent attempts to classify protein astrogliopathies, including deposition of amyloid-β, prion protein, tau, α-synuclein, and transactive response DNA-binding protein 43 (TDP-43), demonstrated that these are present in human neurodegenerative diseases (Kovacs et al., 2017). However, animal models seem still very valuable, particularly where many aspects of this pathology can be, to some extent, reproduced, and the time emergence of AD-like characteristic take a much shorter time to develop, providing certain opportunities for studying AD-related neuropathology experimentally. Not only mice, there are several other animal models of AD including nematodes, fruit flies, rabbits, canines, and non-human primates; in each of the models different aspects of AD properties are manifested (reviewed in Woodruff-Pak, 2008). The selective ablation of LC in rats, as presented in previous chapters, offers interesting insights to be explored as a novel animal model of AD and neurodegeneration, not only for functional cognitive impairments (Coradazzi et al., 2016), but also for morphological alterations and aspects of neurogenesis related to astroglia.
Neuron degeneration in the LC during early stages of AD (Heneka et al., 2006) may lead to reduced levels of NA, which is known to be a generic inhibitor of neuroinflammation (De Keyser et al., 2004) and remodeling of the neurovascular unit (del Zoppo, 2009). Therefore, reduced noradrenergic innervation in the CNS may affect the progression of AD through reduced inhibition of neuroinflammation, a tissue remodeling process. This likely operates via a reduced NA contribution of astrocyte adrenergic excitation. Although NA released by the LC neurons acts through α- and β-adrenergic receptors (α/β-ARs), which are expressed in neurons, microglia and astrocytes, but it is the latter cell type that exhibits a high density of ARs, especially the β-ARs (Aoki, 1992) and thus represents a key cell type mediating adrenergic effects on brain tissue. Indeed, activation of α-ARs stimulates Ca2+ signaling in astrocytes (Salm and McCarthy, 1989; Kirischuk et al., 1996; Horvat et al., 2016); experiments in vivo reported Ca2+ waves propagating through astroglial syncytia after stimulating the LC in anesthetized animals (Bekar et al., 2008). In awake animals, electrical stimulation of LC triggered (via activation of α?-ARs) widespread and synchronous astroglial Ca2+ signals in practically all astrocytes in the field of study (Ding et al., 2013). This phenomenon may be taken as an event resetting neural networks (Bouret and Sara, 2005). In AD, astrocytic Ca2+ signaling is impaired (Lim et al., 2014; Stenovec et al., 2016), which may affect the clearance of Aβ deposits (Mattson, 2004) and subsequent glutamate toxicity (Mattson and Chan, 2003; Mattson, 2004).
Whenever NA is released, not only α-ARs, but also β-ARs are stimulated, although the effects appear in different time-domains (Horvat et al., 2016). These latter receptors stimulate second messenger cAMP, which affects glycogenolysis (Prebil et al., 2011; Kreft et al., 2012), a likely source of glutamate for memory consolidation (Gibbs et al., 2010). Glycogen, an energy reserve, present in astrocytes, but not in neurons (Barros, 2013; Oe et al., 2016), is likely consumed for processes related to morphological plasticity controlled by β-AR/cAMP signaling in astroglia (Vardjan et al., 2014). Not only in memory formation (Zorec et al., 2015), astroglial β-AR/cAMP-dependent morphological changes are central for astrocytic cell oedema attenuation (Vardjan et al., 2016).
Activation of ARs on astrocytes may also affect neurogenesis through neuronal metabolic support by astroglia. Reduced support due to lower NA levels may in AD result in reduced neurogenesis. Astrocytes are the site of aerobic glycolysis, a special metabolic adaptation, present in tissues exhibiting cell division and morphological plasticity. This non-oxidative utilization of glucose, takes place even in the presence of adequate levels of oxygen; it is also known as “the Warburg effect” (Vander Heiden et al., 2009). It is also a characteristic of cancer cells (Salcedo-Sora et al., 2014), hence aerobic glycolysis seems a universal property needed for tissue enlargement and cell shape remodeling.
Astroglial aerobic glycolysis, with the end product L-lactate, is regulated by NA. During exercise, sensory stimulation, alertness and in some pathophysiological states astroglial L-lactate production is up-regulated, requiering the activation of LC neurons (Dienel and Cruz, 2016; Feinstein et al., 2016). LC neurons respond to L-lactate, generated by astroglia when NA activates ARs on astroglial plasma membrane, with elevated electrical activity, an interesting form of communication between the somata of the two cell-types (Tang et al., 2014). It is possible that astroglial L-lactate may also stimulate LC axons away from the somata; as it was recently demonstarted that transcranial direct current stimulation induces NA-dependent elevation of second messenger Ca2+ in astrocytes (Monai et al., 2016). Therefore, degenerated LC neurons in AD fail to adequately stimulate astroglia, a source of necessary support for the metabolic needs of neuronal networks, contributing to attenuated neurogenesis. Hypometabolic manifestation is observed clinically in patients with AD (Rodriguez-Vieitez et al., 2016).
Cholesterol is an important building block of membranes and astroglial aerobic glycolysis is likely linked to cholesterol homeostasis in the brain, the most cholesterol-rich organ in the body (Lütjohann et al., 1996). There is little exchange of cholesterol molecules by circulating lipoproteins, between the brain and systemic circulation, since lipoproteins are unable to pass the blood–brain barrier (BBB). Interestingly, the brain exhibits its own cholesterol synthesis, which occurs mainly in glia (Mauch et al., 2001)). Brain cholesterol is not only synthesized de novo in the brain, it is also modified/degraded by enzymatic conversion to 24(S)-hydroxycholesterol (Lütjohann et al., 1996), a form that can readily cross the BBB, a major route of cholesterol exit from the brain.
Cholesterol metabolism is linked to the synthesis of the neurosteroids including allopregnanolone, a potent stimulator of neural progenitor cell survival reversing the progress of disease in the 3xTgAD mouse model (Singh et al., 2012). Thus, it is possible to speculate that the hypometabolic state in AD, due to impairment of LC-mediated adrenergic stimulation of astroglia, results in a reduced provision of metabolic intermediates and in attenuated neurosteroid-mediated maintenance of neurogenesis, an avenue that will have to be confirmed experimentally in the future.
Future Prospects and Therapy Options
Based on the above observations of the relationship between central noradrenergic depletion and a series of AD-related changes, including cognitive disturbances, and tissue pathology, it is not surprising that much interest has recently mounted concerning the possibility to restore extracellular NA levels in the brain as a prerequisite to ameliorate cognitive performance as well as to promote cell protection and neurogenesis. Several potential therapeutic venues have been explored so far: one holds that voluntary physical exercise may improve memory by enhancing NA release from LC neurons (Segal et al., 2012), an effect possibly mediated by β-ARs (Van Hoomissen et al., 2004; Ebrahimi et al., 2010). Another study has addressed the possible restorative effects of atomoxetine (a NA reuptake inhibitor) in AD patients undergoing anticholinesterase therapy, reporting no clear-cut cognitive improvements (Mohs et al., 2009), possibly due to a poor effectiveness of the NA uptake inhibitor on LC which is already severely depleted of its neurons as a result of the disease (Braun et al., 2014; Braun and Feinstein, 2017). A similar lack of cognitive improvements has been reported in aged healthy patients following treatment with guanfacine, an α2-AR agonist (Van Dyck, 2014; http://ClinicalTrials.gov) that, if anything, should reduce, rather than increase NA levels (Starke, 2001). Indeed, α2-AR antagonists appear to prevent age-related spatial working memory impairments in a transgenic AD mouse model (Scullion et al., 2011). In spite of these inconsistencies, the possibility to ameliorate cognitive performance and cell protection by enhancing noradrenergic neurotransmission remains an exciting prospect: in a recent investigation (Pintus et al., 2018) we have implanted embryonic noradrenergic progenitors bilaterally into the hippocampus of rats whose LC neurons had been almost completely and selectively depleted. We reasoned that if the loss of noradrenergic innervation to the hippocampus was necessary to induce measurable cognitive impairments, its restoration promoted by the implanted embryonic LC neurons should be sufficient to ameliorate/reverse them. As expected (and consistent with our previous findings Coradazzi et al., 2016), the LC lesion induced severe deficits in working memory which were seen fully reversed (up to normal) in transplanted animals and significantly reinstated by a second lesion ablating the implanted neuroblasts. Interestingly, the transplant-promoted noradrenergic reinnervation also normalized the nuclear expression of the transactive response DNA-binding protein 43 (TDP-43) in various hippocampal subregions, whose cytoplasmic (i.e., pathological) occurrence appeared dramatically increased as a result of the lesions. These findings therefore provide support to the view that cognitive and histopathological changes observed in AD patients may require concurrent loss of ascending regulatory noradrenergic inputs from LC and that NA replenishment may be an effective intervention to slow down and/or reverse cognitive decline and tissue pathology, including neurogenesis. Moreover, the NA replenishment may operate via astroglial adrenergic mechanism that include metabolic and neurotrophic support of neural networks.
Concluding Remarks
Noradrenergic neurons in the LC and their widespread efferent connections play a central role in normal cognition and their disruption is increasingly believed to be critically associated with severe memory loss and neurodegeneration in general (Wilson et al., 2013; Feinstein et al., 2016; Bharani et al., 2017; Satoh and Iijima, 2017). Notably, the presence of functional and densely present adrenergic receptors on astrocytes (Aoki, 1992), whose activation triggers metabolic and energetic responses supporting synaptic functioning and plasticity (Zorec et al., 2017), strongly suggests that many, if not all, the NA-mediated processes may take place also via an action on astrocyes. Thus, addressing astrocytes as viable targets for neurological diseases related to noradrenergic dysfunction is an interesting issue with fundamental therapeutic implications, warranting further research.
Author Contributions
GL, RG, and RZ conceived the concept of paper, wrote and edited the manuscript.
Funding
This work was supported by grants to RZ # P3 310, J3 6790, J3 6789 and J3 7605 from the Slovenian Research Agency (ARRS), CipKeBip, COST Action BM1002, EU COST Action CM1207-GLISTEN. GL's work is supported by the Kathleen Foreman-Casali Foundation and the Beneficentia Stiftung.
Conflict of Interest Statement
The authors declare that the research was conducted in the absence of any commercial or financial relationships that could be construed as a potential conflict of interest.
References
Airan, R. D., Meltzer, L. A., Roy, M., Gong, Y., Chen, H., and Deisseroth, K. (2007). High-speed imaging reveals neurophysiological links to behavior in an animal model of depression. Science 317, 819–823. doi: 10.1126/science.1144400
Altman, J., and Das, G. D. (1965). Autoradiographic and histological evidence of postnatal hippocampal neurogenesis in rats. J. Comp. Neurol. 124, 319–335. doi: 10.1002/cne.901240303
Alzheimer, A. (1907). Über eine eigenartige Erkrankung der Hirnrinde. Versammlung Südwestdeutscher Irrenärzte in Tübingen am 3. November 1906. Allgemeine Zeitschrift für Psychiatrie und Psychisch-Gerichtliche Medizin. 64, 146–148.
Alzheimer, A. (1910). “Beiträge zur Kenntnis der pathologischen Neuroglia und ihrer Beziehungen zu den Abbauvorgängen im Nervengewebe,” in Histologische und histopathologische Arbeiten über die Grosshirnrinde mit besonderer Berücksichtigung der pathologischen Anatomie der Geisteskrankheiten, ed F. Nissl (Jena: Verlag von G. Fischer), 401–562.
Amaral, D. G., and Sinnamon, H. M. (1977). The locus coeruleus: neurobiology of a central noradrenergic nucleus. Prog. Neurobiol. 9, 147–196. doi: 10.1016/0301-0082(77)90016-8
Carmona, M., Escobar, L. A., Torrejón-Escribano, B., Aso, E., et al. (2017). Locus coeruleus at asymptomatic early and middle Braak stages of neurofibrillary tangle pathology. Neuropathol. Appl. Neurobiol. 43, 373–392. doi: 10.1111/nan.12386
Aoki, C. (1992). Beta-adrenergic receptors: astrocytic localization in the adult visual cortex and their relation to catecholamine axon terminals as revealed by electron microscopic immunocytochemistry. J. Neurosci. 12, 781–792. doi: 10.1523/JNEUROSCI.12-03-00781.1992
Arendt, T., Bruckner, M. K., Morawski, M., Jager, C., and Gertz, H. J. (2015). Early neurone loss in Alzheimer's disease: cortical or subcortical? Acta Neuropathol. Commun 3:10. doi: 10.1186/s40478-015-0187-1
Aston-Jones, G., and Cohen, J. D. (2005). An integrative theory of locus coeruleus-norepinephrine function: adaptive gain and optimal performance. Annu. Rev. Neurosci. 28, 403–450. doi: 10.1146/annurev.neuro.28.061604.135709
Aztiria, E., Capodieci, G., Arancio, L., and Leanza, G. (2007). Extensive training in a maze task reduces neurogenesis in the adult rat dentate gyrus probably as a result of stress. Neurosci. Lett. 416, 133–137. doi: 10.1016/j.neulet.2007.01.069
Barros, L. F. (2013). Metabolic signaling by lactate in the brain. Trends Neurosci. 36, 396–404. doi: 10.1016/j.tins.2013.04.002
Barthelemy, I., Martineau, D., Ong, M., Matsunami, R., Ling, N., Benatti, L., et al. (1993). The expression of saporin, a ribosome-inactivating protein from the plant Saponaria officinalis, in Escherichia coli. J. Biol. Chem. 268, 6541–6458.
Beach, T. G., and McGeer, E. G. (1988). Lamina-specific arrangement of astrocytic gliosis and senile plaques in Alzheimer's disease visual cortex. Brain Res. 463, 357–361. doi: 10.1016/0006-8993(88)90410-6
Bekar, L. K., He, W., and Nedergaard, M. (2008). Locus coeruleus alpha-adrenergic-mediated activation of cortical astrocytes in vivo. Cereb. Cortex 18, 2789–2795. doi: 10.1093/cercor/bhn040
Benarroch, E. E. (2009). The locus ceruleus norepinephrine system: functional organization and potential clinical significance. Neurology 73, 1699–1704. doi: 10.1212/WNL.0b013e3181c2937c
Bharani, K. L., Derex, R., Granholm, A. C., and Ledreux, A. (2017). A noradrenergic lesion aggravates the effects of systemic inflammation on the hippocampus of aged rats. PLoS ONE 12:e0189821. doi: 10.1371/journal.pone.0189821
Borodovitsyna, O., Flamini, M., and Chandler, D. (2017). Noradrenergic modulation of cognition in health and disease. Neural Plast. 2017:6031478. doi: 10.1155/2017/6031478
Bouret, S., and Sara, S. J. (2004). Reward expectation, orientation of attention and locus coeruleus-medial frontal cortex interplay during learning. Eur. J. Neurosci. 20, 791–802. doi: 10.1111/j.1460-9568.2004.03526.x
Bouret, S., and Sara, S. J. (2005). Network reset: a simplified overarching theory of locus coeruleus noradrenaline function. Trends Neurosci. 28, 574–582. doi: 10.1016/j.tins.2005.09.002
Braak, H., and Del Tredici, K. (2011a). The pathological process underlying Alzheimer's disease in individuals under thirty. Acta Neuropathol. 121, 171–181. doi: 10.1007/s00401-010-0789-4
Braak, H., and Del Tredici, K. (2011b). Alzheimer's pathogenesis: is there neuron-to-neuron propagation? Acta Neuropathol. 121, 589–595. doi: 10.1007/s00401-011-0825-z
Braak, H., and Del Tredici, K. (2015). The preclinical phase of the pathological process underlying sporadic Alzheimer's disease. Brain 138(Pt 10), 2814–2833. doi: 10.1093/brain/awv236
Braak, H., Thal, D. R., Ghebremedhin, E., and Del Tredici, K. (2011). Stages of the pathologic process in Alzheimer disease: age categories from 1 to 100 years. J. Neuropathol. Exp. Neurol. 70, 960–969. doi: 10.1097/NEN.0b013e318232a379
Braun, D., and Feinstein, D. L. (2017). The locus coeruleus neuroprotective drug vindeburnol normalizes behavior in the 5xFAD transgenic mouse model of Alzheimer's disease. Brain Res. doi: 10.1016/j.brainres.2017.12.028. [Epub ahead of print].
Braun, D., Madrigal, J. L., and Feinstein, D. L. (2014). Noradrenergic regulation of glial activation: molecular mechanisms and therapeutic implications. Curr. Neuropharmacol. 12, 342–352. doi: 10.2174/1570159X12666140828220938
Brezun, J. M., and Daszuta, A. (1999). Depletion in serotonin decreases neurogenesis in the dentate gyrus and the subventricular zone of adult rats. Neuroscience 89, 999–1002. doi: 10.1016/S0306-4522(98)00693-9
Cameron, H. A., Woolley, C. S., McEwen, B. S., and Gould, E. (1993). Differentiation of newly born neurons and glia in the dentate gyrus of the adult rat. Neuroscience 56, 337–344. doi: 10.1016/0306-4522(93)90335-D
Carlen, M., Cassidy, R. M., Brismar, H., Smith, G. A., Enquist, L. W., and Frisen, J. (2002). Functional integration of adult-born neurons. Curr. Biol. 12, 606–608. doi: 10.1016/S0960-9822(02)00771-6
Chandler, D. J., Gao, W. J., and Waterhouse, B. D. (2014). Heterogeneous organization of the locus coeruleus projections to prefrontal and motor cortices. Proc. Natl. Acad. Sci. U.S.A. 111, 6816–6821. doi: 10.1073/pnas.1320827111
Chan-Palay, V., and Asan, E. (1989). Alterations in catecholamine neurons of the locus coeruleus in senile dementia of the Alzheimer type and in Parkinson's disease with and without dementia and depression. J. Comp. Neurol. 287, 373–392. doi: 10.1002/cne.902870308
Chen, Y., Peng, Y., Che, P., Gannon, M., Liu, Y., Li, L., et al. (2014). alpha(2A) adrenergic receptor promotes amyloidogenesis through disrupting APP-SorLA interaction. Proc. Natl. Acad. Sci. U.S.A. 111, 17296–172301. doi: 10.1073/pnas.1409513111
Contestabile, A., and Stirpe, F. (1993). Ribosome-inactivating proteins from plants as agents for suicide transport and immunolesioning in the nervous system. Eur. J. Neurosci. 5, 1292–1301. doi: 10.1111/j.1460-9568.1993.tb00915.x
Coradazzi, M., Gulino, R., Fieramosca, F., Falzacappa, L. V., Riggi, M., and Leanza, G. (2016). Selective noradrenaline depletion impairs working memory and hippocampal neurogenesis. Neurobiol. Aging 48, 93–102. doi: 10.1016/j.neurobiolaging.2016.08.012
Coradazzi, M., Gulino, R., Garozzo, S., and Leanza, G. (2010). Selective lesion of the developing central noradrenergic system: short- and long-term effects and reinnervation by noradrenergic-rich tissue grafts. J. Neurochem. 114, 761–771. doi: 10.1111/j.1471-4159.2010.06800.x
De Keyser, J., Zeinstra, E., Mostert, J., and Wilczak, N. (2004). Beta 2-adrenoceptor involvement in inflammatory demyelination and axonal degeneration in multiple sclerosis. Trends Pharmacol. Sci. 25, 67–71. doi: 10.1016/j.tips.2003.12.002
del Zoppo, G. J. (2009). Inflammation and the neurovascular unit in the setting of focal cerebral ischemia. Neuroscience 158, 972–982. doi: 10.1016/j.neuroscience.2008.08.028
De Strooper, B., and Karran, E. (2016). The cellular phase of Alzheimer's disease. Cell 164, 603–615. doi: 10.1016/j.cell.2015.12.056
Dienel, G. A., and Cruz, N. F. (2016). Aerobic glycolysis during brain activation: adrenergic regulation and influence of norepinephrine on astrocytic metabolism. J. Neurochem. 38, 14–52 doi: 10.1111/jnc.13630
Ding, F., O'Donnell, J., Thrane, A. S., Zeppenfeld, D., Kang, H., Xie, L., et al. (2013). α1-Adrenergic receptors mediate coordinated Ca2+ signaling of cortical astrocytes in awake, behaving mice. Cell Calcium 54, 387–394. doi: 10.1016/j.ceca.2013.09.001
Dong, J. H., Wang, Y. J., Cui, M., Wang, X. J., Zheng, W. S., Ma, M. L., et al. (2017). Adaptive activation of a stress response pathway improves learning and memory through Gs and beta-arrestin-1-regulated lactate metabolism. Biol. Psychiatry 81, 654–670. doi: 10.1016/j.biopsych.2016.09.025
Dranovsky, A., and Hen, R. (2006). Hippocampal neurogenesis: regulation by stress and antidepressants. Biol. Psychiatry 59, 1136–1143. doi: 10.1016/j.biopsych.2006.03.082
Duman, R. S., Malberg, J., and Nakagawa, S. (2001). Regulation of adult neurogenesis by psychotropic drugs and stress. J. Pharmacol. Exp. Ther. 299, 401–407.
Ebrahimi, S., Rashidy-Pour, A., Vafaei, A. A., and Akhavan, M. M. (2010). Central beta-adrenergic receptors play an important role in the enhancing effect of voluntary exercise on learning and memory in rat. Behav. Brain Res. 208, 189–193. doi: 10.1016/j.bbr.2009.11.032
Elrod, R., Peskind, E. R., DiGiacomo, L., Brodkin, K. I., Veith, R. C., and Raskind, M. A. (1997). Effects of Alzheimer's disease severity on cerebrospinal fluid norepinephrine concentration. Am. J. Psychiatry 154, 25–30. doi: 10.1176/ajp.154.1.25
Eriksson, P. S., Perfilieva, E., Bjork-Eriksson, T., Alborn, A. M., Nordborg, C., Peterson, D. A., et al. (1998). Neurogenesis in the adult human hippocampus. Nat. Med. 4, 1313–1317. doi: 10.1038/3305
Feinstein, D. L., Kalinin, S., and Braun, D. (2016). Causes, consequences, and cures for neuroinflammation mediated via the locus coeruleus: noradrenergic signaling system. J. Neurochem. 139(Suppl. 2), 154–178. doi: 10.1111/jnc.13447
Forno, L. S., and Alvord, E. C. Jr. (1971). The pathology of Parkinsonism. Part, I. Some new observations and correlations. Contemp. Neurol. Ser. 8, 119–130.
Fuller, R. W., Hemrick-Luecke, S. K., and Snoddy, H. D. (1994). Effects of duloxetine, an antidepressant drug candidate, on concentrations of monoamines and their metabolites in rats and mice. J. Pharmacol. Exp. Ther. 269, 132–136.
Gannon, M., Che, P., Chen, Y., Jiao, K., Roberson, E. D., and Wang, Q. (2015). Noradrenergic dysfunction in Alzheimer's disease. Front. Neurosci. 9:220. doi: 10.3389/fnins.2015.00220
Gannon, M., and Wang, Q. (2018). Complex noradrenergic dysfunction in Alzheimer's disease: Low norepinephrine input is not always to blame. Brain Res. doi: 10.1016/j.brainres.2018.01.001. [Epub ahead of print].
Gao, V., Suzuki, A., Magistretti, P. J., Lengacher, S., Pollonini, G., Steinman, M. Q., et al. (2016). Astrocytic beta2-adrenergic receptors mediate hippocampal long-term memory consolidation. Proc. Natl. Acad. Sci. U.S.A. 113, 8526–8531. doi: 10.1073/pnas.1605063113
Giaume, C., Kirchhoff, F., Matute, C., Reichenbach, A., and Verkhratsky, A. (2007). Glia: the fulcrum of brain diseases. Cell Death Differ. 14, 1324–1335. doi: 10.1038/sj.cdd.4402144
Gibbs, M. E., Hutchinson, D. S., and Summers, R. J. (2010). Noradrenaline release in the locus coeruleus modulates memory formation and consolidation; roles for α- and β-adrenergic receptors. Neuroscience 170, 1209–1222. doi: 10.1016/j.neuroscience.2010.07.052
Gould, E., Tanapat, P., McEwen, B. S., and Fuchs, E. (1998). Proliferation of granule cell precursors in the dentate gyrus of adult monkeys is diminished by stress. Proc. Natl. Acad. Sci. U.S.A. 95, 3168–3171. doi: 10.1073/pnas.95.6.3168
Griffin, W. S., Stanley, L. C., Ling, C., White, L., MacLeod, V., Perrot, L. J., et al. (1989). Brain interleukin 1 and S-100 immunoreactivity are elevated in Down syndrome and Alzheimer disease. Proc. Natl. Acad. Sci. U.S.A. 86, 7611–7615. doi: 10.1073/pnas.86.19.7611
Grudzien, A., Shaw, P., Weintraub, S., Bigio, E., Mash, D. C., and Mesulam, M. M. (2007). Locus coeruleus neurofibrillary degeneration in aging, mild cognitive impairment and early Alzheimer's disease. Neurobiol. Aging 28, 327–335. doi: 10.1016/j.neurobiolaging.2006.02.007
Hagena, H., Hansen, N., and Manahan-Vaughan, D. (2016). beta-adrenergic control of hippocampal function: subserving the choreography of synaptic information storage and memory. Cereb. Cortex 26, 1349–1364. doi: 10.1093/cercor/bhv330
Haglund, M., Sjöbeck M, M., and Englund, E. (2006). Locus ceruleus degeneration is ubiquitous in Alzheimer's disease: possible implications for diagnosis and treatment. Neuropathology 26, 528–532. doi: 10.1111/j.1440-1789.2006.00725.x
Hammerschmidt, T., Kummer, M. P., Terwel, D., Martinez, A., Gorji, A., Pape, H. C., et al. (2013). Selective loss of noradrenaline exacerbates early cognitive dysfunction and synaptic deficits in APP/PS1 mice. Biol. Psychiatry 73, 454–463. doi: 10.1016/j.biopsych.2012.06.013
Heneka, M. T., Carson, M. J., El Khoury, J., Landreth, G. E., Brosseron, F., Feinstein, D. L., et al. (2015). Neuroinflammation in Alzheimer's disease. Lancet Neurol. 14, 388–405. doi: 10.1016/S1474-4422(15)70016-5
Heneka, M. T., Ramanathan, M., Jacobs, A. H., Dumitrescu-Ozimek, L., Bilkei-Gorzo, A., Debeir, T., et al. (2006). Locus ceruleus degeneration promotes Alzheimer pathogenesis in amyloid precursor protein 23 transgenic mice. J. Neurosci. 26, 1343–1354. doi: 10.1523/JNEUROSCI.4236-05.2006
Heneka, M. T., and Verkhratsky, A. (2010). Neuroglia in neurodegeneration. Brain Res. Rev. 63, 189–211. doi: 10.1016/j.brainresrev.2009.11.004
Herregodts, P., Bruyland, M., De Keyser, J., Solheid, C., Michotte, Y., and Ebinger, G. (1989). Monoaminergic neurotransmitters in Alzheimer's disease. An HPLC study comparing presenile familial and sporadic senile cases. J. Neurol. Sci. 92, 101–116. doi: 10.1016/0022-510X(89)90179-2
Horvat, A., Zorec, R., and Vardjan, N. (2016). Adrenergic stimulation of single rat astrocytes results in distinct temporal changes in intracellular Ca2+ and cAMP-dependent PKA responses. Cell Calcium. 59, 156–163. doi: 10.1016/j.ceca.2016.01.002
Iba, M., McBride, J. D., Guo, J. L., Zhang, B., Trojanowski, J. Q., and Lee, V. M. (2015). Tau pathology spread in PS19 tau transgenic mice following locus coeruleus (LC) injections of synthetic tau fibrils is determined by the LC's afferent and efferent connections. Acta Neuropathol. 130, 349–362. doi: 10.1007/s00401-015-1458-4
Ishii, T. (1966). Distribution of Alzheimer's neurofibrillary changes in the brain stem and hypothalamus of senile dementia. Acta Neuropathol. 6, 181–187. doi: 10.1007/BF00686763
Iversen, L. L., Rossor, M. N., Reynolds, G. P., Hills, R., Roth, M., Mountjoy, C. Q., et al. (1983). Loss of pigmented dopamine-beta-hydroxylase positive cells from locus coeruleus in senile dementia of Alzheimer's type. Neurosci. Lett. 39, 95–100. doi: 10.1016/0304-3940(83)90171-4
Jhaveri, D. J., Mackay, E. W., Hamlin, A. S., Marathe, S. V., Nandam, L. S., Vaidya, V. A., et al. (2010). Norepinephrine directly activates adult hippocampal precursors via beta3-adrenergic receptors. J. Neurosci. 30, 2795–2806. doi: 10.1523/JNEUROSCI.3780-09.2010
Jhaveri, D. J., Nanavaty, I., Prosper, B. W., Marathe, S., Husain, B. F., Kernie, S. G., et al. (2014). Opposing effects of alpha2- and beta-adrenergic receptor stimulation on quiescent neural precursor cell activity and adult hippocampal neurogenesis. PLoS ONE 9:e98736. doi: 10.1371/journal.pone.0098736
Kalaria, R. N., Andorn, A. C., Tabaton, M., Whitehouse, P. J., Harik, S. I., and Unnerstall, J. R. (1989). Adrenergic receptors in aging and Alzheimer's disease: increased beta 2-receptors in prefrontal cortex and hippocampus. J. Neurochem. 53, 1772–17781. doi: 10.1111/j.1471-4159.1989.tb09242.x
Kano, M., and Hashimoto, K. (2009). Synapse elimination in the central nervous system. Curr. Opin. Neurobiol. 19, 154–161. doi: 10.1016/j.conb.2009.05.002
Khakpour-Taleghani, B., Lashgari, R., Motamedi, F., and Naghdi, N. (2009). Effect of reversible inactivation of locus ceruleus on spatial reference and working memory. Neuroscience 158, 1284–1291. doi: 10.1016/j.neuroscience.2008.11.001
Kirischuk, S., Tuschick, S., Verkhratsky, A., and Kettenmann, H. (1996). Calcium signalling in mouse Bergmann glial cells mediated by alpha1-adrenoreceptors and H1 histamine receptors. Eur. J. Neurosci. 8, 1198–1208. doi: 10.1111/j.1460-9568.1996.tb01288.x
Kovacs, G. G., Lee, V. M., and Trojanowski, J. Q. (2017). Protein astrogliopathies in human neurodegenerative diseases and aging. Brain Pathol. 27, 675–690. doi: 10.1111/bpa.12536
Kreft, M., Bak, L. K., Waagepetersen, H. S., and Schousboe, A. (2012). Aspects of astrocyte energy metabolism, amino acid neurotransmitter homoeostasis and metabolic compartmentation. ASN Neuro. 4:e00086. doi: 10.1042/AN20120007
Kulijewicz-Nawrot, M., Verkhratsky, A., Chvátal, A., Syková, E., and Rodríguez, J. J. (2012). Astrocytic cytoskeletal atrophy in the medial prefrontal cortex of a triple transgenic mouse model of Alzheimer's disease. J. Anat. 221, 252–262. doi: 10.1111/j.1469-7580.2012.01536.x
Kulkarni, V. A., Jha, S., and Vaidya, V. A. (2002). Depletion of norepinephrine decreases the proliferation, but does not influence the survival and differentiation, of granule cell progenitors in the adult rat hippocampus. Eur. J. Neurosci. 16, 2008–2012. doi: 10.1046/j.1460-9568.2002.02268.x
Lapiz, M. D., Mateo, Y., Durkin, S., Parker, T., and Marsden, C. A. (2001). Effects of central noradrenaline depletion by the selective neurotoxin DSP-4 on the behaviour of the isolated rat in the elevated plus maze and water maze. Psychopharmacology 155, 251–259. doi: 10.1007/s002130100702
Lappi, D. A., Esch, F. S., Barbieri, L., Stirpe, F., and Soria, M. (1985). Characterization of a Saponaria officinalis seed ribosome-inactivating protein: immunoreactivity and sequence homologies. Biochem. Biophys. Res. Commun. 129, 934–942. doi: 10.1016/0006-291X(85)91981-3
Leverenz, J. B., Miller, M. A., Dobie, D. J., Peskind, E. R., and Raskind, M. A. (2001). Increased alpha 2-adrenergic receptor binding in locus coeruleus projection areas in dementia with Lewy bodies. Neurobiol. Aging 22, 555–561. doi: 10.1016/S0197-4580(01)00221-4
Liddelow, S. A., Guttenplan, K. A., Clarke, L. E., Bennett, F. C., Bohlen, C. J., Schirmer, L., et al. (2017). Neurotoxic reactive astrocytes are induced by activated microglia. Nature 541, 481–487. doi: 10.1038/nature21029
Lim, D., Ronco, V., Grolla, A. A., Verkhratsky, A., and Genazzani, A. A. (2014). Glial calcium signalling in Alzheimer's disease. Rev. Physiol. Biochem. Pharmacol. 167, 45–65. doi: 10.1007/112_2014_19
Lütjohann, D., Breuer, O., Ahlborg, G., Nennesmo, I., Sidén, A., Diczfalusy, U., et al. (1996). Cholesterol homeostasis in human brain: evidence for an age-dependent flux of 24S-hydroxycholesterol from the brain into the circulation. Proc. Natl. Acad. Sci. U.S.A. 93, 9799–9804. doi: 10.1073/pnas.93.18.9799
Mair, R. D., Zhang, Y., Bailey, K. R., Toupin, M. M., and Mair, R. G. (2005). Effects of clonidine in the locus coeruleus on prefrontal- and hippocampal-dependent measures of attention and memory in the rat. Psychopharmacology 181, 280–288. doi: 10.1007/s00213-005-2263-x
Mann, D. M., Lincoln, J., Yates, P. O., Stamp, J. E., and Toper, S. (1980). Changes in the monoamine containing neurones of the human CNS in senile dementia. Br. J. Psychiatry 136, 533–541. doi: 10.1192/bjp.136.6.533
Mann, D. M., Yates, P. O., and Hawkes, J. (1982). The noradrenergic system in Alzheimer and multi-infarct dementias. J. Neurol. Neurosurg. Psychiatry 45, 113–119. doi: 10.1136/jnnp.45.2.113
Marino, M. D., Bourdelat-Parks, B. N., Cameron Liles, L., and Weinshenker, D. (2005). Genetic reduction of noradrenergic function alters social memory and reduces aggression in mice. Behav. Brain Res. 161, 197–203. doi: 10.1016/j.bbr.2005.02.005
Markakis, E. A., and Gage, F. H. (1999). Adult-generated neurons in the dentate gyrus send axonal projections to field CA3 and are surrounded by synaptic vesicles. J. Comp. Neurol. 406, 449–460. doi: 10.1002/(SICI)1096-9861(19990419)406:4<449::AID-CNE3>3.0.CO;2-I
Martignoni, E., Blandini, F., Petraglia, F., Pacchetti, C., Bono, G., and Nappi, G. (1992). Cerebrospinal fluid norepinephrine, 3-methoxy-4-hydroxyphenylglycol and neuropeptide Y levels in Parkinson's disease, multiple system atrophy and dementia of the Alzheimer type. J. Neural Transm. Park. Dis. Dement. Sect. 4, 191–205. doi: 10.1007/BF02260903
Masuda, T., Nakagawa, S., Boku, S., Nishikawa, H., Takamura, N., Kato, A., et al. (2012). Noradrenaline increases neural precursor cells derived from adult rat dentate gyrus through beta2 receptor. Prog. Neuropsychopharmacol. Biol. Psychiatry 36, 44–51. doi: 10.1016/j.pnpbp.2011.08.019
Mather, M., and Harley, C. W. (2016). The locus coeruleus: essential for maintaining cognitive function and the aging brain. Trends Cogn. Sci. 20, 214–226. doi: 10.1016/j.tics.2016.01.001
Matthews, K. L., Chen, C. P., Esiri, M. M., Keene, J., Minger, S. L., and Francis, P. T. (2002). Noradrenergic changes, aggressive behavior, and cognition in patients with dementia. Biol. Psychiatry 51, 407–416. doi: 10.1016/S0006-3223(01)01235-5
Mattson, M. P. (2004). Pathways towards and away from Alzheimer's disease. Nature 430, 631–639. doi: 10.1038/nature02621
Mattson, M. P., and Chan, S. L. (2003). Neuronal and glial calcium signaling in Alzheimer's disease. Cell Calcium 34, 385–397. doi: 10.1016/S0143-4160(03)00128-3
Mauch, D. H., Nägler, K., Schumacher, S., Göritz, C., Müller, E. C., Otto, A., et al. (2001). CNS synaptogenesis promoted by glia-derived cholesterol. Science 294, 1354–1357. doi: 10.1126/science.294.5545.1354
Milstein, J. A., Lehmann, O., Theobald, D. E., Dalley, J. W., and Robbins, T. W. (2007). Selective depletion of cortical noradrenaline by anti-dopamine beta-hydroxylase-saporin impairs attentional function and enhances the effects of guanfacine in the rat. Psychopharmacology 190, 51–63. doi: 10.1007/s00213-006-0594-x
Mohapel, P., Leanza, G., Kokaia, M., and Lindvall, O. (2005). Forebrain acetylcholine regulates adult hippocampal neurogenesis and learning. Neurobiol Aging 26, 939–946. doi: 10.1016/j.neurobiolaging.2004.07.015
Mohapel, P., Mundt-Petersen, K., Brundin, P., and Frielingsdorf, H. (2006). Working memory training decreases hippocampal neurogenesis. Neuroscience 142, 609–613. doi: 10.1016/j.neuroscience.2006.07.033
Mohs, R. C., Shiovitz, T. M., Tariot, P. N., Porsteinsson, A. P., Baker, K. D., and Feldman, P. D. (2009). Atomoxetine augmentation of cholinesterase inhibitor therapy in patients with Alzheimer disease: 6-month, randomized, double-blind, placebo-controlled, parallel-trial study. Am. J. Geriatr. Psychiatry 17, 752–759. doi: 10.1097/JGP.0b013e3181aad585
Monai, H., Ohkura, M., Tanaka, M., Oe, Y., Konno, A., Hirai, H., et al. (2016). Calcium imaging reveals glial involvement in transcranial direct current stimulation-induced plasticity in mouse brain. Nat. Commun. 7:11100. doi: 10.1038/ncomms11100
Mrak, R. E., and Griffin, W. S. (2005). Glia and their cytokines in progression of neurodegeneration. Neurobiol. Aging 26, 349–354. doi: 10.1016/j.neurobiolaging.2004.05.010.
Murchison, C. F., Zhang, X. Y., Zhang, W. P., Ouyang, M., Lee, A., and Thomas, S. A. (2004). A distinct role for norepinephrine in memory retrieval. Cell 117, 131–143. doi: 10.1016/S0092-8674(04)00259-4
Nagele, R. G., Wegiel, J., Venkataraman, V., Imaki, H., and Wang, K. C. (2004). Contribution of glial cells to the development of amyloid plaques in Alzheimer's disease. Neurobiol. Aging 25, 663–674. doi: 10.1016/j.neurobiolaging.2004.01.007
Nazarali, A. J., and Reynolds, G. P. (1992). Monoamine neurotransmitters and their metabolites in brain regions in Alzheimer's disease: a postmortem study. Cell Mol. Neurobiol. 12, 581–587. doi: 10.1007/BF00711237
Nedergaard, M., Rodriguez, J. J., and Verkhratsky, A. (2010). Glial calcium and diseases of the nervous system. Cell Calcium 47, 140–149. doi: 10.1016/j.ceca.2009.11.010
Oe, Y., Baba, O., Ashida, H., Nakamura, K. C., and Hirase, H. (2016). Glycogen distribution in the microwave-fixed mouse brain reveals heterogeneous astrocytic patterns. Glia. doi: 10.1002/glia.23020
Olabarria, M., Noristani, H. N., Verkhratsky, A., and Rodríguez, J. J. (2010). Concomitant astroglial atrophy and astrogliosis in a triple transgenic animal model of Alzheimer's disease. Glia 58, 831–838. doi: 10.1002/glia.20967
Olabarria, M., Noristani, H. N., Verkhratsky, A., and Rodríguez, J. J. (2011). Age-dependent decrease in glutamine synthetase expression in the hippocampal astroglia of the triple transgenic Alzheimer's disease mouse model: mechanism for deficient glutamatergic transmission? Mol. Neurodegener. 6:55. doi: 10.1186/1750-1326-6-55
Pankratov, Y., and Lalo, U. (2015). Role for astroglial alpha1-adrenoreceptors in gliotransmission and control of synaptic plasticity in the neocortex. Front. Cell Neurosci. 9:230. doi: 10.3389/fncel.2015.00230
Parpura, V., Heneka, M. T., Montana, V., Oliet, S. H., Schousboe, A., Haydon, P. G., et al. (2012). Glial cells in (patho)physiology. J. Neurochem. 121, 4–27. doi: 10.1111/j.1471-4159.2012.07664.x
Parpura, V., and Zorec, R. (2010). Gliotransmission: exocytotic release from astrocytes. Brain Res. Rev. 63, 83–92. doi: 10.1016/j.brainresrev.2009.11.008
Pascual, J., Grijalba, B., Garcia-Sevilla, J. A., Zarranz, J. J., and Pazos, A. (1992). Loss of high-affinity alpha 2-adrenoceptors in Alzheimer's disease: an autoradiographic study in frontal cortex and hippocampus. Neurosci. Lett. 142, 36–40. doi: 10.1016/0304-3940(92)90614-D
Paukert, M., Agarwal, A., Cha, J., Doze, V. A., Kang, J. U., and Bergles, D. E. (2014). Norepinephrine controls astroglial responsiveness to local circuit activity. Neuron 82, 1263–1270. doi: 10.1016/j.neuron.2014.04.038
Pekny, M., Pekna, M., Messing, A., Steinhauser, C., Lee, J. M., Parpura, V., et al. (2016). Astrocytes: a central element in neurological diseases. Acta Neuropathol. 131, 323–345. doi: 10.1007/s00401-015-1513-1
Peterson, A. C., and Li, C. R. (2018). Noradrenergic dysfunction in Alzheimer's and Parkinson's Diseases-an overview of imaging studies. Front. Aging Neurosci. 10:127. doi: 10.3389/fnagi.2018.00127
Picklo, M. J., Wiley, R. G., Lappi, D. A., and Robertson, D. (1994). Noradrenergic lesioning with an anti-dopamine beta-hydroxylase immunotoxin. Brain Res. 666, 195–200. doi: 10.1016/0006-8993(94)90772-2
Pintus, R., Riggi, M., Cannarozzo, C., Valeri, A., de Leo, G., Romano, M., et al. (2018). Essential role of hippocampal noradrenaline in the regulation of spatial working memory and TDP-43 tissue pathology. J. Comp. Neurol. 526, 1131–1147. doi: 10.1002/cne.24397
Prebil, M., Vardjan, N., Jensen, J., Zorec, R., and Kreft, M. (2011). Dynamic monitoring of cytosolic glucose in single astrocytes. Glia 59, 903–913. doi: 10.1002/glia.21161
Rodriguez-Vieitez, E., Saint-Aubert, L., Carter, S. F., Almkvist, O., Farid, K., Schöll, M., et al. (2016). Diverging longitudinal changes in astrocytosis and amyloid PET in autosomal dominant Alzheimer's disease. Brain 139(Pt 3):922–936. doi: 10.1093/brain/awv404
Rub, U., Del Tredici, K., Schultz, C., Thal, D. R., Braak, E., and Braak, H. (2001). The autonomic higher order processing nuclei of the lower brain stem are among the early targets of the Alzheimer's disease-related cytoskeletal pathology. Acta Neuropathol. 101, 555–564.
Sacchetti, G., Bernini, M., Bianchetti, A., Parini, S., Invernizzi, R. W., and Samanin, R. (1999). Studies on the acute and chronic effects of reboxetine on extracellular noradrenaline and other monoamines in the rat brain. Br. J. Pharmacol. 128, 1332–1338.
Salcedo-Sora, J. E., Caamano-Gutierrez, E., Ward, S. A., and Biagini, G. A. (2014). The proliferating cell hypothesis: a metabolic framework for Plasmodium growth and development. Trends Parasitol. 30, 170–175. doi: 10.1016/j.pt.2014.02.001
Salm, A. K., and McCarthy, K. D. (1989). Expression of beta-adrenergic receptors by astrocytes isolated from adult rat cortex. Glia 2, 346–352.
Sanchez-Padilla, J., Guzman, J. N., Ilijic, E., Kondapalli, J., Galtieri, D. J., Yang, B., et al. (2014). Mitochondrial oxidant stress in locus coeruleus is regulated by activity and nitric oxide synthase. Nat. Neurosci. 17, 832–840. doi: 10.1038/nn.3717
Santarelli, L., Saxe, M., Gross, C., Surget, A., Battaglia, F., Dulawa, S., et al. (2003). Requirement of hippocampal neurogenesis for the behavioral effects of antidepressants. Science 301, 805–809. doi: 10.1126/science.1083328
Sara, S. J. (2009). The locus coeruleus and noradrenergic modulation of cognition. Nat. Rev. Neurosci. 10, 211–223. doi: 10.1038/nrn2573
Satoh, A., and Iijima, K. M. (2017). Roles of tau pathology in the locus coeruleus (LC) in age-associated pathophysiology and Alzheimer's disease pathogenesis: Potential strategies to protect the LC against aging. Brain Res. doi: 10.1016/j.brainres.2017.12.027. [Epub ahead of print].
Scullion, G. A., Kendall, D. A., Marsden, C. A., Sunter, D., and Pardon, M. C. (2011). Chronic treatment with the alpha2-adrenoceptor antagonist fluparoxan prevents age-related deficits in spatial working memory in APPxPS1 transgenic mice without altering beta-amyloid plaque load or astrocytosis. Neuropharmacology 60, 223–234. doi: 10.1016/j.neuropharm.2010.09.002
Segal, S. K., Stark, S. M., Kattan, D., Stark, C. E., and Yassa, M. A. (2012). Norepinephrine-mediated emotional arousal facilitates subsequent pattern separation. Neurobiol Learn. Mem. 97, 465–469. doi: 10.1016/j.nlm.2012.03.010
Sherpa, A. D., Xiao, F., Joseph, N., Aoki, C., and Hrabetova, S. (2016). Activation of beta-adrenergic receptors in rat visual cortex expands astrocytic processes and reduces extracellular space volume. Synapse 70, 307–316. doi: 10.1002/syn.21908
Shimohama, S., Taniguchi, T., Fujiwara, M., and Kameyama, M. (1986). Biochemical characterization of alpha-adrenergic receptors in human brain and changes in Alzheimer-type dementia. J. Neurochem. 47, 1295–1301.
Singh, C., Liu, L., Wang, J. M., Irwin, R. W., Yao, J., Chen, S., et al. (2012). Allopregnanolone restores hippocampal-dependent learning and memory and neural progenitor survival in aging 3xTgAD and nonTg mice. Neurobiol. Aging 33, 1493–1506. doi: 10.1016/j.neurobiolaging.2011.06.008
Sontag, T. A., Hauser, J., Kaunzinger, I., Gerlach, M., Tucha, O., and Lange, K. W. (2008). Effects of the noradrenergic neurotoxin DSP4 on spatial memory in the rat. J. Neural. Transm. 115, 299–303. doi: 10.1007/s00702-007-0830-5
Sparks, D. L., DeKosky, S. T., and Markesbery, W. R. (1988). Alzheimer's disease. Aminergic-cholinergic alterations in hypothalamus. Arch. Neurol. 45, 994–999.
Starke, K. (2001). Presynaptic autoreceptors in the third decade: focus on alpha2-adrenoceptors. J. Neurochem 78, 685–693.
Stenovec, M., Trkov, S., Lasič, E., Terzieva, S., Kreft, M., Rodríguez Arellano, J. J., et al. (2016). Expression of familial Alzheimer disease presenilin 1 gene attenuates vesicle traffic and reduces peptide secretion in cultured astrocytes devoid of pathologic tissue environment. Glia 64, 317–329. doi: 10.1002/glia.22931
Strassnig, M., and Ganguli, M. (2005). About a peculiar disease of the cerebral cortex: Alzheimer's original case revisited. Psychiatry 2, 30–33.
Studelska, D. R., and Brimijoin, S. (1989). Partial isolation of two classes of dopamine beta-hydroxylase-containing particles undergoing rapid axonal transport in rat sciatic nerve. J. Neurochem. 53, 622–631.
Swanson, L. W., and Hartman, B. K. (1975). The central adrenergic system. An immunofluorescence study of the location of cell bodies and their efferent connections in the rat utilizing dopamine-beta-hydroxylase as a marker. J. Comp. Neurol. 163, 467–505.
Szot, P., Miguelez, C., White, S. S., Franklin, A., Sikkema, C., Wilkinson, C. W., et al. (2010). A comprehensive analysis of the effect of DSP4 on the locus coeruleus noradrenergic system in the rat. Neuroscience 166, 279–291. doi: 10.1016/j.neuroscience.2009
Szot, P., White, S. S., Greenup, J. L., Leverenz, J. B., Peskind, E. R., and Raskind, M. A. (2006). Compensatory changes in the noradrenergic nervous system in the locus ceruleus and hippocampus of postmortem subjects with Alzheimer's disease and dementia with Lewy bodies. J. Neurosci. 26, 467–478. doi: 10.1523/JNEUROSCI.4265-05.2006
Szot, P., White, S. S., Greenup, J. L., Leverenz, J. B., Peskind, E. R., and Raskind, M. A. (2007). Changes in adrenoreceptors in the prefrontal cortex of subjects with dementia: evidence of compensatory changes. Neuroscience 146, 471–480. doi: 10.1016/j.neuroscience.2007.01.031
Tang, F., Lane, S., Korsak, A., Paton, J. F., Gourine, A. V., Kasparov, S., et al. (2014). Lactate-mediated glia-neuronal signalling in the mammalian brain. Nat. Commun. 5:3284. doi: 10.1038/ncomms4284
Terry, R. D. (2000). Cell death or synaptic loss in Alzheimer disease. J. Neuropathol. Exp. Neurol. 59, 1118–1189.
Thomas, S. A., and Palmiter, R. D. (1997). Disruption of the dopamine beta-hydroxylase gene in mice suggests roles for norepinephrine in motor function, learning, and memory. Behav. Neurosci. 111, 579–589.
Thrane, A. S., Rangroo Thrane, V., and Nedergaard, M. (2014). Drowning stars: reassessing the role of astrocytes in brain edema. Trends Neurosci. 37, 620–628. doi: 10.1016/j.tins.2014.08.010
Tohgi, H., Ueno, M., Abe, T., Takahashi, S., and Nozaki, Y. (1992). Concentrations of monoamines and their metabolites in the cerebrospinal fluid from patients with senile dementia of the Alzheimer type and vascular dementia of the Binswanger type. J. Neural Transm. Par.k Dis. Dement. Sect. 4, 69–77.
Tomlinson, B. E., Irving, D., and Blessed, G. (1981). Cell loss in the locus coeruleus in senile dementia of Alzheimer type. J. Neurol Sci. 49, 419–428.
Van Dyck, C. H. (2014). Guanfacine Treatment for Prefrontal Cognitive Dysfunction in Elderly Subjects. Available online at: https://clinicaltrials.gov/ct2/show/NCT00935493
Vander Heiden, M. G., Cantley, L. C., and Thompson, C. B. (2009). Understanding the Warburg effect: the metabolic requirements of cell proliferation. Science 324, 1029–1033. doi: 10.1126/science.1160809
Van Hoomissen, J. D., Holmes, P. V., Zellner, A. S., Poudevigne, A., and Dishman, R. K. (2004). Effects of beta-adrenoreceptor blockade during chronic exercise on contextual fear conditioning and mRNA for galanin and brain-derived neurotrophic factor. Behav. Neurosci. 118, 1378–1390.
van Praag, H., Schinder, A. F., Christie, B. R., Toni, N., Palmer, T. D., and Gage, F. H. (2002). Functional neurogenesis in the adult hippocampus. Nature 415, 1030–1034. doi: 10.1038/4151030a
Vardjan, N., Horvat, A., Anderson, J. E., Yu, D., Croom, D., Zeng, X., et al. (2016). Adrenergic activation attenuates astrocyte swelling induced by hypotonicity and neurotrauma. Glia 64, 1034–1049. doi: 10.1002/glia.22981
Vardjan, N., Kreft, M., and Zorec, R. (2014). Dynamics of β-adrenergic/cAMP signaling and morphological changes in cultured astrocytes. Glia.
Vardjan, N., and Zorec, R. (2015). Excitable astrocytes: Ca2+- and cAMP-regulated exocytosis. Neurochem. Res. 40, 2414–2414. doi: 10.1007/s11064-015-1545-x
Verkhratsky, A., Marutle, A., Rodríguez-Arellano, J. J., and Nordberg, A. (2014). Glial Asthenia and Functional Paralysis: a new perspective on neurodegeneration and Alzheimer's disease. Neuroscientist 21, 552–568. doi: 10.1177/1073858414547132
Verkhratsky, A., and Nedergaard, M. (2014). Astroglial cradle in the life of the synapse. Philos. Trans. R. Soc. Lond. B. Biol. Sci. 369:20130595. doi: 10.1098/rstb.2013.0595
Verkhratsky, A., and Nedergaard, M. (2018). Physiology of Astroglia. Physiol. Rev. 98, 239–389. doi: 10.1152/physrev.00042.2016
Verkhratsky, A., and Parpura, V. (2015). Astrogliopathology in neurological, neurodevelopmental and psychiatric disorders. Neurobiol. Dis. 85, 254–261. doi: 10.1016/j.nbd.2015.03.025
Verkhratsky, A., Zorec, R., Rodriguez, J. J., and Parpura, V. (2016). Astroglia dynamics in ageing and Alzheimer's disease. Curr. Opin. Pharmacol. 26, 74–79. doi: 10.1016/j.coph.2015.09.011
Walker, T. L., White, A., Black, D. M., Wallace, R. H., Sah, P., and Bartlett, P. F. (2008). Latent stem and progenitor cells in the hippocampus are activated by neural excitation. J. Neurosci. 28, 5240–5247. doi: 10.1523/JNEUROSCI.0344-08.2008
Warner-Schmidt, J. L., and Duman, R. S. (2006). Hippocampal neurogenesis: opposing effects of stress and antidepressant treatment. Hippocampus 16, 239–249. doi: 10.1002/hipo.20156
Whitehouse, P. J., Price, D. L., Struble, R. G., Clark, A. W., Coyle, J. T., and Delon, M. R. (1982). Alzheimer's disease and senile dementia: loss of neurons in the basal forebrain. Science 215, 1237–1239.
Wiley, R. G., and Kline, I. R. (2000). Neuronal lesioning with axonally transported toxins. J. Neurosci. Methods 103, 73–82
Wilson, R. S., Nag, S., Boyle, P. A., Hizel, L. P., Yu, L., Buchman, A. S., et al. (2013). Neural reserve, neuronal density in the locus ceruleus, and cognitive decline. Neurology 80, 1202–1208. doi: 10.1212/WNL.0b013e3182897103
Woodruff-Pak, D. S. (2008). Animal models of Alzheimer's disease: therapeutic implications. J. Alzheimers. Dis. 15, 507–521.
Wrenn, C. C., Picklo, M. J., Lappi, D. A., Robertson, D., and Wiley, R. G. (1996). Central noradrenergic lesioning using anti-DBH-saporin: anatomical findings. Brain Res. 740, 175–184.
Yeh, C. Y., Vadhwana, B., Verkhratsky, A., and Rodríguez, J. J. (2011). Early astrocytic atrophy in the entorhinal cortex of a triple transgenic animal model of Alzheimer's disease. ASN Neuro 3, 271–279. doi: 10.1042/AN20110025
Zorec, R., Horvat, A., Vardjan, N., and Verkhratsky, A. (2015). Memory Formation Shaped by Astroglia. Front. Integr. Neurosci. 9:56. doi: 10.3389/fnint.2015.00056
Keywords: noradrenaline (norepinephrine), cognitive decline, Alzheimer's disease, neurodegeneration, neurogenesis, astroglia
Citation: Leanza G, Gulino R and Zorec R (2018) Noradrenergic Hypothesis Linking Neurodegeneration-Based Cognitive Decline and Astroglia. Front. Mol. Neurosci. 11:254. doi: 10.3389/fnmol.2018.00254
Received: 21 March 2018; Accepted: 05 July 2018;
Published: 27 July 2018.
Edited by:
Margaret Su-chun Ho, ShanghaiTech University, ChinaReviewed by:
Claudio Liguori, Università degli Studi di Roma Tor Vergata, ItalyWolfgang Härtig, Leipzig University, Germany
Copyright © 2018 Leanza, Gulino and Zorec. This is an open-access article distributed under the terms of the Creative Commons Attribution License (CC BY). The use, distribution or reproduction in other forums is permitted, provided the original author(s) and the copyright owner(s) are credited and that the original publication in this journal is cited, in accordance with accepted academic practice. No use, distribution or reproduction is permitted which does not comply with these terms.
*Correspondence: Giampiero Leanza, gleanza@units.it
Robert Zorec, robert.zorec@mf.uni-lj.si