Tryptophan Metabolism and COVID-19-Induced Skeletal Muscle Damage: Is ACE2 a Key Regulator?
- Department of Geriatric and General Medicine, Osaka University Graduate School of Medicine, Osaka, Japan
The severity of coronavirus disease 2019 (COVID-19) is characterized by systemic damage to organs, including skeletal muscle, due to excessive secretion of inflammatory cytokines. Clinical studies have suggested that the kynurenine pathway of tryptophan metabolism is selectively enhanced in patients with severe COVID-19. In addition to acting as a receptor for severe acute respiratory syndrome coronavirus 2, the causative virus of COVID-19, angiotensin converting enzyme 2 (ACE2) contributes to tryptophan absorption and inhibition of the renin-angiotensin system. In this article, we review previous studies to assess the potential for a link between tryptophan metabolism, ACE2, and skeletal muscle damage in patients with COVID-19.
Introduction
The global spread of coronavirus disease 2019 (COVID-19) is a serious ongoing issue owing to the high infectiousness of the causative virus, severe acute respiratory syndrome coronavirus 2 (SARS-CoV2), and encroaching resistance to vaccine therapy due to viral mutations. One of the serious challenges of this disease is that some patients who become seriously ill, ultimately develop fatal acute respiratory distress syndrome (ARDS). The prognostic factors are not thoroughly elucidated, but clinical studies have shown that the disease is more severe in men compared to women, specifically in the elderly, and depends on underlying lifestyle-associated chronic diseases, including diabetes and hypertension. Initial symptoms resemble common influenza symptoms. If severe pneumonia develops, symptoms of ARDS can appear, with increased blood levels of pro-inflammatory cytokines including IL-6, IL-10, and TNF-α, and decreased frequency and abnormal functionality of T lymphocytes (1–3). Generation of an excessive inflammatory response, called a cytokine storm, is a probable molecular mechanism underlying the development of ARDS (4–6). In skeletal muscle, myalgia and increased levels of creatine kinase, together with signs of skeletal muscle damage are more pronounced in critically ill patients at higher risk of mortality (7–9). Examination of postmortem skeletal muscle specimens from critically ill patients revealed an inflammatory signature, independent of direct viral effects (10), suggesting that excessive immune activation throughout the body leads to organ damage in severe disease scenarios. It is of considerable interest to note that interferon (IFN) type I reactivity, which is normally elevated upon viral infection in COVID-19 patients, is attenuated with increasing severity, in contrast to IL-6, IL-10, and TNF-α. One possible mechanism could be the presence of proteins with strong IFN1 suppressive activity in SARS-CoV2, including ORF3b and ORF6 (11, 12).
Changes in Tryptophan Metabolism in Patients With Severe COVID-19
Metabolomics analyses of serum samples from COVID-19 patients have revealed the involvement of metabolites of the kynurenine pathway (responsible for metabolism of the essential amino acid tryptophan) in the immune response to SARS-CoV2 infection (13, 14). Previous studies have shown decreased serum tryptophan levels, and increased levels of intermediate metabolites [kynurenine (KYN) and kynurenic acid (KYNA)] in patients with severe COVID-19 compared to healthy controls (13, 14). These differences in KYN and KYNA levels, as well as the reactivity to these metabolites, have been suggested to be contributing factors to the enhanced severity of COVID-19 in male patients (14). In males, the ratio of KYNA to KYN (KA:K) is elevated in patients with severe disease, and KA:K positively correlates with blood levels of IL-6. In females, KA:K does not correlate with disease severity or particular cytokine levels (14), indicating that biological responses to KYN and KYNA are important in determining the prognosis of COVID-19.
Dietary tryptophan absorption occurs mostly in the small intestine, whereas tryptophan metabolism occurs mainly in the liver and kidneys. Approximately 95% of absorbed tryptophan is metabolized by the kynurenine pathway, and the rate-limiting enzymes are tryptophan-2, 3-dioxygenase (TDO) and indoleamine-2, 3-dioxygenase 1/2 (IDO1/2), which metabolize tryptophan to KYN. Under physiological conditions, TDO, which is mainly expressed in the liver, is regulated by hormones including cortisol, insulin, glucagon, and epinephrine (15), while IDO1, which is expressed in monocytes and dendritic cells in organs other than the liver, is activated by pro-inflammatory cytokines such as IFN-γ, IL-6, and TNF-α. The recently identified IDO2 gene, which displays 45% homology with IDO1, is constitutively expressed in the brain, liver, kidney, and epididymis. However, its tryptophan metabolic activity is thought to be lower than that of IDO1 (16). According to previous studies, blood levels of serotonin, a kynurenine pathway-independent metabolite of tryptophan, were lower in patients with severe COVID-19 compared to healthy controls (13), indicating that tryptophan utilization during SARS-CoV2 infection is specifically facilitated by the kynurenine pathway.
Immunomodulatory Mechanisms Mediated by AhR Activation by IDO1 and Tryptophan Metabolites
IDO1 and its kynurenine pathway metabolites have been actively studied as immune regulators because of their involvement in fetal-maternal immune tolerance (17). In cancer immunity, IDO1 and TDO, which are highly expressed in various tumors, including colon and gastric cancers, are thought to be involved in the pathogenesis of immune tolerance in cancer cells (18–20). These cells evade the immune system by activating IDO1, which suppresses T cell proliferation by depleting tryptophan in their microenvironment, and suppresses differentiation of naive CD4+ T cells into regulatory T (Treg)cells via the immunosuppressive properties of tryptophan metabolites (21–26). This immunosuppressive effect of IDO1 has also been observed in pulmonary inflammatory diseases, where its activity increased in response to elevated IFNs in lung epithelial cells during microbial infection. In this regard, the KYN-AhR (aryl hydrocarbon receptor) pathway is thought to contribute to the immunosuppressive function of IDO1 (27). KYN and KYNA are ligands of AhR, a receptor-type transcription factor activated by cyclic aromatic hydrocarbons (28). KYNA, which is generally thought to contribute to immunosuppressive functions by regulating cytokine release from invariant natural killer T cells through G protein-coupled receptor 35 (GPR35) activation (29), has been reported to promote IL-6 secretion in experimental breast cancer cells by binding to AhR (30). Since IL-6 is an immunomodulator involved in both inflammatory and anti-inflammatory functions (31), it remains unclear whether the binding of KYNA to AhR contributes to the promotion or suppression of immune responses. It has been shown that physiological concentrations of KYN promote Treg differentiation from naive T cells in an AhR-dependent manner in vitro during inflammation (26). Tregs, including Tr1 cells, CD8+ Tregs, and FoxP3+ Tregs, are involved in immunosuppression through secretion of TGF-β and IL-10. In contrast, Th17 cells, which differentiate in the presence of TGF-β, IL-6, or IL-21 (31, 32), are known to be involved in tissue inflammation by secreting IL-17A, IL-17F, IL-21, IL-22, and TNF-α. Although Treg and Th17 cells have contradictory functions, they share a requirement for TGF-β for differentiation. AhR agonism may also play a role in promoting differentiation of both cell types (33, 34), where TGF-β stimulation increases AhR expression, which in turn increases ligand-binding activity (26). Thus, Tregs and Th17 cells are known to have plasticity and the ability to display similar traits to each other under the influence of cytokines and other factors (35).
AhR is involved in regulating helper T cell differentiation and macrophage activation, and is therefore involved in both acquired and innate immunity. Its ligands include dioxin and tryptophan metabolites (36). Dioxin is known to promote the generation of Tregs by activating AhR (34). On the other hand, 6-formylindolo[3,2-b]carbazole (FICZ), a compound produced by UV irradiation of tryptophan, and also an AhR agonist and tryptophan metabolite, has been reported to promote the generation of Th17 cells (26, 33). These reports indicate that the immunosuppressive response to AhR agonism can produce different results, depending on the type of ligand (Figure 1A). One possible reason is that the ligands themselves undergo AhR-induced enzymatic modification by cytochrome P450, details of which remain unclear (26).
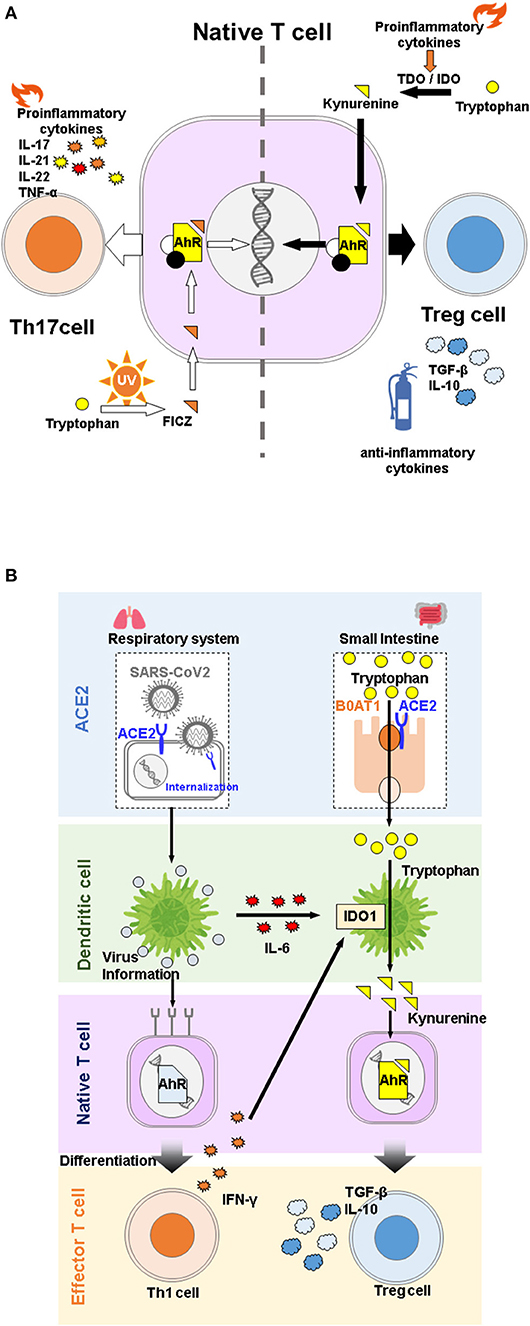
Figure 1. (A) Effects of different tryptophan metabolites on differentiation of native T cells via. aryl hydrocarbon receptor (AhR) activation. Despite belonging to the same pathway, 6-Formylindolo[3,2-b]carbazole (FICZ) promotes differentiation of native T cells into Th17 cells, and induces inflammation, while kynurenine promotes differentiation into Treg cells and functions as an anti-inflammatory agent. (B) Overview of angiotensin converting enzyme 2 (ACE2) physiological functions during COVID-19 infection. SARS-CoV2 infection stimulates secretion of proinflammatory cytokines, which in turn activates IDO1 and promotes the immunosuppressive system through kynurenine binding to AhR. ACE2 is a receptor for SARS-CoV2 but is also involved in immune regulation through tryptophan absorption.
In the case of innate immunity, increased production of type I IFN in IDO1-deficient mice infected with murine leukemia virus or wild type mice treated with IDO inhibitors (37) and suppression of type I IFN secretion in the presence of AhR in vitro (36) suggest that the metabolites produced by IDO1 suppress type I IFN secretion via AhR activation, but the ligand specificity is still unclear. Therefore, in addition to the ORF gene sequence in SARS-CoV2, AhR activation by KYN may be involved in type I IFN suppression in patients with severe COVID-19 (38), suggesting that activation of the IDO1-KYN-AhR pathway promotes mucus secretion in alveolar epithelial cells and induces hypoxia in patients with COVID-19 (39). However, during the cytokine storm observed in severe COVID-19, KYN mediated AhR activation is considered to be an essential biological response to suppress systemic organ damage (13, 40), as is mucus secretion in terms of infection defense. Therefore, AhR activation by IDO1-derived tryptophan metabolites in patients with severe COVID-19 can be regarded as a biological defense mechanism against severe infection (13).
Tryptophan metabolites produced by catabolism in gut microbiota are thought to mediate immunomodulatory functions via activation of AhR, KYN, and KYNA (41–44). Data from mouse models and patients with Inflammatory Bowel Disease (IBD) suggest that AhR activation exerts a preventive effect on the development of colitis (45–48). A possible mechanism underlying this phenomenon is that AhR activation by tryptophan metabolites promotes the differentiation of native T cells in the intestinal epithelium into CD4+CD8α+ T-cells, which are responsible for the anti-inflammatory effects complementary to Tregs (46). On the other hand, IL-22, a cytokine secreted by innate lymphoid cells upon infection with pathogenic bacteria enhances defense against bacterial infection by producing mucus and antimicrobial proteins in intestinal epithelial cells. Tryptophan metabolites produced by intestinal microflora during induction of colitis by bacterial infection in mice are known to play a protective role in the pathogenesis of the disease through AhR activation, which enhances IL-22 secretion (49, 50). Additionally, decreased tryptophan catabolism in the host alters the intestinal microflora and consequently alters the tryptophan metabolites produced by the microflora, thereby promoting differentiation of naive T cells into IL-22-producing cells (50).
ACE2 and Tryptophan Metabolism in COVID-19
SARS-CoV2 targets host cells by binding of the receptor-binding domain of the spike protein expressed on its surface to angiotensin-converting enzyme 2 (ACE2) on the host cell surface. ACE2 is located on the X chromosome and is expressed throughout the body, including the heart, blood vessels, lungs, kidneys, and brain, but predominantly in the small intestine (51–54). As suggested by its name, ACE2 was identified as the Angiotensin converting enzyme (ACE) homologue in renin-angiotensin system (RAS). It cleaves the vasoactive peptide angiotensin II to angiotensin 1-7 that has been reported to protect against the pathological effects of RAS activation in multiple organs (55, 56). Interestingly, ACE2 is involved in the absorption of neutral amino acids, particularly tryptophan, in the small intestine as a chaperone of the amino acid transporter, B0AT1 (57, 58). Indeed, reduced tryptophan absorption causes a decrease in brain serotonin (59) or impaired gut microbial ecology (57) in ACE2 knockout mice. Changes in intestinal microbiota in ACE2 knockout mice may also cause changes in tryptophan metabolites of intestinal bacteria, potentially affecting the regulation of immune responses by AhR activation (50).
Interestingly, several studies have reported that tryptophan metabolites regulate ACE2 expression via AhR (60, 61). Therefore, reduction in membrane-bound ACE2 potentially reduces risk of COVID-19. ACE2 is an organ-protective molecule that has been reported to protect against severe infectious diseases, including ARDS (62). In COVID-19, it is assumed that the expression of membrane-bound ACE2 is reduced by mechanisms such as internalization of SARS-CoV2 upon intracellular entry and cleavage to its soluble form by activation of TNF-α converting enzyme due to inflammation (63). Indeed, recent studies have suggested that the plasma concentration or activity of soluble ACE2 is associated with COVID-19 severity (64–66). Therefore, a vicious cycle is created by viral consumption of ACE2, which accelerates the development of COVID-19. Taken together, these results suggest that ACE2 involvement in tryptophan metabolism is specifically associated with COVID-19 pathogenesis in a complex manner (Figure 1B).
Role of Tryptophan Metabolism and ACE2 in Aging
Tryptophan metabolism and activation of the kynurenine pathway are closely associated with aging (67). Blood tryptophan levels are reported to be lower in older individuals with frailty and cognitive impairment compared to healthy controls (68). Metabolites in the kynurenine pathway are also increased in subjects with frailty (69). Tryptophan metabolism is regarded as the de novo synthesis pathway of nicotinamide adenine dinucleotide (NAD+), which decreases in various organs with aging and is involved in the pathogenesis of diseases including obesity, diabetes, and Alzheimer's disease through energy metabolism and decreased sirtuin function (70). A recent study suggested that inhibition of an enzyme that negatively regulates this pathway enhances mitochondrial function and improves mammalian health (71). Deletion of ACE2 inhibits the promotion of aging phenotypes, including age-related muscle loss (sarcopenia) and skin atrophy, which is accompanied by enhanced p16INK4a (a senescence marker in mice) expression (72, 73). It is conceivable that the, overactivation of RAS does not contribute to the early aging phenotypes in ACE2 knockout mice. This is supported by the findings that both Mas (angiotensin 1-7 receptor) knockout and mice producing excessive angiotensin II by carrying both human renin and angiotensinogen genes did not exhibit the same aging phenotype as ACE2 knockout mice (74).
Tryptophan Metabolism and Muscle Function
Muscle protein anabolism is promoted by increased amino acid uptake in response to increased blood amino acid levels (75). Recent studies have shown that there is a significant difference in blood tryptophan concentration between frail and healthy elderly individuals. However, the results of metabolomic analysis in blood have been inconsistent (68, 76, 77), which could be attributed to several factors, including differences in the criteria used to select a frail study population, subject backgrounds, and experimental protocol details, including sample collection time, storage method, and measurement protocol. In contrast, metabolomic analysis of muscles has shown that frail elderly people have lower tryptophan levels than healthy elderly people, and exercise intervention enhances these levels (78). In this regard, the large neutral amino acid transporter 1, which is responsible for the uptake of tryptophan and kynurenine into skeletal muscle, has been shown to increase in response to acute resistance exercise in young and elderly individuals (79). Since expression of TDO and IDO is almost absent in skeletal muscle (80), kynurenine produced in the liver and other organs is considered to be the starting point of the kynurenine pathway in skeletal muscle. In contrast, kynurenine aminotransferase (KAT), the enzyme responsible for conversion of KYN to KYNA, is highly expressed in skeletal muscle (80), and its expression is increased by endurance training, resulting in increased circulating KYNA levels (81–83). KYN passes through the blood-brain barrier (BBB) and is involved in the pathogenesis of depression and schizophrenia. However, since KYNA does not pass through the BBB, the conversion of KYN to KYNA in skeletal muscle is thought to have neuroprotective effects (84). Studies in animal models have shown conflicting results on the effects of tryptophan and kynurenine on skeletal muscle. Administration of kynurenine to young mice induced oxidative stress along with increased lipid peroxides, and reduction in the size of muscle fibers (85). In contrast, the diameter of fibers in the tibialis anterior muscle of C57BL/6 mice fed a tryptophan-deficient diet was smaller than that of mice fed a standard diet, and blood myostatin (which promotes skeletal muscle breakdown) levels were increased (86). Thus elevated blood kynurenine levels may contribute to skeletal muscle atrophy, while an increase in blood tryptophan may contribute to skeletal muscle maintenance by inhibiting muscle degradation. The skeletal muscle damage observed in patients with COVID-19 may be due to various factors, such as the effect of cytokine storm or disuse due to inactivity (87). However, further investigation on the possible role of altered tryptophan metabolism in the pathogenesis of COVID-19 is warranted (Figure 2A).
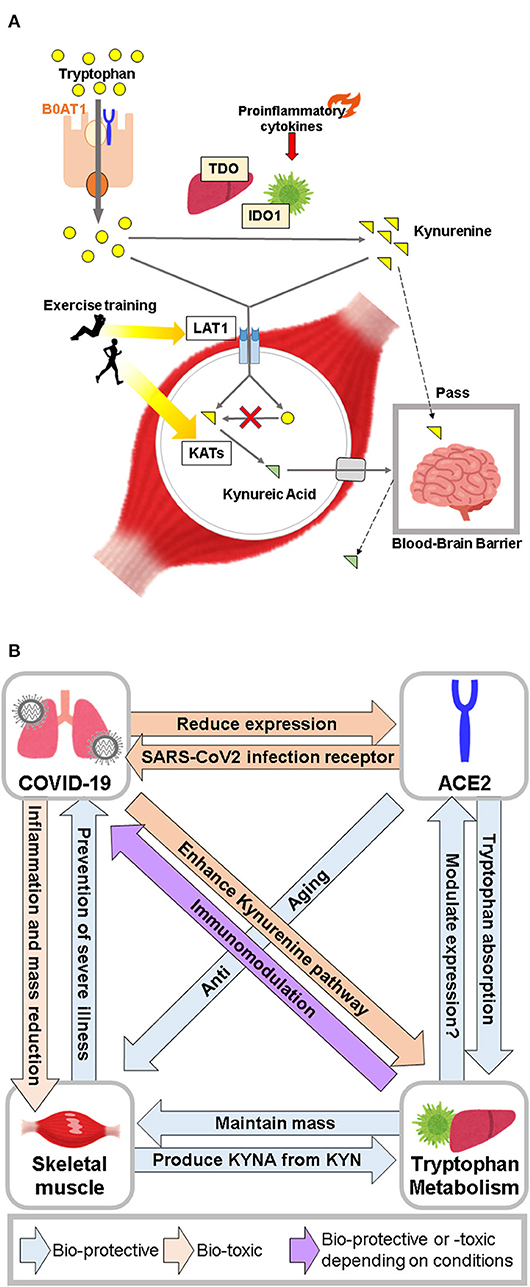
Figure 2. (A) Major role of skeletal muscle in tryptophan metabolism. Skeletal muscle takes up tryptophan and kynurenine, but does not metabolize kynurenine from tryptophan due to the absence of TDO and IDO. Skeletal muscle plays a neuroprotective role by converting kynurenine to kynurenic acid. (B) Conceptual diagram of the relationship between COVID-19, ACE2, tryptophan metabolism, and skeletal muscle.
Perspectives
From a clinical perspective, it is important to investigate the utility of tryptophan supplementation for promotion of human health, particularly in the elderly, who are at high risk from COVID-19. Supplementation of tryptophan (a precursor of serotonin and melatonin) is widely used to improve mood and emotional functioning, and to treat insomnia (88). Studies in animal models have shown that a tryptophan-restricted diet is capable of delaying reproductive aging (89) and extending lifespan in female rats (90, 91). However, the effects of tryptophan depletion on quality of life at older ages remains unknown (92). Given the pro-aging phenotypes of ACE2 knockout mice independent of RAS, it is worth clarifying whether ACE2-mediated absorption of tryptophan provides protection from aging. In the case of inflammatory diseases, a high-tryptophan diet has been shown to reduce gut inflammation and severity of colitis (93). Conversely, perturbation of the intestinal microbiota also affects tryptophan metabolism. Probiotic supplements are capable of reducing upper respiratory tract infections accompanied by the attenuation of exercise-induced tryptophan degradation rates in trained athletes (94). Furthermore, AhR activation by tryptophan metabolites can increase or decrease ACE2 expression as mentioned earlier. In humans as well as mice, tryptophan supplementation has been found to elevate plasma levels of tryptophan metabolites, KYN, and KYNA (95) therefore, it is clinically relevant to understand the role of tryptophan supplementation on organ ACE2 levels, and its relation to COVID-19 susceptibility.
Conclusion
In patients with severe COVID-19, activation of the kynurenine pathway by various cytokines, and tryptophan metabolites regulate immune responses through AhR and GPR35 activation. In contrast, ACE2, which is the primary receptor for SARS-CoV2 infection and has an anti-aging function, is involved in absorption of tryptophan from the small intestine. Furthermore, tryptophan metabolites regulate expression of ACE2 via AhR. These data suggest that ACE2 serves as a gateway to SARS-CoV2 infection, and may also play an immunomodulatory role in the pathogenesis of COVID-19 through mutual regulation of tryptophan metabolites. Furthermore, skeletal muscle may contribute to immunoregulatory mechanisms mediated via tryptophan metabolites by storing tryptophan and producing KYNA (Figure 2B).Collectively, tryptophan metabolism, ACE2, and skeletal muscle are closely linked to the severity of COVID-19. Further studies are needed to elucidate the role of tryptophan supplementation in the maintenance of human health in relation to its effect on ACE2 and skeletal muscle in the COVID-19 era.
Author Contributions
HT and KY were equally responsible for conceptualization, drafting, writing, and editing. All authors contributed to the article and approved the submitted version.
Conflict of Interest
The authors declare that the research was conducted in the absence of any commercial or financial relationships that could be construed as a potential conflict of interest.
Publisher's Note
All claims expressed in this article are solely those of the authors and do not necessarily represent those of their affiliated organizations, or those of the publisher, the editors and the reviewers. Any product that may be evaluated in this article, or claim that may be made by its manufacturer, is not guaranteed or endorsed by the publisher.
Abbreviations
ACE2, angiotensin converting enzyme 2; ARDS, acute respiratory distress syndrome; AhR, aryl hydrocarbon receptor; BBB, blood-brain barrier; COVID-19, coronavirus disease 2019; FICZ, 6-formylindolo[3,2-b]carbazole; GPR35, G protein-coupled receptor 35; IDO, indoleamine-2, 3-dioxygenase; KA:K, KYNA to KYN; KAT, kynurenine aminotransferase; KYN, kynurenine; KYNA, kynurenic acid; NAD+, nicotinamide adenine dinucleotide; RAS, renin-angiotensin system; SARS-CoV2, severe acute respiratory syndrome coronavirus 2; TDO, tryptophan-2, 3-dioxygenase; Treg, regulatory T.
References
1. Chen G, Wu D, Guo W, Cao Y, Huang D, Wang HW, et al. Clinical and immunological features of severe and moderate coronavirus disease 2019. J Clin Invest. (2020) 130:2620–9. doi: 10.1172/JCI137244
2. Huang CL, Wang YM, Li XW, Ren LL, Zhao JP, Hu Y, et al. Clinical features of patients infected with 2019 novel coronavirus in Wuhan, China. Lancet. (2020) 395:497–506. doi: 10.1016/S0140-6736(20)30183-5
3. Chen NS, Zhou M, Dong X, Qu JM, Gong FY, Han Y, et al. Epidemiological and clinical characteristics of 99 cases of 2019 novel coronavirus pneumonia in Wuhan, China: a descriptive study. Lancet. (2020) 395:507–13. doi: 10.1016/S0140-6736(20)30211-7
4. Mehta P, McAuley DF, Brown M, Sanchez E, Tattersall RS, Manson JJ, et al. Covid-19: consider cytokine storm syndromes and immunosuppression. Lancet. (2020) 395:1033–4. doi: 10.1016/S0140-6736(20)30628-0
5. Chen XH, Zhao BH, Qu YM, Chen YR, Xiong J, Feng Y, et al. Detectable serum severe acute respiratory syndrome coronavirus 2 viral load (Rnaemia) is closely correlated with drastically elevated interleukin 6 level in critically ill patients with coronavirus disease 2019. Clin Infect Dis. (2020) 71:1937–42. doi: 10.1093/cid/ciaa449
6. Giamarellos-Bourboulis EJ, Netea MG, Rovina N, Akinosoglou K, Antoniadou A, Antonakos N, et al. Complex immune dysregulation in covid-19 patients with severe respiratory failure. Cell Host Microbe. (2020) 27:992. doi: 10.1016/j.chom.2020.04.009
7. Mao L, Jin HJ, Wang MD, Hu Y, Chen SC, He QW, et al. Neurologic manifestations of hospitalized patients with coronavirus disease 2019 in Wuhan, China. JAMA Neurol. (2020) 77:683–90. doi: 10.1001/jamaneurol.2020.1127
8. Wang DW, Hu B, Hu C, Zhu FF, Liu X, Zhang J, et al. Clinical characteristics of 138 hospitalized patients with 2019 novel coronavirus-infected pneumonia in Wuhan, China. JAMA. (2020) 323:1061–9. doi: 10.1001/jama.2020.1585
9. Zhou F, Yu T, Du RH, Fan GH, Liu Y, Liu ZB, et al. Clinical course and risk factors for mortality of adult inpatients with Covid-19 in Wuhan, China: a retrospective cohort study. Lancet. (2020) 395:1054–62. doi: 10.1016/S0140-6736(20)30566-3
10. Aschman T, Schneider J, Greuel S. Association between Sars-Cov-2 infection and immune-mediated myopathy in patients who have died. JAMA Neurol. (2021) 78:1021. doi: 10.1001/jamaneurol.2021.2004
11. Konno Y, Kimura I, Uriu K, Fukushi M, Irie T, Koyanagi Y, et al. Sars-Cov-2 Orf3b is a potent interferon antagonist whose activity is increased by a naturally occurring elongation variant. Cell Rep. (2020) 32:108185. doi: 10.1016/j.celrep.2020.108185
12. Kimura I, Konno Y, Uriu K, Hopfensperger K, Sauter D, Nakagawa S, et al. Sarbecovirus Orf6 proteins hamper induction of interferon signaling. Cell Rep. (2021) 34:108916. doi: 10.1016/j.celrep.2021.108916
13. Thomas T, Stefanoni D, Reisz JA, Nemkov T, Bertolone L, Francis RO, et al. Covid-19 infection alters kynurenine and fatty acid metabolism, correlating with il-6 levels and renal status. Jci Insight. (2020) 5:140327. doi: 10.1172/jci.insight.140327
14. Cai YP, Kim DJ, Takahashi T, Broadhurst DI, Yan H, Ma SG, et al. Kynurenic acid may underlie sex-specific immune responses to covid-19. Sci Signal. (2021) 14:eabf8483. doi: 10.1126/scisignal.abf8483
15. Isenovic ER, Zakula Z, Koricanac G, Ribarac-Stepic N. Comparative analysis of tryptophan oxygenase activity and glucocorticoid receptor under the influence of insulin. Physiol Res. (2008) 57:101–7. doi: 10.33549/physiolres.931135
16. Fukunaga M, Yamamoto Y, Kawasoe M, Arioka Y, Murakami Y, Hoshi M, et al. Studies on tissue and cellular distribution of indoleamine 2,3-dioxygenase 2: the absence of ido1 upregulates ido2 expression in the epididymis. J Histochem Cytochem. (2012) 60:854–60. doi: 10.1369/0022155412458926
17. Munn DH, Zhou M, Attwood JT, Bondarev I, Conway SJ, Marshall B, et al. Prevention of allogeneic fetal rejection by tryptophan catabolism. Science. (1998) 281:1191–3. doi: 10.1126/science.281.5380.1191
18. Uyttenhove C, Pilotte L, Theate I, Stroobant V, Colau D, Parmentier N, et al. Evidence for a tumoral immune resistance mechanism based on tryptophan degradation by indoleamine 2,3-dioxygenase. Nat Med. (2003) 9:1269–74. doi: 10.1038/nm934
19. Munn DH, Mellor AL. Indoleamine 2,3-dioxygenase and tumor-induced tolerance. J Clin Invest. (2007) 117:1147–54. doi: 10.1172/JCI31178
20. Opitz CA, Litzenburger UM, Sahm F, Ott M, Tritschler I, Trump S, et al. An endogenous tumour-promoting ligand of the human aryl hydrocarbon receptor. Nature. (2011) 478:197–203. doi: 10.1038/nature10491
21. Platten M, Wick W, Van den Eynde BJ. Tryptophan catabolism in cancer: beyond ido and tryptophan depletion. Cancer Res. (2012) 72:5435–40. doi: 10.1158/0008-5472.CAN-12-0569
22. Zamanakou M, Germenis AE, Karanikas V. Tumor immune escape mediated by indoleamine 2,3-dioxygenase. Immunol Lett. (2007) 111:69–75. doi: 10.1016/j.imlet.2007.06.001
23. Curti A, Pandolfi S, Valzasina B, Aluigi M, Isidori A, Ferri E, et al. Modulation of tryptophan catabolism by human leukemic cells results in the conversion of Cd25(-) into Cd25(+) T Regulatory cells. Blood. (2007) 109:2871–7. doi: 10.1182/blood-2006-07-036863
24. Sharma MD, Hou DY, Liu YJ, Koni PA, Metz R, Chandler P, et al. Indoleamine 2,3-dioxygenase controls conversion of foxp3(+) tregs to Th17-like cells in tumor-draining lymph nodes. Blood. (2009) 113:6102–11. doi: 10.1182/blood-2008-12-195354
25. Munn DH, Mellor AL. IDO in the tumor microenvironment: inflammation, counter-regulation, and tolerance. Trends Immunol. (2016) 37:193–207. doi: 10.1016/j.it.2016.01.002
26. Mezrich JD, Fechner JH, Zhang XJ, Johnson BP, Burlingham WJ, Bradfield CA. An interaction between kynurenine and the aryl hydrocarbon receptor can generate regulatory T Cells. J Immunol. (2010) 185:3190–8. doi: 10.4049/jimmunol.0903670
27. Campesato LF, Budhu S, Tchaicha J, Weng CH, Gigoux M, Cohen IJ, et al. Blockade of the Ahr restricts a treg-macrophage suppressive axis induced by l-kynurenine. Nat Commun. (2020) 11:4011. doi: 10.1038/s41467-020-17750-z
28. Stockinger B, Di Meglio P, Gialitakis M, Duarte JH. The aryl hydrocarbon receptor: multitasking in the immune system. Ann Rev Immunol. (2014) 32:403–32. doi: 10.1146/annurev-immunol-032713-120245
29. Fallarini S, Magliulo L, Paoletti T, de Lalla C, Lombardi G. Expression of functional gpr35 in human inkt cells. Biochem Biophys Res Commun. (2010) 398:420–5. doi: 10.1016/j.bbrc.2010.06.091
30. DiNatale BC, Murray IA, Schroeder JC, Flaveny CA, Lahoti TS, Laurenzana EM, et al. Kynurenic acid is a potent endogenous aryl hydrocarbon receptor ligand that synergistically induces interleukin-6 in the presence of inflammatory signaling. Toxicol Sci. (2010) 115:89–97. doi: 10.1093/toxsci/kfq024
31. Korn T, Bettelli E, Gao W, Awasthi A, Jager A, Strom TB, et al. Il-21 Initiates an alternative pathway to induce proinflammatory T(H)17 cells. Nature. (2007) 448:484–U9. doi: 10.1038/nature05970
32. Veldhoen M, Hocking RJ, Atkins CJ, Locksley RM, Stockinger B. Tgf Beta in the context of an inflammatory cytokine milieu supports de novo differentiation of Il-17-producing T cells. Immunity. (2006) 24:179–89. doi: 10.1016/j.immuni.2006.01.001
33. Veldhoen M, Hirota K, Westendorf AM, Buer J, Dumoutier L, Renauld JC, et al. The Aryl hydrocarbon receptor links T(H)17-cell-mediated autoimmunity to environmental toxins. Nature. (2008) 453:106. doi: 10.1038/nature06881
34. Quintana FJ, Basso AS, Iglesias AH, Korn T, Farez MF, Bettelli E, et al. Control of T-Reg and Th17 cell differentiation by the aryl hydrocarbon receptor. Nature. (2008) 453:65. doi: 10.1038/nature06880
35. Yang XO, Nurieva R, Martinez GJ, Kang HS, Chung Y, Pappu BP, et al. Molecular antagonism and plasticity of regulatory and inflammatory T cell programs. Immunity. (2008) 29:44–56. doi: 10.1016/j.immuni.2008.05.007
36. Yamada T, Horimoto H, Kameyama T, Hayakawa S, Yamato H, Dazai M, et al. Constitutive aryl hydrocarbon receptor signaling constrains type I interferon-mediated antiviral innate defense. Nat Immunol. (2016) 17:687. doi: 10.1038/ni.3422
37. Hoshi M, Saito K, Hara A, Taguchi A, Ohtaki H, Tanaka R, et al. The absence of ido upregulates type I Ifn production, resulting in suppression of viral replication in the retrovirus-infected mouse. J Immunol. (2010) 185:3305–12. doi: 10.4049/jimmunol.0901150
38. Anderson G, Carbone A, Mazzoccoli G. Tryptophan metabolites and aryl hydrocarbon receptor in severe acute respiratory syndrome, coronavirus-2 (Sars-Cov-2) pathophysiology. Int J Mol Sci. (2021) 22:1597. doi: 10.3390/ijms22041597
39. Liu YY, Lv JD, Liu JN, Li M, Xie J, Lv Q, et al. Mucus production stimulated by Ifn-Ahr signaling triggers hypoxia of Covid-19. Cell Res. (2020) 30:1078–87. doi: 10.1038/s41422-020-00435-z
40. Sorgdrager FJH, Naude PJW, Kema IP, Nollen EA, De Deyn PP. Tryptophan metabolism in inflammaging: from biomarker to therapeutic target. Front Immunol. (2019) 10:2565. doi: 10.3389/fimmu.2019.02565
41. Lamas B, Hernandez-Galan L, Galipeau HJ, Constante M, Clarizio A, Jury J, et al. Aryl hydrocarbon receptor ligand production by the gut microbiota is decreased in celiac disease leading to intestinal inflammation. Sci Transl Med. (2020) 12:eaba0624. doi: 10.1126/scitranslmed.aba0624
42. Agus A, Planchais J, Sokol H. Gut microbiota regulation of tryptophan metabolism in health and disease. Cell Host Microbe. (2018) 23:716–24. doi: 10.1016/j.chom.2018.05.003
43. Alexeev EE, Lanis JM, Kao DJ, Campbell EL, Kelly CJ, Battista KD, et al. Microbiota-derived indole metabolites promote human and murine intestinal homeostasis through regulation of interleukin-10 receptor. Am J Pathol. (2018) 188:1183–94. doi: 10.1016/j.ajpath.2018.01.011
44. Hubbard TD, Murray IA, Perdew GH. Indole and tryptophan metabolism: endogenous and dietary routes to Ah receptor activation. Drug Metabol Dispos. (2015) 43:1522–35. doi: 10.1124/dmd.115.064246
45. Lamas B, Richard ML, Leducq V, Pham HP, Michel ML, Da Costa G, et al. Card9 Impacts colitis by altering gut microbiota metabolism of tryptophan into Aryl hydrocarbon receptor ligands. Nat Med. (2016) 22:598. doi: 10.1038/nm.4102
46. Luisa Cervantes B, Chai J, Tianero MD, Di Luccia B, Ahern P, Merriman J, et al. Lactobacillus reuteri induces gut intraepithelial CD4+ CD8αα+ T Cells. J Immunol. (2017) 25:806–10. doi: 10.1126/science.aah5825
47. Takamura T, Harama D, Fukumoto S, Nakamura Y, Shimokawa N, Ishimaru K, et al. Lactobacillus bulgaricus Oll1181 activates the aryl hydrocarbon receptor pathway and inhibits colitis. Immunol Cell Biol. (2011) 89:817–22. doi: 10.1038/icb.2010.165
48. Sujino T, London M, van Konijnenburg DPH, Rendon T, Buch T, Silva HM, et al. Tissue adaptation of regulatory and intraepithelial Cd4(+) T Cells controls gut inflammation. Science. (2016) 352:1581–6. doi: 10.1126/science.aaf3892
49. Qiu J, Heller JJ, Guo XH, Chen ZME, Fish K, Fu YX, et al. The Aryl hydrocarbon receptor regulates gut immunity through modulation of innate lymphoid cells. Immunity. (2012) 36:92–104. doi: 10.1016/j.immuni.2011.11.011
50. Zelante T, Iannitti RG, Cunha C, De Luca A, Giovannini G, Pieraccini G, et al. Tryptophan catabolites from microbiota engage Aryl hydrocarbon receptor and balance mucosal reactivity via interleukin-22. Immunity. (2013) 39:372–85. doi: 10.1016/j.immuni.2013.08.003
51. Hamming I, Timens W, Bulthuis MLC, Lely AT, Navis GJ, van Goor H. Tissue distribution of ace2 protein, the functional receptor for sars coronavirus. a first step in understanding sars pathogenesis. J Pathol. (2004) 203:631–7. doi: 10.1002/path.1570
52. Hikmet F, Mear L, Edvinsson A, Micke P, Uhlen M, Lindskog C. The protein expression profile of Ace2 in human tissues. Mol Syst Biol. (2020) 16:e9610. doi: 10.15252/msb.20209610
53. Wang YL, Wang Y, Luo WS, Huang LZ, Xiao J, Li F, et al. A Comprehensive investigation of the Mrna and protein level of Ace2, the putative receptor of sars-cov-2, in human tissues and blood cells. Int J Med Sci. (2020) 17:1522–31. doi: 10.7150/ijms.46695
54. Li MY, Li L, Zhang Y, Wang XS. Expression of the Sars-Cov-2 cell receptor gene Ace2 in a wide variety of human tissues. Infect Dis Poverty. (2020) 9:45. doi: 10.1186/s40249-020-00662-x
55. Prestes TRR, Rocha NP, Miranda AS, Teixeira AL, Silva A. The anti-inflammatory potential of Ace2/angiotensin-(1-7)/mas receptor axis: evidence from basic and clinical research. Curr Drug Targets. (2017) 18:1301–13. doi: 10.2174/1389450117666160727142401
56. Santos RAS, Sampaio WO, Alzamora AC, Motta-Santos D, Alenina N, Bader M, et al. The Ace2/angiotensin-(1-7)/mas axis of the renin-angiotensin system: focus on angiotensin-(1-7). Physiol Rev. (2018) 98:505–53. doi: 10.1152/physrev.00023.2016
57. Hashimoto T, Perlot T, Rehman A, Trichereau J, Ishiguro H, Paolino M, et al. Ace2 Links amino acid malnutrition to microbial ecology and intestinal inflammation. Nature. (2012) 487:477–81. doi: 10.1038/nature11228
58. Perlot T, Penninger JM. Ace2-from the renin-angiotensin system to gut microbiota and malnutrition. Microbes Infect. (2013) 15:866–73. doi: 10.1016/j.micinf.2013.08.003
59. Klempin F, Mosienko V, Matthes S, Villela DC, Todiras M, Penninger JM, et al. Depletion of angiotensin-converting enzyme 2 reduces brain serotonin and impairs the running-induced neurogenic response. Cell Mol Life Sci. (2018) 75:3625–34. doi: 10.1007/s00018-018-2815-y
60. Lv JD, Yu P, Wang ZF, Deng W, Bao LL, Liu JN, et al. Ace2 Expression is regulated by Ahr in Sars-Cov-2-infected macaques. Cell Mol Immunol. (2021) 18:1308–10. doi: 10.1038/s41423-021-00672-1
61. Tanimoto K, Hirota K, Fukazawa T, Matsuo Y, Nomura T, Tanuza N, et al. Inhibiting Sars-Cov-2 infection in vitro by suppressing its receptor, angiotensin-converting enzyme 2, via aryl-hydrocarbon receptor signal. Sci Rep. (2021) 11:16629. doi: 10.1038/s41598-021-96109-w
62. Imai Y, Kuba K, Rao S, Huan Y, Guo F, Guan B, et al. Angiotensin-converting enzyme 2 protects from severe acute lung failure. Nature. (2005) 436:112–6. doi: 10.1038/nature03712
63. Lambert DW, Yarski M, Warner FJ, Thornhill P, Parkin ET, Smith AI, et al. Tumor necrosis factor-alpha convertase (Adam17) mediates regulated ectodomain shedding of the severe-acute respiratory syndrome-coronavirus (Sars-Cov) receptor, angiotensin-converting enzyme-2 (Ace2). J Biol Chem. (2005) 280:30113–9. doi: 10.1074/jbc.M505111200
64. Nagy B, Fejes Z, Szentkereszty Z, Suto R, Varkonyi I, Ajzner E, et al. A dramatic rise in serum Ace2 activity in a critically ill Covid-19 patient. Int J Infect Dis. (2021) 103:412–4. doi: 10.1016/j.ijid.2020.11.184
65. Kragstrup TW, Singh HS, Grundberg I, Nielsen ALL, Rivellese F, Mehta A, et al. Plasma Ace2 predicts outcome of Covid-19 in hospitalized patients. PLoS ONE. (2021) 16:e0252799. doi: 10.1371/journal.pone.0252799
66. Fagyas M, Fejes Z, Süto R, Nagy Z, Székely B, Pócsi M, et al. Circulating Ace2 activity predicts mortality and disease severity in hospitalized Covid-19 patients. Int J Infect Dis. (2022) 115:8–16. doi: 10.1016/j.ijid.2021.11.028
67. van der Goot AT, Nollen EAA. Tryptophan metabolism: entering the field of aging and age-related pathologies. Trends Mol Med. (2013) 19:336–44. doi: 10.1016/j.molmed.2013.02.007
68. Kameda M, Teruya T, Yanagida M, Kondoh H. Frailty markers comprise blood metabolites involved in antioxidation, cognition, and mobility. Proc Natl Acad Sci U S A. (2020) 117:9483–9. doi: 10.1073/pnas.1920795117
69. Rattray NJW, Trivedi DK, Xu Y, Chandola T, Johnson CH, Marshall AD, et al. Metabolic dysregulation in vitamin E and carnitine shuttle energy mechanisms associate with human frailty. Nat Commun. (2019) 10:5027. doi: 10.1038/s41467-019-12716-2
70. Yaku K, Okabe K, Nakagawa T. Nad metabolism: implications in aging and longevity. Ageing Res Rev. (2018) 47:1–17. doi: 10.1016/j.arr.2018.05.006
71. Katsyuba E, Mottis A, Zietak M, De Franco F, van der Velpen V, Gariani K, et al. De novo Nad(+) synthesis enhances mitochondrial function and improves health. Nature. (2018) 563:354. doi: 10.1038/s41586-018-0645-6
72. Takeshita H, Yamamoto K, Nozato S, Takeda M, Fukada SI, Inagaki T, et al. Angiotensin-converting enzyme 2 deficiency accelerates and angiotensin 1-7 restores age-related muscle weakness in mice. J Cachexia Sarcopenia Muscle. (2018) 9:975-86. doi: 10.1002/jcsm.12334
73. Nozato S, Yamamoto K, Takeshita H, Nozato Y, Imaizumi Y, Fujimoto T, et al. Angiotensin 1-7 alleviates aging-associated muscle weakness and bone loss, but is not associated with accelerated aging in Ace2-knockout mice. Clin Sci. (2019) 133:2005–18. doi: 10.1042/CS20190573
74. Takeshita H, Yamamoto K, Mogi M, Nozato S, Horiuchi M, Rakugi H. Different effects of the deletion of angiotensin converting enzyme 2 and chronic activation of the renin-angiotensin system on muscle weakness in middle-aged mice. Hypertens Res. (2019) 43:1-9. doi: 10.1038/s41440-019-0375-7
75. Biolo G, Tipton KD, Klein S, Wolfe RR. An abundant supply of amino acids enhances the metabolic effect of exercise on muscle protein. Am J Physiol. (1997) 273:E122–9.
76. Marron MM, Harris TB, Boudreau RM, Clish CB, Moore SC, Murphy RA, et al. Metabolites associated with vigor to frailty among community-dwelling older black men. Metabolites. (2019) 9:83. doi: 10.3390/metabo9050083
77. Marcos-Pérez D, Sánchez-Flores M, Maseda A, Lorenzo-López L, Millán-Calenti JC, Strasser B, et al. Frailty status in older adults is related to alterations in indoleamine 2,3-dioxygenase 1 and guanosine triphosphate cyclohydrolase i enzymatic pathways. J Am Med Dir Assoc. (2017) 18:1049–57. doi: 10.1016/j.jamda.2017.06.021
78. Fazelzadeh P, Hangelbroek RW, Tieland M, de Groot LC, Verdijk LB, van Loon LJ, et al. The muscle metabolome differs between healthy and frail older adults. J Proteome Res. (2016) 15:499–509. doi: 10.1021/acs.jproteome.5b00840
79. Drummond MJ, Fry CS, Glynn EL, Timmerman KL, Dickinson JM, Walker DK, et al. Skeletal muscle amino acid transporter expression is increased in young and older adults following resistance exercise. J Appl Physiol (1985). (2011) 111:135–42. doi: 10.1152/japplphysiol.01408.2010
80. Martin KS, Azzolini M, Lira Ruas J. The kynurenine connection: how exercise shifts muscle tryptophan metabolism and affects energy homeostasis, the immune system, and the brain. Am J Physiol Cell Physiol. (2020) 318:C818–30. doi: 10.1152/ajpcell.00580.2019
81. Agudelo LZ, Femenia T, Orhan F, Porsmyr-Palmertz M, Goiny M, Martinez-Redondo V, et al. Skeletal muscle pgc-1 alpha 1 modulates kynurenine metabolism and mediates resilience to stress-induced depression. Cell. (2014) 159:33–45. doi: 10.1016/j.cell.2014.07.051
82. Schlittler M, Goiny M, Agudelo LZ, Venckunas T, Brazaitis M, Skurvydas A, et al. Endurance exercise increases skeletal muscle kynurenine aminotransferases and plasma kynurenic acid in humans. Am J Physiol Cell Physiol. (2016) 310:C836–40. doi: 10.1152/ajpcell.00053.2016
83. Lewis GD, Farrell L, Wood MJ, Martinovic M, Arany Z, Rowe GC, et al. Metabolic signatures of exercise in human plasma. Sci Transl Med. (2010) 2:33ra7. doi: 10.1126/scitranslmed.3001006
84. Allison DJ, Nederveen JP, Snijders T, Bell KE, Kumbhare D, Phillips SM, et al. Exercise training impacts skeletal muscle gene expression related to the kynurenine pathway. Am J Physiol Cell Physiol. (2019) 316:C444–8. doi: 10.1152/ajpcell.00448.2018
85. Kaiser H, Yu K, Pandya C, Mendhe B, Isales CM, McGee-Lawrence ME, et al. Kynurenine, a tryptophan metabolite that increases with age, induces muscle atrophy and lipid peroxidation. Oxid Med Cell Longev. (2019) 2019:9894238. doi: 10.1155/2019/9894238
86. Ninomiya S, Nakamura N, Nakamura H, Mizutani T, Kaneda Y, Yamaguchi K, et al. Low levels of serum tryptophan underlie skeletal muscle atrophy. Nutrients. (2020) 12:978. doi: 10.3390/nu12040978
87. Ali AM, Kunugi H. Skeletal muscle damage in covid-19: a call for action. Medicina. (2021) 57:372. doi: 10.3390/medicina57040372
88. Jenkins TA, Nguyen JCD, Polglaze KE, Bertrand PP. Influence of tryptophan and serotonin on mood and cognition with a possible role of the gut-brain axis. Nutrients. (2016) 8:56. doi: 10.3390/nu8010056
89. Segall PE, Timiras PS, Walton JR. Low tryptophan diets delay reproductive aging. Mech Ageing Dev. (1983) 23:245–52. doi: 10.1016/0047-6374(83)90024-6
90. Ooka H, Segall PE, Timiras PS. Histology and survival in age-delayed low-tryptophan-fed rats. Mech Ageing Dev. (1988) 43:79–98. doi: 10.1016/0047-6374(88)90099-1
91. Segall PE, Timiras PS. Patho-Physiologic findings after chronic tryptophan deficiency in rats - model for delayed growth and aging. Mech Ageing Dev. (1976) 5:109–24. doi: 10.1016/0047-6374(76)90012-9
92. Brown-Borg HM, Buffenstein R. Cutting back on the essentials: can manipulating intake of specific amino acids modulate health and lifespan? Ageing Res Rev. (2017) 39:87–95. doi: 10.1016/j.arr.2016.08.007
93. Gao J, Xu K, Liu HN, Liu G, Bai MM, Peng C, et al. Impact of the gut microbiota on intestinal immunity mediated by tryptophan metabolism. Front Cell Infect Microbiol. (2018) 8:13. doi: 10.3389/fcimb.2018.00013
94. Strasser B, Geiger D, Schauer M, Gostner JM, Gatterer H, Burtscher M, et al. Probiotic supplements beneficially affect tryptophan-kynurenine metabolism and reduce the incidence of upper respiratory tract infections in trained athletes: a randomized, double-blinded, placebo-controlled trial. Nutrients. (2016) 8:752. doi: 10.3390/nu8110752
Keywords: angiotensin converting enzyme 2 (ACE2), tryptophan, skeletal muscle, COVID-19, SARS-CoV2
Citation: Takeshita H and Yamamoto K (2022) Tryptophan Metabolism and COVID-19-Induced Skeletal Muscle Damage: Is ACE2 a Key Regulator? Front. Nutr. 9:868845. doi: 10.3389/fnut.2022.868845
Received: 03 February 2022; Accepted: 07 March 2022;
Published: 08 April 2022.
Edited by:
Caterina Conte, Università telematica San Raffaele, ItalyReviewed by:
Teresa Femenia, Institute of Neurosciences (CSIC), SpainCopyright © 2022 Takeshita and Yamamoto. This is an open-access article distributed under the terms of the Creative Commons Attribution License (CC BY). The use, distribution or reproduction in other forums is permitted, provided the original author(s) and the copyright owner(s) are credited and that the original publication in this journal is cited, in accordance with accepted academic practice. No use, distribution or reproduction is permitted which does not comply with these terms.
*Correspondence: Hikari Takeshita, takeshita@geriat.med.osaka-u.ac.jp; Koichi Yamamoto, kyamamoto@geriat.med.osaka-u.ac.jp
†These authors have contributed equally to this work