- Department of Medicine, Section of Internal Medicine C, University of Verona, Verona, Italy
Platelet-derived large extracellular vesicles (often referred to as microparticles in the field of cardiovascular disease) have been identified as effector in the atherothrombotic process, therefore representing a target of pharmacological intervention of potential interest. Despite that, limited evidence is so far available concerning the effects of antiplatelet agents on the release of platelet-derived extracellular vesicles. In the present narrative review, the mechanisms leading to vesiculation in platelets and the pathophysiological processes implicated will be discussed. This will be followed by a summary of the present evidence concerning the effects of antiplatelet agents under experimental conditions and in clinical settings.
Biochemical Characterization of Platelet-Derived Extracellular Vesicles
The first evidence of extracellular vesicles (EVs) derived from platelet dates back to 1967, thanks to Wolf (1967), who named them “platelet dusts” even if considered factors promoting clot formation (Nieuwland et al., 2012).
EVs from different cellular origin circulate or are detectable in biological fluids, endowed with different biological functions that are being investigated for their potential pharmacological and clinical relevance. In fact, correlation has been observed between the number of circulating EVs and the clinical expression of diseases such as diabetes mellitus, chronic kidney disease, preeclampsia, and severe hvypertension (Burger et al., 2013; Campello et al., 2015). Thus, platelet-derived extracellular vesicles (PEVs) may represent both useful biomarkers of platelet-mediated pathophysiological processes and mediators of intercellular communication.
Platelets release different types of extracellular products in response to activation and apoptosis. Generally, they are 30- to 800-nm-large vesicles, whose size and amount make them difficult to purify and characterize properly.
The International Society for Extracellular Vesicles (ISEV) proposed the MISEV2018 guidelines, which include suggestions about nomenclature, collection, separation, characterization, and concentrations of EVs (Théry et al., 2018).
ISEV defines EVs as particles released from the cell that are delimited by a lipid bilayer without a functional nucleus. EVs can be classified by physical characteristics such as size (<100-nm small EVs, 100- to 200-nm medium/large EVs, and >200-nm large EVs) or density (low, middle, high, with each range defined) (Théry et al., 2018). ISEV proposes to classify EVs also according to their biochemical characteristics (i.e., CD63+/CD81+- EVs, annexin A5–stained EVs, etc.) or by descriptions of conditions or cell of origin [podocyte EVs, hypoxic EVs, large oncosomes, apoptotic bodies (ABs)]. Without further classification, the generic term EVs is considered as appropriate (Théry et al., 2018).
EVs, based on their size and supposed origin, can be further grouped into three specific classes: (i) ABs, having an average diameter of 800 to 5,000 nm, which are released by programmed cell death; (ii) large EVs, with a diameter of approximately 50–1,000 nm, deriving from budding of the cell membrane; and (iii) small EVs, also named exosomes, the smallest vesicles of the three classes with a diameter of 40 to 100 nm, released through endocytic process (Kalra et al., 2012; Simpson and Mathivanan, 2012).
Large and small EVs display some specific features that can help to differentiate one from the other. Small EV generation involves tetraspanins (CD9, CD63), tumor susceptibility gene 101 (TSG101), and programmed cell death 6–interacting protein (PDCD6IP or ALIX) (Théry et al., 2018). Furthermore, small and large EVs can be distinguished for their RNA content in terms of RNA types and RNA amount (Tao et al., 2017). Since both large and small EVs carry and deliver cellular signals, it is plausible to suggest a potential role in cell–cell signaling (Dovizio et al., 2015). An important difference between the two families of vesicles is their different biological activity such as the procoagulant activity of large EVs originated from platelets and the involvement of vesiculation in a variety of physiological and pathological processes including angiogenesis, cell proliferation, apoptosis, and inflammation (Heijnen et al., 1999; Tao et al., 2017).
Focus of the present review will be on large PEVs, often named microparticles in the field of cardiovascular medicine, representing 70% to 90% of circulating large EVs in healthy subjects (Horstman and Ahn, 1999;Diamant et al., 2004). Under pathological conditions including cancer, sepsis, diabetes, and acute coronary syndromes, increased number of circulation PEVs has been detected (Shantsila et al., 2010; Varon and Shai, 2015). Deficiency in PEV release is associated with a bleeding disorder characterized by prolonged bleeding time (Castaman et al., 1997). Evidence exists that the number of released PEVs is reduced after pharmacological treatment in cardiovascular disorders (Morel et al., 2006). This has been observed, for instance, in hyperlipidemic patients with type 2 diabetes after treatment with statins and eicosapentaenoic acid (Nomura et al., 2009; Nomura et al., 2018). The effects of antiplatelet agents have so far been addressed only in a limited number of studies, not always as the main endpoint, although this may represent a rational pharmacological intervention aimed at reducing the release of PEVs. For instance, reduced number of circulating EVs from different cellular origin has been observed in patients with acute coronary syndrome treated with aspirin and P2Y12 receptor antagonists (Behan et al., 2005; Bulut et al., 2011). However, data are so far controversial, and this is the main topic of the present review that will be discussed in detail further on.
PEVs carry on their surface most of the proteins and receptors that are expressed on platelet plasma membrane. Large PEVs carry the prothrombinase complex and the alpha granule-derived factor V (Sims et al., 1989). Furthermore, large PEVs express receptors including the fibrinogen receptor αIIb/β3, the von Willebrand factor receptor GPIb, and P-selectin (Mustard et al., 2002). Along with cell-surface proteins, cytosolic content of PEVs includes RNA, miRNA, and perchance DNA that can be transferred to target cells (Burger et al., 2013).
The important role of PEVs in intercellular communication, hemostasis, angiogenesis, and several other physiological and pathological conditions, due to their procoagulant surface, the expression of several receptors, and the cytosolic content, is receiving increasing scientific interest as discussed in several reports (Morel et al., 2008; Vajen et al., 2015; Todorova et al., 2017).
Isolation, Detection, and Characterization of Platelet-Derived Extracellular Vesicles: Methodological Issues
Different methods are available for PEV isolation, characterization, and quantification, but when multiple parameters have to be considered, the use of different techniques in combination is required (Kailashiya, 2018). In fact, none of the techniques so far used in clinical studies and in vitro models allow simultaneous and accurate information on biochemical properties and quantity of PEVs; exhaustive information could only be obtained by combining methodological approaches (Momen-Heravi et al., 2013; Burnouf et al., 2014; van der Pol and Harrison, 2017).
In addition, many preanalytical and postanalytical factors affect PEV measurement and characterization, such as blood collection, handling, and storage (Jy et al., 2004). It is therefore necessary to use any possible precaution and to follow updated recommendations to minimize errors. All these factors have to be taken into account as source of potential bias and limitation in the reliability of experimental studies and clinical trials.
Isolation and Concentration of Platelet-Derived Extracellular Vesicles
Collection and manipulation of platelets from blood samples require very rigorous handling and processing in order to avoid artifacts and to favor interlaboratory standardizations, first of all using a large needle size (size 21-gauge or larger), discarding the first milliliters of collected blood to prevent platelet activation or desensitization.
The choice of the anticoagulant is important for PEV characterization and quantification, being conditioned by the downstream analysis. The most used anticoagulant is sodium citrate (Robert et al., 2009; Mobarrez et al., 2010; Iversen et al., 2013; Kailashiya, 2018; Zhang et al., 2018; Mitrugno et al., 2019), which minimizes in vitro platelet activation and consequent PEV release that is observed with ethylenediaminetetraacetic acid (EDTA) or heparin (Beutler et al., 1990; Gomes et al., 2018). However, EDTA (Chandler, 2016) is suitable when interest is in RNA analysis (Ostenfeld et al., 2016; van Eijndhoven et al., 2016), although affecting EV quantification (Shah et al., 2008; Nelles and Chandler, 2014). Citrate-dextrose solution (ACD) plus EDTA is an alternative anticoagulant for the quantification of circulating PEVs in plasma samples (Shirafuji et al., 2008; Nomura et al., 2009; Shirafuji et al., 2009). ACD alone is used when EVs derived from washed platelets are studied in vitro (Castaman et al., 1997; Conde et al., 2005; Pontiggia et al., 2006; Suades et al., 2012; Hsu et al., 2013; Aatonen et al., 2014; György et al., 2014; Vajen et al., 2015; Anene et al., 2018). CTAD (sodium citrate, citric acid, theophylline, adenosine, and dipyridamole) can be useful to prevent in vitro platelet activation but can alter intraplatelet signaling, since it increases cytosolic AMP concentration (Mody et al., 1999; Kim et al., 2002). To avoid coagulation of blood or plasma samples, PPACK is used as inhibitor of thrombin-mediated platelet activation, not affecting extracellular calcium concentration (Gemmell et al., 1993; Chandler et al., 2011; Chandler, 2013; Giacomazzi et al., 2016).
Concerning the techniques used in PEV separation and concentration, the MISEV 2018 guidelines define these procedures as follows: separation of EVs (purification or isolation) is generally referred to separation from non-EV component or separation of a specific population of EVs from the other ones. Concentration is the procedure that allows to increase the number of EVs per volume unit with or without separation (Théry et al., 2018).
The most used methods to isolate/concentrate PEVs are based on differential centrifugation (where PEVs can be obtained from supernatant or pellet) or density gradient centrifugation. Isolation depends on the size and mass density or mass density only, but does not separate PEVs from non-EV components such as lipoprotein particles (i.e., chylomicrons), cellular debris, protein aggregates, and very large proteins such as von Willebrand factor (Coumans et al., 2017).
Size exclusion chromatography enables size-based separation on a single column, thus separating EVs from non-EV soluble components (Xu et al., 2016). The choice of matrix determines the size cutoff (e.g., Sepharose 2B has a pore of about 60 nm). Size exclusion chromatography preserves structure and functionality of EVs better than ultracentrifugation (Nordin et al., 2015; Gámez-Valero et al., 2016; Hong et al., 2016). Size exclusion chromatography can be used in association with other techniques such as ultrafiltration in which EVs are retained (Grasso et al., 2015; Nordin et al., 2015).
Immunocapture techniques with monoclonal antibodies and magnetic beads or surfaces are used to isolate subpopulations of EVs on the basis of their immunophenotype (Osumi et al., 2001; Shih et al., 2016; Obeid et al., 2017).
Detection and Identification of Platelet-Derived Extracellular Vesicles
PEV detection methods can be classified into quantitative (count of EVs) and qualitative (size distribution, morphology, phenotyping, content of proteins o nucleic acid, and functional analysis).
Currently, a single method does not allow phenotyping, sizing, and enumerating PEVs. Standardization of the protocols is therefore recommended to reduce interlaboratory variability (Lacroix et al., 2010a; Lacroix et al., 2010b).
Referring to PEV count, conventional flow cytometry is the mostly used technique. Fluorescein isothiocyanate–phalloidin staining allows to distinguish PEVs from cell fragments in the samples, thus minimizing errors (Lacroix et al., 2010a; Mobarrez et al., 2010; Unsworth et al., 2017). Fluorescent-labeled monoclonal antibodies against CD41 (αIIb), CD62P (P-selectin), CD61 (β3), and the active fibrinogen receptor αIIb/β3 (using PAC-1 monoclonal antibody) specifically detect PEVs, besides that most PEVs are also positive for annexin V or lactadherin (Christersson et al., 2010; Connor et al., 2010; Mobarrez et al., 2010; Ayers et al., 2011; Chandler, 2013; Giacomazzi et al., 2016). Annexin V and lactadherin are able to bind phosphatidylserine in a stereospecific manner that is calcium dependent for annexin V and calcium independent for lactadherin (Shi et al., 2003). Unlike PEVs, megakaryocyte-derived large EVs do not express CD62P and LAMP-1 (Flaumenhaft et al., 2009; Italiano et al., 2010; Boilard et al., 2015). Beads are used for the standardization of EV quantification across different cytofluorometric platforms (Robert et al., 2009; Cointe et al., 2016). Standardization is in fact fundamental when the clinical relevance of PEV count is evaluated, allowing multicenter studies.
For ex vivo and in vitro studies, electron microscopy gives the advantage of detecting very small size particles and is considered the gold standard as imaging technique for PEVs (Brisson et al., 2017). The resolution is in the nanometer scale and allows evaluating structure and morphology of EVs.
Cryo-electron microscopy is useful to study size distribution, morphology, and structure of EVs (Tatischeff et al., 2012).
Atomic force microscopy can be used to count and characterize dimension and distribution of EVs in the nanometer size range (10- to 475-nm range), below the lower limit of detection of conventional flow cytometry. It has been shown that the number of PEVs detected by atomic force microscopy is approximately 1,000‐fold higher than the number detected by flow cytometry (Yuana et al., 2010). Atomic force microscopy can be used to study EVs immobilized on a functionalized surface avoiding interference from abundant proteins (fibrinogen, albumin, immunoglobulins, etc.) and can be coupled to other techniques such as surface plasmon resonance to detect different subpopulations of EVs over a wide concentration range (Gajos et al., 2017; Obeid et al., 2017).
New methodological approaches to the analysis of EVs should allow a more effective characterization of PEVs as summarized in Table 1.
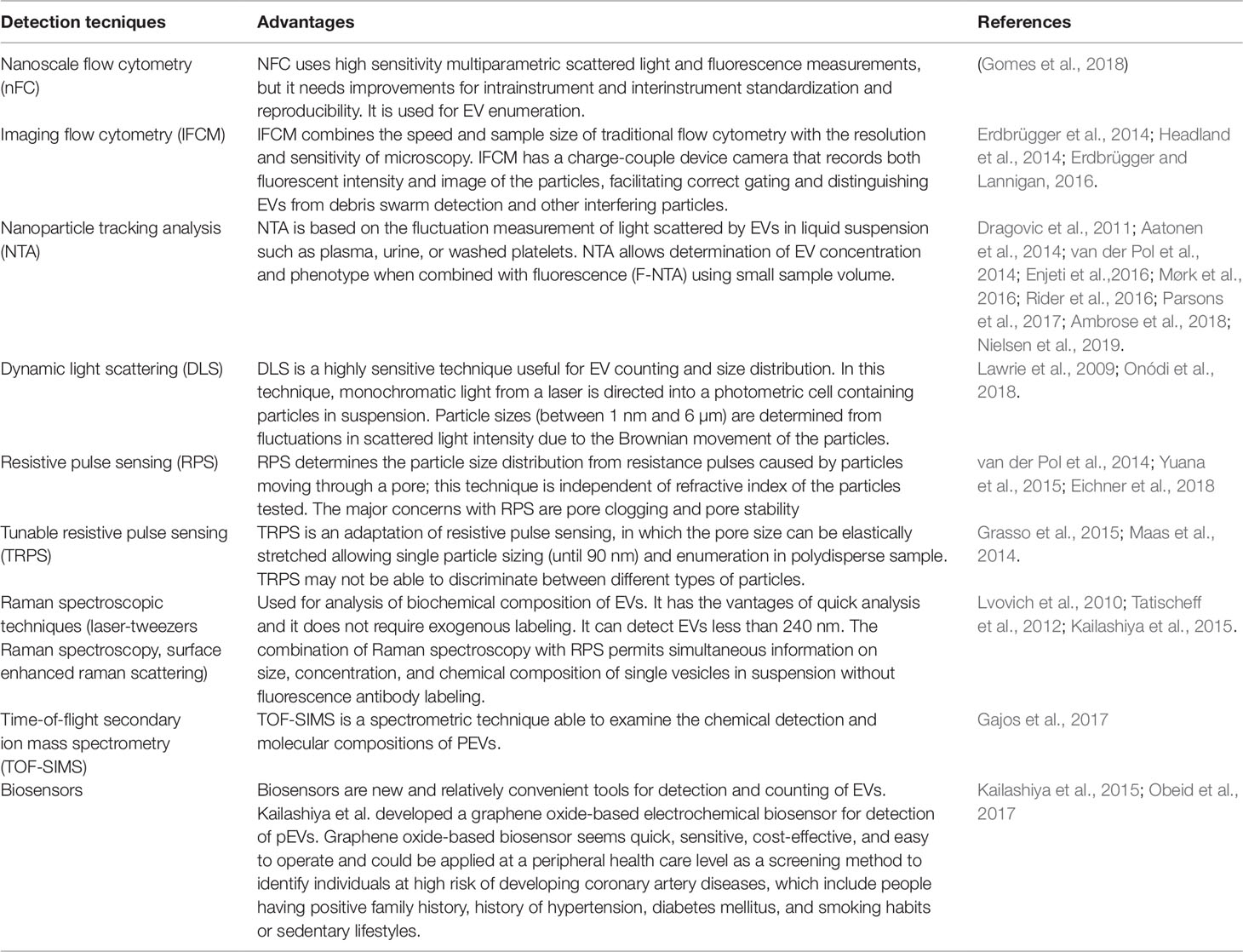
Table 1 Innovative techniques potentially useful for the detection and quantification of platelet-derived extracellular vesicles (PEVs).
Platelet Activation and the Release of Large Extracellular Vesicles
Platelet activation in response to soluble agonists (such as thrombin, ADP, and collagen), activators of second messengers (like calcium ionophores and phorbol esters), high shear stress, contact with exogenous surfaces, complement, or low temperatures gives rise to granule secretion and large EV release (Heijnen et al., 1999; Nieuwland et al., 2012). Large PEV formation is an event in which the inner cytoskeleton is disrupted, the symmetry of membrane phospholipids is altered, and the outward plasma membrane undergoes blebbing fragmentation (Morel et al., 2011). Actin filaments, main component of the cytoskeletal, play a major role in PEV formation. The α-actin fibers are cleaved by the Ca++dependent protease, calpain, and its inhibition reduces blebbing of PEVs (Yano et al., 1993). Another essential event involved in the release of large PEVs is the externalization of phosphatidylserine, an amino-phospholipid mainly present on the inner leaflet of plasma membrane (Bevers et al., 1999). Flippases promote the translocation of phosphatidylserine and phosphatidylethanolamine toward the inner side of the plasma membrane of the platelet against their own electrochemical gradient through an ATP-dependent mechanism. Floppases, ABC transporter family members (ATP-binding cassette transporter), allow the transport of phosphatidylserine to the outer membrane. Lastly, in an ATP-independent manner, scramblase enzymes help the translocation of phospholipids, inside the two membrane leaflets (Suzuki et al., 2010). Alterations in the membrane phospholipids symmetry and, consequently, the exposure of phosphatidylserine on the plasma membrane turned out to be key mechanisms in PEV generation. It has been shown that lipid-rich microdomains, such as lipid rafts and caveolae, are involved in large EV formation (Biró et al., 2005).
To summarize, remodeling of the cytoskeleton is required for large PEV formation. The reorganization of the cytoskeleton leads to external blebbing of the plasma membrane within lipid-rich microdomains, highly dependent on actin fiber polymerization and their calpain-mediated cleavage (Burger et al., 2013).
Large PEV removal from the circulation has been found to be also caused by phosphatidylserine exposure on the outer membrane leaflet, which not only allows the binding of coagulation factors, but also promotes the elimination of PEVs (Lee et al., 1992; Lemke and Burstyn-Cohen, 2010). Part of PEV clearance takes place in the spleen, so that splenectomized mice show higher levels of CD42+ and phosphatidylserine-exposing circulating large PEVs (Dasgupta et al., 2009).
Platelet-Derived Large Extracellular Vesicles as Mediators in Thrombosis and Hemostasis With Broad Bioactivity
Several studies addressed the mechanism of large PEV generation and their potential bioactivity, with the aim of identifying their biological role and possibly new targets of pharmacological intervention.
PEV generation is dependent on the agonist involved in platelet stimulation (Shai et al., 2012; Milioli et al., 2015; Varon and Shai, 2015). Indeed, the modality of platelet activation is a determinant of size, content, and amount of released PEVs, therefore potentially exerting different effect and having different involvement in the development of diseases associated with platelet activation (Zaldivia et al., 2017).
Large PEVs are important mediators of intracellular communication, transferring their cargo (cytoplasmic or membrane protein, mRNAs, and noncoding RNAs) to target cells (Randriamboavonjy and Fleming, 2018). This explains part of the effects that large PEVs exert in inflammation, thrombosis, immunoregulation, and the transmission of biological information (Boilard et al., 2010). However, the precise mechanism by which PEVs selectively incorporate and release their biological cargo to influence the functionality of target cells needs to be determined (Zaldivia et al., 2017).
Intercellular exchanges of microRNAs mediated by PEVs may modulate gene expression in recipient cells and may determine vascular and tissue response in disease conditions associated with platelet activation (Laffont et al., 2013). Thrombin-activated platelets transfer their miR-223 content to PEVs, which is then delivered to endothelial cells. PEV-derived miR223 regulates the endothelial expression of two of its mRNA target, FBXW7 and EFNA1 (Bartel, 2009; Duchez et al., 2015). High levels of miR-142-3p found in PEVs released by activated platelets, enhance the endothelial cell proliferation/dysfunction via Bcl-2 associated transcription factor (BCLAF)1 (Bao et al., 2018). These results provide a possible mechanism by which activated platelets regulate the function of endothelial cells in hypertension, suggesting a novel potential therapeutic approach based on circulating PEVs.
PEVs may also prove protective in some disease conditions (Berckmans et al., 2001; Owens and Mackman, 2011). PEVs may in fact be involved in remote protection against cardiac ischemia–reperfusion injury (Giricz et al., 2014). Few reports focused on the anticoagulant properties of PEVs. The anticoagulant activity of large PEVs is associated with the binding of the anticoagulant protein S and the activation of protein C. Indeed, protein S specifically binds to PEVs, and the anticoagulant function of the protein C depends on negatively charged surface, therefore acting as potential regulator of the assembly of coagulation factors on PEVs (Dahlbaeck et al., 1992; Somajo et al., 2014). Given the potential procoagulantand anticoagulant effects of large PEVs, the tight regulation of PEV release is likely an important factor regulating the hemostatic process (Zaldivia et al., 2017). Drawing inspiration from structural and mechanistic aspects of the PEVs, Pawlowski et al. (2017) build a liposomal formulation that can be actively anchored to platelet-rich thrombi, releasing encapsulated thrombolytic drugs to generate a site-specific thrombolytic activity. Further molecular and cellular research is warranted to define of the actual effectors of PEVs bioactivity (Ma et al., 2015).
Antiplatelet Agents and Platelet-Derived Large Extracellular Vesicles: Experimental Studies
PEV formation can be induced in vitro by the activation of platelets with agonists, calcium ionophores, phorbol esters, or complement (Sims et al., 1988), but also by a variety of factors including high shear stress (Dachary-Prigent et al., 1995; Holme et al., 1997; Takano et al., 2004), contact with artificial surfaces (Gemmell et al., 1995), and low temperature (Bode and Knupp, 1994). The results from different in vitro studies are consistent with a general model in which EVs are released from platelets activated by soluble agonists with the necessary contribution of integrin engagement and shear stress. All these factors are required to generate large PEVs in vitro (Giacomazzi et al., 2016). Under these conditions, procoagulant activity is induced, as assessed by measuring the binding of annexin V, index of phosphatidylserine expression, which dramatically increases in PEVs (almost all PEVs become positive) after platelet stimulation (Fox et al., 1991; Barry et al., 1997; Perez-Pujol et al., 2007).
To assess the signaling pathways implicated in large PEV generation, an in vitro protocol was set up in our laboratory using antiplatelet agents to investigate the singling pathways implicated in large PEV generation and the potential of antiplatelet agents in reducing their release (Giacomazzi et al., 2016). Platelets generate large PEVs either when stimulated with strong agonists (thrombin, collagen and calcium ionophores), as shown in several independent studies (Fox et al., 1991; Barry et al., 1997; Perez-Pujol et al., 2007), or by weak agonists, such as the thromboxane (TX)A2 analog U46619, ADP, and epinephrine (Judge et al., 2010; Zhang et al., 2013). Under these experimental conditions, shear stress needs to be applied to obtain platelet vesiculation (Giacomazzi et al., 2016).
When platelets are activated by soluble agonists in the presence of aspirin or the TXA2 receptor (TP) antagonist SQ-29,548, a significant reduction in large PEV release is observed, except when ADP or epinephrine is the stimulus. The inhibitory effects of aspirin and SQ-29,548 indicate a role for endogenous TXA2 on agonist-triggered PEV release (Giacomazzi et al., 2016). In the same study, the contribution of ADP secreted from delta granules of activated platelets was assessed using apyrase, an ADP-scavenger enzyme, and PSB-0739, a potent and selective P2Y12 receptor antagonist (Hoffmann et al., 2009). Under these conditions, a significant decrease in large PEV released from platelets stimulated with U46619 and low concentration of collagen is observed, not with high-concentration collagen (Giacomazzi et al., 2016). Since similar effects are observed using apyrase and the specific P2Y12 inhibitor PSB-0739, it can be inferred that P2Y12 is implicated in large PEV generation in response to a large number of agonists. It has also been observed that incubation of normal platelets with aspirin inhibits platelet aggregation and large PEV formation only in response to arachidonic acid, while MeSAMP, a P2Y12 inhibitor, blunts platelet aggregation and PEV release induced by arachidonic acid, TRAP, a thrombin PAR-1 receptor agonist, as well as by ADP (Connor et al., 2016). Also, the active metabolite of prasugrel, a P2Y12 inhibitor, was shown to strongly inhibit collagen- and TRAP-induced large PEV formation, demonstrating the implication ADP and integrin in this process (Judge et al., 2010).
Therefore, both ADP and TXA2 contribute to large PEV release. However, other signaling pathways are implicated in vesiculation, as indicated by the platelet response to epinephrine (Giacomazzi et al., 2016). Large PEV generation does not require platelet secretion, since it has been shown that these two phenomena are dissociated (Delaney et al., 2014). However, large PEV release is entirely dependent on activation of the fibrinogen receptor or an integrin, since eptifibatide, a specific inhibitor of the fibrinogen receptor, prevents the release induced by soluble platelet agonists, except when high-concentration collagen is tested (Giacomazzi et al., 2016). There is evidence that also the engagement of the von Willebrand factor receptor triggers the generation of procoagulant EVs. In fact, the interaction between platelets and immobilized von Willebrand factor leads to the generation of large PEVs under high shear stress (Reininger et al., 2006).
The use of the antiplatelet agents aspirin and a P2Y12 in combination, blocking two different but interconnected signaling pathways involved in the amplification phase of platelet activation, determines a more profound inhibition of large PEV release (Judge et al., 2005). Inhibitory activity has been demonstrated also with ticagrelor, a P2Y12 antagonist that decreases the release of PEVs exposing P-selectin and procoagulant phosphatidylserine, likely decreasing the proinflammatory/procoagulant interaction between PEVs, monocytes, and coagulation factors (Gasecka et al., 2018). In fact, it has been shown that large PEVs bearing P-selectin, able to bind PSGL-1 on monocytes surface, lead to monocyte activation, responsible for cytokine release and the exposure of tissue factor on monocyte (Falati et al., 2003). Large PEVs exposing phosphatidylserine-bound coagulation factors also propagate thrombin generation (Swieringa et al., 2018).
Glycoprotein αIIb/β3 inhibitors and P2Y12 antagonists are additive to aspirin in preventing large PEV production. Both cangrelor, a P2Y12 antagonist, and abciximab, a fibrinogen receptor inhibitor, individually prevented the formation of large PEVs in response to collagen, and the combination of these two agents resulted in further inhibition, while TRAP-induced large PEV formation was insensitive to the effects of aspirin, was reduced by P2Y12 or fibrinogen receptor antagonists, and was further inhibited in the presence of both agents (Judge et al., 2005;; Judge et al., 2008).
Additional evidence derives from animal models. For instance, the inhibition of circulating large PEVs by aspirin has been shown to prevent endothelial injury and the progression of early atherosclerotic lesions in experimental diabetes mellitus, suggesting that PEVs may represent a new target of pharmacological intervention in different clinical settings (Wang et al., 2019).
To summarize, in vitro studies indicate that the inhibitory effects of antiplatelet agents on PEVs may prove beneficial, beyond inhibition of thrombus formation. All these interactions may potentially contribute to the development and progression of atherosclerosis to atherothrombosis (Badimon et al., 2016). Indeed, PEVs, being involved in cell–cell interaction and transfer of proteins and RNAs to different cells, play a role in a variety of pathological processes including thrombosis and hemostasis, inflammation, atherosclerosis, angiogenesis, and tumor progression (Martinez and Andriantsitohaina, 2011;Owens and Mackman, 2011; Baron et al., 2012; Dovizio et al., 2018). This suggests a broad potential benefit of antiplatelet agents as inhibitors of PEV release.
Antiplatelet Agents and Platelet-Derived Large Extracellular Vesicles: Clinical Studies
Clinical studies on the impact of antiplatelet agents on PEV release are limited; most of the available data concern large PEVs and derive from nonrandomized clinical trials. In addition, little information is available concerning the relations between PEV generation and clinical outcomes or the relations between pharmacodynamics of antiplatelet agents and PEV release (Rosińska et al., 2017).
Studies concerning treatment with aspirin gave inconsistent results. The generation of procoagulant large PEVs measured in venous blood and in blood taken at the site of a vascular injury in 13 healthy subjects, as assessed by flow cytometry and immunoassays, was not altered after a 7-day treatment with aspirin 100 mg/day in a randomized crossover study (Lubsczyk et al., 2010). It has been demonstrated that patients treated with aspirin undergoing elective coronary artery stenting have high levels of circulating large PEVs, suggesting that aspirin fails to inhibit the PEV formation in that setting (Kim and Kunapuli, 2011). A single study investigated the effects of aspirin given for 7 days to 24 healthy male and female subjects on the release of small PEVs from ex vivo–stimulated platelets. No effects of aspirin were observed as for small PEV count, but a significant suppression of their cargo protein levels (Goetzl et al., 2016). The concentration of circulating large PEVs, but not other platelet parameters, was found to be decreased after antiplatelet therapy with aspirin 100 mg/day plus cilostazol 200 mg/day, given for 4 weeks to 112 patients with acute ischemic stroke in a nonrandomized clinical trial including 35 control subjects (Chen et al., 2015). In that study, circulating large PEVs were found to be an independent risk factor for the infarct size in a pooled analysis of patients with ischemic stroke after adjustments of other factors including hypertension and diabetes mellitus (Chen et al., 2015).
In comparison with healthy subjects, patients with stable angina show increased release of PEVs that decreases after administration of low-dose aspirin. When tested in vitro in rat thoracic aorta, PEVs collected from patients decreased the tissue expression of ERK1/2 and increased the expression of p38 mitogen-activated protein kinases (MAPKs), c-Jun N-terminal kinases (JNKs), nuclear factor kappa-light-chain-enhancer of activated B cells (NF-kB) and vascular cell adhesion molecule 1 (VCAM-1) and the generation of superoxide anion while decreasing the release of NO. Treatment with aspirin significantly inhibited all these cellular responses, with efficacy similar to that of ERK1/2, p38 MAPKs, and NF-κB inhibitors (Cheng et al., 2017).
Treatment with aspirin (100 mg/day) was shown to decrease the number of circulating endothelial- and platelet-derived large EVs in 15 male patients with coronary artery disease, but nonsignificant coronary artery stenosis, in a nonrandomized clinical study (Bulut et al., 2011).
Furthermore, in 160 male patients with stable coronary heart disease, elevated concentration in peripheral blood of large PEVs bearing tissue factor, together with coexistent hypertriglyceridemia, was among the factors supporting the resistance to the antiplatelet activity of clopidogrel (Jastrzebska et al., 2018).
The inhibitory effects of clopidogrel on large PEVs were found to be related to the maximum plasma concentration reached by clopidogrel active metabolite and the area under the curve observed in a pharmacokinetics/pharmacodynamics study including 26 patients with stable coronary artery disease (França et al., 2012).
Using flow cytometry, the release of large EVs from platelets, monocytes, erythrocytes, and smooth muscle cells was found to be increased in patients with diabetes mellitus. Aspirin therapy reduces biomarkers of vascular wall cell activation and large EV shedding from smooth muscle cells and erythrocytes, but not from platelets, although decreases the number of tissue factor–bearing PEVs with similar effects in 43 patients with types 1 and 2 diabetes mellitus (Chiva-Blanch et al., 2016). Referring to a different clinical setting, recurrent increase in circulating large PEVs was observed after discontinuing aspirin in 17 of 46 patients with Kawasaki disease, a chronic inflammatory arterial disease (Kim et al., 2017).
The additive contribution of platelet P2Y12 receptors in the setting of acute vascular disease is well recognized. Dual antiplatelet therapy with aspirin and a P2Y12 inhibitor is commonly used in these patients to reduce their very high thrombotic risk (Hechler and Gachet, 2015, Nylander and Schulz, 2016), but the effect of this combination therapy on the release of PEVs has not yet been extensively investigated (Connor et al., 2016). In a randomized clinical trial comparing treatment with aspirin alone or in combination with clopidogrel in 70 patients with ischemic stroke, the addition of aspirin to clopidogrel was found to be associated with significant inhibition of collagen-induced platelet aggregation and diminished formation of platelet–monocyte large EVs (Serebruany et al., 2005). Extended-release dipyridamole plus aspirin, clopidogrel plus aspirin, or clopidogrel alone similarly reduced different markers of platelet activation, while only aspirin plus clopidogrel diminished the formation of platelet–monocyte large EVs in a randomized, single-blind clinical trial including patients with type 2 diabetes mellitus and history of transient ischemic attach (Serebruany et al., 2008).
In conclusion, the hypothesis that PEVs may have a pathogenetic role in atherothrombosis is strong. The secondary hypothesis that modulation of PEV generation may contribute to the antithrombotic activity of antiplatelet agents is so far supported by weak experimental evidence. In vitro studies indicate that activation of the arachidonic acid pathway and the release of ADP from delta granules are implicated in the release of large EVs from platelets. Therefore, both aspirin and P2Y12 inhibitors may be beneficial also in vivo by reducing the prothrombotic potential of activated platelets mediated by released PEVs. Since the release of PEVs is strongly dependent on integrin activation, inhibitors of the fibrinogen receptor, von Willebrand factor receptor, and collagen receptor may prove effective in vivo. None of these potential activities have so far been exhaustively demonstrated. A number of clinical trials describe variations in PEV concentration in blood collected from patients treated with antiplatelet agents. However, inconsistency in the study protocols, the small sample size, and differences in the methods used to quantify PEVs represent major limitations. Since PEVs may have a pathogenetic role not only in atherothombosis, but also in other clinical settings, including inflammatory diseases (Boilard et al., 2010) and cancer (Varon and Shai, 2015), broad beneficial effects of antiplatelet intervention may be expected. Further investigation on the efficacy of antiplatelet agents as inhibitors of PEVs with ad hoc–designed controlled clinical trials is warranted.
Author Contributions
All authors listed have made a substantial, direct and intellectual contribution to the work, and approved it for publication.
Funding
The study was supported by a research grant given by Cariverona Foundation to FT.
Conflict of Interest
The authors declare that the research was conducted in the absence of any commercial or financial relationships that could be construed as a potential conflict of interest.
References
Aatonen, M. T., Ohman, T., Nyman, T. A., Laitinen, S., Grönholm, M., Siljander, P. R.-M. (2014). Isolation and characterization of platelet-derived extracellular vesicles. J. Extracell. vesicles 3, 24692–24707. doi: 10.3402/jev.v3.24692
Ambrose, A. R., Alsahli, M. A., Kurmani, S. A., Goodall, A. H. (2018). Comparison of the release of microRNAs and extracellular vesicles from platelets in response to different agonists. Platelets 29, 446–454. doi: 10.1080/09537104.2017.1332366
Anene, C., Graham, A. M., Boyne, J., Roberts, W. (2018). Platelet microparticle delivered microRNA-Let-7a promotes the angiogenic switch. Biochim. Biophys. Acta - Mol. Basis Dis. 1864, 2633–2643. doi: 10.1016/j.bbadis.2018.04.013
Ayers, L., Kohler, M., Harrison, P., Sargent, I., Dragovic, R., Schaap, M., et al. (2011). Measurement of circulating cell-derived microparticles by flow cytometry: Sources of variability within the assay. Thromb. Res. 127, 370–377.
Badimon, L., Suades, R., Fuentes, E., Palomo, I., Padró, T. (2016). Role of Platelet-Derived Microvesicles As Crosstalk Mediators in Atherothrombosis and Future Pharmacology Targets: A Link between Inflammation, Atherosclerosis, and Thrombosis. Front. Pharmacol. 7, 293–310. doi: 10.3389/fphar.2016.00293
Bao, H., Chen, Y. X., Huang, K., Zhuang, F., Bao, M., Han, Y., et al. (2018). Platelet-derived microparticles promote endothelial cell proliferation in hypertension via miR-142-3p. FASEB J. 32, 3912–3923. doi: 10.1096/fj.201701073R
Baron, M., Boulanger, C. M., Staels, B., Tailleux, A. (2012). Cell-derived microparticles in atherosclerosis: Biomarkers and targets for pharmacological modulation? J. Cell. Mol. Med. 16 (7), 1365–1376 doi: 10.1111/j.1582-4934.2011.01486.x
Barry, O. P., Pratico, D., Lawson, J. A., FitzGerald, G. A. (1997). Transcellular activation of platelets and endothelial cells by bioactive lipids in platelet microparticles. J. Clin. Invest. 99, 2118–27. doi: 10.1172/JCI119385
Bartel, D. P. (2009). MicroRNAs: Target Recognition and Regulatory Functions. Cell 136, 215–233. doi: 10.1016/j.cell.2009.01.002
Behan, M. W. H., Fox, S. C., Heptinstall, S., Storey, R. F. (2005). Inhibitory effects of P2Y12 receptor antagonists on TRAP-induced platelet aggregation, procoagulant activity, microparticle formation and intracellular calcium responses in patients with acute coronary syndromes. Platelets 16, 73–80. doi: 10.1080/09537100400005634
Berckmans, R., Nieuwland, R., Böing, A., Romijn, F., Hack, C. E., Sturk, A. (2001). Cell-derived Microparticles Circulate in Healthy Humans and Support Low Grade Thrombin Generation. Thromb. Haemost. 85, 639–649. doi: 10.1055/s-0037-1615646
Beutler, E., Gelbart, T., Kuhl, W. (1990). Interference of heparin with the polymerase chain reaction. Biotechniques 9 (2), 166.
Bevers, E. M., Comfurius, P., Dekkers, D. W., Zwaal, R. F. (1999). Lipid translocation across the plasma membrane of mammalian cells. Biochim. Biophys. Acta 1439, 317–30. doi: 10.1016/s1388-1981(99)00110-9
Biró, E., Akkerman, J. W. N., Hoek, F. J., Gorter, G., Pronk, L. M., Sturk, A., et al. (2005). The phospholipid composition and cholesterol content of platelet-derived microparticles: a comparison with platelet membrane fractions. J. Thromb. Haemost. 3, 2754–2763. doi: 10.1111/j.1538-7836.2005.01646.x
Bode, A., Knupp, C. (1994). Effect of cold storage on platelet glycoprotein Ib and vesiculation. Transfusion 34, 690–696. doi: 10.1046/j.1537-2995.1994.34894353465.x
Boilard, E., Duchez, A.-C., Brisson, A. (2015). The diversity of platelet microparticles. Curr. Opin. Hematol. 22, 437–444. doi: 10.1097/MOH.0000000000000166
Boilard, E., Nigrovic, P. A., Larabee, K., Watts, G. F. M., Coblyn, J. S., Weinblatt, M. E., et al. (2010). Platelets amplify inflammation in arthritis via collagen-dependent microparticle production. Science 327(5965), 580–583. doi: 10.1126/science.1181928
Brisson, A. R., Tan, S., Linares, R., Gounou, C., Arraud, N. (2017). Extracellular vesicles from activated platelets: a semiquantitative cryo-electron microscopy and immuno-gold labeling study. Platelets 28, 263–271. doi: 10.1080/09537104.2016.1268255
Bulut, D., Becker, V., Mügge, A. (2011). Acetylsalicylate reduces endothelial and platelet-derived microparticles in patients with coronary artery disease. Can. J. Physiol. Pharmacol. 89, 239–244. doi: 10.1139/y11-013
Burger, D., Schock, S., Thompson, C. S., Montezano, A. C., Hakim, A. M., Touyz, R. M. (2013). Microparticles: biomarkers and beyond. Clin. Sci. 124, 423–441. doi: 10.1042/CS20120309
Burnouf, T., Goubran, H. A., Chou, M.-L., Devos, D., Radosevic, M. (2014). Platelet microparticles: Detection and assessment of their paradoxical functional roles in disease and regenerative medicine. Blood Rev. 28, 155–166. doi: 10.1016/J.BLRE.2014.04.002
Campello, E., Spiezia, L., Radu, C. M., Dhima, S., Visentin, S., Valle, F. D., et al. (2015). Circulating microparticles in umbilical cord blood in normal pregnancy and pregnancy with preeclampsia. Thromb. Res. 136, 427–431. doi: 10.1016/J.THROMRES.2015.05.029
Castaman, G., Yu-Feng, L., Battistin, E., Rodeghiero, F. (1997). Characterization of a novel bleeding disorder with isolated prolonged bleeding time and deficiency of platelet microvesicle generation. Br. J. Haematol. 96, 458–463. doi: 10.1046/j.1365-2141.1997.d01-2072.x
Chandler, W. L. (2013). Microparticle counts in platelet-rich and platelet-free plasma, effect of centrifugation and sample-processing protocols. Blood Coagul. Fibrinolysis 24, 125–32. doi: 10.1097/MBC.0b013e32835a0824
Chandler, W. L. (2016). Measurement of microvesicle levels in human blood using flow cytometry. Cytom. Part B Clin. Cytom. 90, 326–336. doi: 10.1002/cyto.b.21343
Chandler, W. L., Yeung, W., Tait, J. F. (2011). A new microparticle size calibration standard for use in measuring smaller microparticles using a new flow cytometer. J. Thromb. Haemost. 9, 1216–1224. doi: 10.1111/j.1538-7836.2011.04283.x
Chen, Y., Xiao, Y., Lin, Z., Xiao, X., He, C., Bihl, J. C., et al. (2015). The role of circulating platelets microparticles and platelet parameters in acute ischemic stroke patients. J. Stroke Cerebrovasc. Dis. 24 (10), 2313–2320. doi: 10.1016/j.jstrokecerebrovasdis.2015.06.018
Cheng, G., Shan, X. F., Wang, X. L., Dong, W. W., Li, Z., Liu, X. H., et al. (2017). Endothelial damage effects of circulating microparticles from patients with stable angina are reduced by aspirin through ERK/p38 MAPKs pathways. Cardiovasc. Ther. 34 (4), e12273 doi: 10.1111/1755-5922.12273
Chiva-Blanch, G., Suades, R., Padró, T., Vilahur, G., Peña, E., Ybarra, J., et al. (2016). Microparticle Shedding by Erythrocytes, Monocytes and Vascular Smooth Muscular Cells Is Reduced by Aspirin in Diabetic Patients. Rev. Española Cardiol. (English Ed. 69, 672–680. doi: 10.1016/j.rec.2015.12.033
Christersson, C., Johnell, M., Siegbahn, A. (2010). The In fl uence of Direct Thrombin Inhibitors on the Formation of Platelet-leukocyte Aggregates and Tissue Factor Expression. Thromb. Res. 126, e327–e333. doi: 10.1016/j.thromres.2010.03.019
Cointe, S., Judicone, C., Robert, S., Mooberry, M. J., Poncelet, P., Wauben, M., et al. (2016). Standardization of microparticle enumeration across different flow cytometry platforms: results of a multicenter collaborative workshop. J. Thromb. Haemost. 15 (1), 187–193.
Conde, I., Shrimpton, C. N., Thiagarajan, P., López, J. A. (2005). Tissue-factor–bearing microvesicles arise from lipid rafts and fuse with activated platelets to initiate coagulation. Blood 106, 1604–1611. doi: 10.1182/BLOOD-2004-03-1095
Connor, D. E., Exner, T., Ma, D. D. F., Joseph, J. E. (2010). The majority of circulating platelet-derived microparticles fail to bind annexin V, lack phospholipid-dependent procoagulant activity and demonstrate greater expression of glycoprotein Ib. Thromb. Haemost. 103, 1044–1052. doi: 10.1160/TH09-09-0644
Connor, D. E., Ly, K., Aslam, A., Boland, J., Low, J., Jarvis, S., et al. (2016). Effects of antiplatelet therapy on platelet extracellular vesicle release and procoagulant activity in health and in cardiovascular disease. Platelets 27, 805–811. doi: 10.1080/09537104.2016.1190008
Coumans, F. A. W., Brisson, A. R., Buzas, E. I., Dignat-George, F., Drees, E. E. E., El-Andaloussi, S., et al. (2017). Methodological Guidelines to Study Extracellular Vesicles. Circ. Res. 120 (10), 1632–1648. doi: 10.1161/CIRCRESAHA.117.309417
Dachary-Prigent, J., Pasquet, J.-M., Freyssinet, J.-M., Nurden, A. T. (1995). Calcium involvement in aminophospholipid exposure and microparticle formation during platelet activation: A study using Ca2+-ATPase inhibitors. Biochemistry 34, 11625–11634. doi: 10.1021/bi00036a039
Dahlbaeck, B., Wiedmer, T., Sims, P. J. (1992). Binding of anticoagulant vitamin K-dependent protein S to platelet-derived microparticles. Biochemistry 31, 12769–12777. doi: 10.1021/bi00166a009
Dasgupta, S. K., Abdel-Monem, H., Niravath, P., Le, A., Bellera, R. V., Langlois, K., et al. (2009). Lactadherin and clearance of platelet-derived microvesicles. Blood 113, 1332–1339. doi: 10.1182/blood-2008-07-167148
Delaney, M. K., Liu, J., Kim, K., Shen, B., Stojanovic-Terpo, A., Zheng, Y., et al. (2014). Agonist-induced platelet procoagulant activity requires shear and a Rac1-dependent signaling mechanism. Blood 124, 1957–67. doi: 10.1182/blood-2014-03-560821
Diamant, M., Tushuizen, M. E., Sturk, A., Nieuwland, R. (2004). Cellular microparticles: new players in the field of vascular disease? Eur. J. Clin. Invest. 34(6), 392–401. doi: 10.1111/j.1365-2362.2004.01355.x
Dovizio, M., Alberti, S., Sacco, A., Guillem-Llobat, P., Schiavone, S., Maier, T. J., et al. (2015). Novel insights into the regulation of cyclooxygenase-2 expression by platelet-cancer cell cross-talk. Biochem. Soc. Trans. 43, 707–714. doi: 10.1042/BST20140322
Dovizio, M., Bruno, A., Contursi, A., Grande, R., Patrignani, P. (2018). Platelets and extracellular vesicles in cancer: diagnostic and therapeutic implications. Cancer Metastasis Rev. 37, 455–467. doi: 10.1007/s10555-018-9730-4
Dragovic, R. A., Gardiner, C., Brooks, A. S., Tannetta, D. S., Ferguson, D. J. P., Hole, P., et al. (2011). Sizing and phenotyping of cellular vesicles using Nanoparticle Tracking Analysis. Nanomedicine Nanotechnology, Biol. Med. 7, 780–788. doi: 10.1016/J.NANO.2011.04.003
Duchez, A.-C., Boudreau, L. H., Naika, G. S., Bollinger, J., Belleannée, C., Cloutier, N., et al. (2015). Platelet microparticles are internalized in neutrophils via the concerted activity of 12-lipoxygenase and secreted phospholipase A 2 -IIA. Proc. Natl. Acad. Sci. 112, E3564–E3573. doi: 10.1073/pnas.1507905112
Eichner, N. Z. M., Gilbertson, N. M., Gaitan, J. M., Heiston, E. M., Musante, L., LaSalvia, S., et al. (2018). Low cardiorespiratory fitness is associated with higher extracellular vesicle counts in obese adults. Physiol. Rep. 6, e13701. doi: 10.14814/phy2.13701
Enjeti, A. K., Ariyarajah, A., D’Crus, A., Seldon, M., Lincz, L. F. (2016). Correlative analysis of nanoparticle tracking, flow cytometric and functional measurements for circulating microvesicles in normal subjects. Thromb. Res. 145, 18–23. doi: 10.1016/j.thromres.2016.06.029
Erdbrügger, U., Lannigan, J. (2016). Analytical challenges of extracellular vesicle detection: A comparison of different techniques. Cytom. Part A 89, 123–134. doi: 10.1002/cyto.a.22795
Erdbrügger, U., Rudy, C. K., Etter, M., Dryden, K. A., Yeager, M., Klibanov, A. L., et al. (2014). Imaging flow cytometry elucidates limitations of microparticle analysis by conventional flow cytometry. Cytom. Part A 85, 756–770. doi: 10.1002/cyto.a.22494
Falati, S., Liu, Q., Gross, P., Merrill-Skoloff, G., Chou, J., Vandendries, E., et al. (2003). Accumulation of tissue factor into developing thrombi in vivo is dependent upon microparticle P-selectin Glycoprotein Ligand 1 and platelet P-Selectin. J. Exp. Med. 197 (11), 1585–1598. doi: 10.1084/jem.20021868
Flaumenhaft, R., Dilks, J. R., Richardson, J., Alden, E., Patel-Hett, S. R., Battinelli, E., et al. (2009). Megakaryocyte-derived microparticles: direct visualization and distinction from platelet-derived microparticles. Blood 113, 1112–21. doi: 10.1182/blood-2008-06-163832
Fox, J. E., Austin, C. D., Reynolds, C. C., Steffen, P. K. (1991). Evidence that agonist-induced activation of calpain causes the shedding of procoagulant-containing microvesicles from the membrane of aggregating platelets. J. Biol. Chem. 266, 13289–13295.
França, C. N., Pinheiro, L. F. M., Izar, M. C. O., Brunialti, M. K. C., Salomão, R., Bianco, H. T., et al. (2012). Endothelial progenitor cell mobilization and platelet microparticle release are influenced by clopidogrel plasma levels in stable coronary artery disease. Circ. J. 76, 729–736.
Gajos, K., Kamińska, A., Awsiuk, K., Bajor, A., Gruszczyński, K., Pawlak, A., et al. (2017). Immobilization and detection of platelet-derived extracellular vesicles on functionalized silicon substrate: cytometric and spectrometric approach. Anal. Bioanal. Chem. 409, 1109–1119. doi: 10.1007/s00216-016-0036-5
Gámez-Valero, A., Monguió-Tortajada, M., Carreras-Planella, L., Franquesa, M., Beyer, K., Borràs, F. E. (2016). Size-Exclusion Chromatography-based isolation minimally alters Extracellular Vesicles’ characteristics compared to precipitating agents. Sci. Rep. 6, 33641. doi: 10.1038/srep33641
Gasecka, A., Nieuwland, R., van der Pol, E., Hajji, N., Ćwiek, A., Pluta, K., et al. (2018). P2Y12 antagonist ticagrelor inhibits the release of procoagulant extracellular vesicles from activated platelets: Preliminary results. Cardiol. J. doi: 10.5603/CJ.a2018.0045
Gemmell, C. H., Ramirez, S. M., Yeo, E. L., Sefton, M. V (1995). Platelet activation in whole blood by artificial surfaces: identification of platelet-derived microparticles and activated platelet binding to leukocytes as material-induced activation events. J. Lab. Clin. Med. 125 (2), 276–287.
Gemmell, C. H., Sefton, M. V, and Yeo, E. L. (1993). Platelet-derived microparticle formation involves glycoprotein IIb-IIIa. Inhibition by RGDS and a Glanzmann’s thrombasthenia defect. J. Biol. Chem. 268, 14586–9. doi: 10.4045/tidsskr.10.0728
Giacomazzi, A., Degan, M., Calabria, S., Meneguzzi, A., Minuz, P. (2016). Antiplatelet agents inhibit the generation of platelet-derived microparticles. Front. Pharmacol. 7, 314. doi: 10.3389/fphar.2016.00314
Giricz, Z., Varga, Z., Baranyai, T., Sipos, P., Pálóczi, K., Kittel, Á., et al. (2014). Cardioprotection by remote ischemic preconditioning of the rat heart is mediated by extracellular vesicles. J. Mol. Cell. Cardiol. 68, 75–78. doi: 10.1016/j.yjmcc.2014.01.004
Goetzl, E. J., Goetzl, L., Karliner, J. S., Tang, N., Pulliam, L. (2016). Human plasma platelet-derived exosomes: effects of aspirin. FASEB J. 30, 2058–63. doi: 10.1096/fj.201500150R
Gomes, J., Lucien, F., Cooper, T., Kim, Y., Williams, K., Liao, X., et al. (2018). Analytical Considerations in Nanoscale Flow Cytometry of Extracellular Vesicles to Achieve Data Linearity. Thromb. Haemost. 118, 1612–1624. doi: 10.1055/s-0038-1668544
Grasso, L., Wyss, R., Weidenauer, L., Thampi, A., Demurtas, D., Prudent, M., et al. (2015). Molecular screening of cancer-derived exosomes by surface plasmon resonance spectroscopy. Anal. Bioanal. Chem. 407, 5425–5432. doi: 10.1007/s00216-015-8711-5
György, B., Pálóczi, K., Kovács, A., Barabás, E., Bekő, G., Várnai, K., et al. (2014). Improved circulating microparticle analysis in acid-citrate dextrose (ACD) anticoagulant tube. Thromb. Res. 133, 285–292. doi: 10.1016/j.thromres.2013.11.010
Headland, S. E., Jones, H. R., D’Sa, A. S., Perretti, M., Norling, L. V (2014). Cutting-edge analysis of extracellular microparticles using ImageStream(X) imaging flow cytometry. Sci. Rep. 4, 5237.
Hechler, B., Gachet, C. (2015). Purinergic Receptors in Thrombosis and Inflammation. Arterioscler. Thromb. Vasc. Biol. 35 (11), 2307–2315. doi: 10.1161/ATVBAHA.115.303395
Heijnen, H. F., Schiel, A. E., Fijnheer, R., Geuze, H. J., Sixma, J. J. (1999). Activated platelets release two types of membrane vesicles: microvesicles by surface shedding and exosomes derived from exocytosis of multivesicular bodies and alpha-granules. Blood 94, 3791–3799. doi: 10.1111/jth.13147
Hoffmann, K., Baqi, Y., Morena, M. S., Glänzel, M., Müller, C. E., von Kügelgen, I. (2009). Interaction of new, very potent non-nucleotide antagonists with Arg256 of the human platelet P2Y12 receptor. J. Pharmacol. Exp. Ther. 331, 648–55. doi: 10.1124/jpet.109.156687
Holme, P. A., Orvim, U., Hamers, M. J., Solum, N. O., Brosstad, F. R., Barstad, R. M., et al. (1997). Shear-induced platelet activation and platelet microparticle formation at blood flow conditions as in arteries with a severe stenosis. Arterioscler. Thromb. Vasc. Biol. 17, 646–53.
Hong, C.-S., Funk, S., Muller, L., Boyiadzis, M., Whiteside, T. L. (2016). Isolation of biologically active and morphologically intact exosomes from plasma of patients with cancer. J. Extracell. Vesicles 5, 29289. doi: 10.3402/jev.v5.29289
Horstman, L. L., Ahn, Y. S. (1999). Platelet microparticles: A wide-angle perspective. Crit. Rev. Oncol. Hematol. 30, 111–142.
Hsu, J., Gu, Y., Tan, S. L., Narula, S., DeMartino, J. A., Liao, C. (2013). Bruton’s Tyrosine Kinase mediates platelet receptor-induced generation of microparticles: a potential mechanism for amplification of inflammatory responses in rheumatoid arthritis synovial joints. Immunol. Lett. 150, 97–104.
Italiano, J. E., Mairuhu, A. T., Flaumenhaft, R. (2010). Clinical relevance of microparticles from platelets and megakaryocytes. Curr. Opin. Hematol. 17 (6), 578–584. doi: 10.1097/MOH.0b013e32833e77ee
Iversen, L. V., Østergaard, O., Nielsen, C. T., Jacobsen, S., Heegaard, N. H. H. (2013). A heparin-based method for flow cytometric analysis of microparticles directly from platelet-poor plasma in calcium containing buffer. J. Immunol. Methods 388, 49–59. doi: 10.1016/j.jim.2012.12.001
Jastrzebska, M., Marcinowska, Z., Oledzki, S., Chelstowski, K., Siennicka, A., Klysz, M., et al. (2018). Variable gender-dependent platelet responses to combined antiplatelet therapy in patients with stable coronary-artery disease. J. Physiol. Pharmacol. 69, 595–605. doi: 10.26402/jpp.2018.4.10
Judge, H. M., Buckland, R. J., Holgate, C. E., Storey, R. F. (2005). Glycoprotein IIb/IIIa and P2Y 12 receptor antagonists yield additive inhibition of platelet aggregation, granule secretion, soluble CD40L release and procoagulant responses. Platelets 16, 398–407. doi: 10.1080/09537100500163226
Judge, H. M., Buckland, R. J., Sugidachi, A., Jakubowski, J. A., Storey, R. F. (2008). The active metabolite of prasugrel effectively blocks the platelet P2Y12 receptor and inhibits procoagulant and pro-inflammatory platelet responses. Platelets. 19 (2), 125–133. doi: 10.1080/09537100701694144
Judge, H. M., Buckland, R. J., Sugidachi, A., Jakubowski, J. A., Storey, R. F. (2010). Relationship between degree of P2Y12 receptor blockade and inhibition of P2Y12-mediated platelet function. Thromb. Haemost. 103, 1210–7. doi: 10.1160/TH09-11-0770
Jy, W., Horstman, L. L., Jimenez, J. J., Ahn, Y. S., Biró, E, Nieuwland, R., et al . (2004). Measuring circulating cell-derived micrparticles. J. Thromb. Haemost. 2 (10), 1842–1843. doi: 10.1111/j.1538-7836.2004.00936.x
Kailashiya, J. (2018). Platelet-derived microparticles analysis: Techniques, challenges and recommendations. Anal. Biochem. 546, 78–85. doi: 10.1016/j.ab.2018.01.030
Kailashiya, J., Singh, N., Singh, S. K., Agrawal, V., Dash, D. (2015). Graphene oxide-based biosensor for detection of platelet-derived microparticles: A potential tool for thrombus risk identification. Biosens. Bioelectron. 65, 274–280. doi: 10.1016/J.BIOS.2014.10.056
Kalra, H., Simpson, R. J., Ji, H., Aikawa, E., Altevogt, P., Askenase, P., et al. (2012). Vesiclepedia: A Compendium for Extracellular Vesicles with Continuous Community Annotation. PLoS Biol. 10, e1001450. doi: 10.1371/journal.pbio.1001450
Kim, H. J., Choi, E.-H., Lim, Y. J., Kil, H. R. (2017). The Usefulness of Platelet-derived Microparticle as Biomarker of Antiplatelet Therapy in Kawasaki Disease. J. Korean Med. Sci. 32, 1147. doi: 10.3346/jkms.2017.32.7.1147
Kim, H. K., Song, K. S., Lee, E. S., Lee, Y. J., Park, Y. S., Lee, K. R., et al. (2002). Optimized flow cytometric assay for the measurement of platelet microparticles in plasma: Pre-analytic and analytic considerations. Blood Coagul. Fibrinolysis. 13 (5), 393–397. doi: 10.1097/00001721-200207000-00003
Kim, S., Kunapuli, S. P. (2011). P2Y12 receptor in platelet activation. Platelets 22 (1), 54–58. doi: 10.3109/09537104.2010.497231
Lacroix, R., Robert, S., Poncelet, P., Dignat-George, F. (2010a). Overcoming Limitations of Microparticle Measurement by Flow Cytometry. Semin. Thromb. Hemost. 36, 807–818. doi: 10.1055/s-0030-1267034
Lacroix, R., Robert, S., Poncelet, P., Kasthuri, R. S., Key, N. S., Dignat-George, F. (2010b). Standardization of platelet-derived microparticle enumeration by flow cytometry with calibrated beads: results of the International Society on Thrombosis and Haemostasis SSC Collaborative workshop. J. Thromb. Haemost. 8, 2571–2574. doi: 10.1111/j.1538-7836.2010.04047.x
Laffont, B., Corduan, A., Plé, H., Duchez, A.-C., Cloutier, N., Boilard, E., et al. (2013). Activated platelets can deliver mRNA regulatory Ago2•microRNA complexes to endothelial cells via microparticles. Blood 122, 253–61. doi: 10.1182/blood-2013-03-492801
Lawrie, A. S., Albanyan, A., Cardigan, R. A., Mackie, I. J., Harrison, P. (2009). Microparticle sizing by dynamic light scattering in fresh-frozen plasma. Vox Sang. 96, 206–212. doi: 10.1111/j.1423-0410.2008.01151.x
Lee, K. D., Hong, K., Papahadjopoulos, D. (1992). Recognition of liposomes by cells: in vitro binding and endocytosis mediated by specific lipid headgroups and surface charge density. Biochim. Biophys. Acta 1103, 185–97. doi: 10.1016/0005-2736(92)90086-2
Lemke, G., Burstyn-Cohen, T. (2010). TAM receptors and the clearance of apoptotic cells. Ann. N. Y. Acad. Sci. 1209, 23–29. doi: 10.1111/j.1749-6632.2010.05744.x
Lubsczyk, B., Kollars, M., Hron, G., Kyrle, P. A., Weltermann, A., Gartner, V. (2010). Low dose acetylsalicylic acid and shedding of microparticles in vivo in humans. Eur. J. Clin. Invest. 40, 477–482. doi: 10.1111/j.1365-2362.2010.02299.x
Lvovich, V., Srikanthan, S., Silverstein, R. L. (2010). A novel broadband impedance method for detection of cell-derived microparticles. Biosens. Bioelectron. 26, 444–451. doi: 10.1016/j.bios.2010.07.094
Ma, F., Liu, H., Shen, Y., Zhang, Y., Pan, S. (2015). Platelet-derived microvesicles are involved in cardio-protective effects of remote preconditioning. Int. J. Clin. Exp. Pathol. 8, 10832–9.
Maas, S. L. N., Vrij, J., De, Broekman, M. L. D. (2014). Quantification and size-profiling of extracellular vesicles using tunable resistive pulse sensing. J. Vis. Exp. doi: 10.3791/51623
Martinez, M. C., Andriantsitohaina, R. (2011). Microparticles in angiogenesis: Therapeutic potential. Circ. Res. 109 (1), 110–119. doi: 10.1161/CIRCRESAHA.110.233049
Milioli, M., Ibáñez-Vea, M., Sidoli, S., Palmisano, G., Careri, M., Larsen, M. R. (2015). Quantitative proteomics analysis of platelet-derived microparticles reveals distinct protein signatures when stimulated by different physiological agonists. J. Proteomics 121, 56–66. doi: 10.1016/j.jprot.2015.03.013
Mitrugno, A., Tassi Yunga, S., Sylman, J. L., Zilberman-Rudenko, J., Shirai, T., Hebert, J. F., et al. (2019). The role of coagulation and platelets in colon cancer-associated thrombosis. Am. J. Physiol. Cell Physiol. 316, C264–C273. doi: 10.1152/ajpcell.00367.2018
Mobarrez, F., Antovic, J., Egberg, N., Hansson, M., Jörneskog, G., Hultenby, K., et al. (2010). A multicolor flow cytometric assay for measurement of platelet-derived microparticles. Thromb. Res. 125, e110–e116. doi: 10.1016/j.thromres.2009.10.006
Mody, M., Lazarus, A. H., Semple, J. W., Freedman, J. (1999). Preanalytical requirements for flow cytometric evaluation of platelet activation: choice of anticoagulant. Transfus. Med. 9, 147–154. doi: 10.1046/j.1365-3148.1999.00188.x
Momen-Heravi, F., Balaj, L., Alian, S., Mantel, P. Y., Halleck, A. E., Trachtenberg, A. J., et al. (2013). Current methods for the isolation of extracellular vesicles. Biol. Chem. 394 (10), 1253–1262. doi: 10.1515/hsz-2013-0141
Morel, O., Jesel, L., Freyssinet, J. M., Toti, F. (2011). Cellular mechanisms underlying the formation of circulating microparticles. Arterioscler. Thromb. Vasc. Biol. 31, 15–26. doi: 10.1161/ATVBAHA.109.200956
Morel, O., Morel, N., Freyssinet, J.-M., Toti, F. (2008). Platelet microparticles and vascular cells interactions: a checkpoint between the haemostatic and thrombotic responses. Platelets 19, 9–23. doi: 10.1080/09537100701817232
Morel, O., Toti, F., Hugel, B., Bakouboula, B., Camoin-Jau, L., Dignat-George, F., et al. (2006). Procoagulant microparticles: disrupting the vascular homeostasis equation? Arterioscler. Thromb. Vasc. Biol. 26, 2594–604. doi: 10.1161/01.ATV.0000246775.14471.26
Mørk, M., Pedersen, S., Botha, J., Lund, S. M., Kristensen, S. R. (2016). Preanalytical, analytical, and biological variation of blood plasma submicron particle levels measured with nanoparticle tracking analysis and tunable resistive pulse sensing. Scand. J. Clin. Lab. Invest. 76, 349–360. doi: 10.1080/00365513.2016.1178801
Mustard, J. F., Kinlough-Rathbone, R. L., Packham, M. A. (2002). “History of platelets,” in Platelets in Thrombotic and Non-thrombotic Disorders, eds. Gresele, P. , Page, C. P., Fuster, V., Vermylen, J.(Cambridge: Cambridge University Press), 3–24. doi: 10.1017/CBO9780511545283.002
Nelles, N. J., Chandler, W. L. (2014). Platelet Mapping Assay Interference Due to Platelet Activation in Heparinized Samples. Am. J. Clin. Pathol. 142, 331–338. doi: 10.1309/AJCPQ9BYJ0OEENGA
Nielsen, T., Kristensen, S. R., Gregersen, H., Teodorescu, E. M., Christiansen, G., Pedersen, S. (2019). Extracellular vesicle-associated procoagulant phospholipid and tissue factor activity in multiple myeloma. PLoS One 14, e0210835. doi: 10.1371/journal.pone.0210835
Nieuwland, R., van der Pol, E., Gardiner, C., Sturk, A., (2012). “Platelet-Derived Microparticles,” in Platelets, ed. Michelson, A.D. (San Diego, CA: Academic Press), 453–467. doi: 10.1016/B978-0-12-387837-3.00022-5
Nomura, S., Inami, N., Shouzu, A., Omoto, S., Kimura, Y., Takahashi, N., et al. (2009). The effects of pitavastatin, eicosapentaenoic acid and combined therapy on platelet-derived microparticles and adiponectin in hyperlipidemic, diabetic patients. Platelets 20, 16–22. doi: 10.1080/09537100802409921
Nomura, S., Taniura, T., Shouzu, A., Omoto, S., Suzuki, M., Okuda, Y., et al. (2018). Effects of sarpogrelate, eicosapentaenoic acid and pitavastatin on arterioslcerosis obliterans-related biomarkers in patients with type 2 diabetes (SAREPITASO study). Vasc. Health Risk Manag. doi: 10.2147/VHRM.S171143
Nordin, J. Z., Lee, Y., Vader, P., Mäger, I., Johansson, H. J., Heusermann, W., et al. (2015). Ultrafiltration with size-exclusion liquid chromatography for high yield isolation of extracellular vesicles preserving intact biophysical and functional properties. Nanomedicine Nanotechnology, Biol. Med. 11, 879–883. doi: 10.1016/J.NANO.2015.01.003
Nylander, S., Schulz, R. (2016). Effects of P2Y 12 receptor antagonists beyond platelet inhibition - comparison of ticagrelor with thienopyridines. Br. J. Pharmacol. 173, 1163–1178. doi: 10.1111/bph.13429
Obeid, S., Ceroi, A., Mourey, G., Saas, P., Elie-Caille, C., Boireau, W. (2017). Development of a NanoBioAnalytical platform for "on-chip" qualification and quantification of platelet-derived microparticles. Biosens. Bioelectron. 93, 250–259. doi: 10.1016/j.bios.2016.08.100
Onódi, Z., Pelyhe, C., Terézia Nagy, C., Brenner, G. B., Almási, L., Kittel, Á., et al. (2018). Isolation of high-purity extracellular vesicles by the combination of iodixanol density gradient ultracentrifugation and bind-elute chromatography from blood plasma. Front. Physiol. 9, 1479. doi: 10.3389/fphys.2018.01479.
Ostenfeld, M. S., Jensen, S. G., Jeppesen, D. K., Christensen, L.-L., Thorsen, S. B., Stenvang, J., et al. (2016). miRNA profiling of circulating EpCAM + extracellular vesicles: promising biomarkers of colorectal cancer. J. Extracell. Vesicles 5, 31488. doi: 10.3402/jev.v5.31488
Osumi, K., Ozeki, Y., Saito, S., Nagamura, Y., Ito, H., Kimura, Y., et al. (2001). Development and assessment of enzyme immunoassay for platelet-derived microparticles. Thromb. Haemost. 85, 326–330. doi: 10.1055/s-0037-1615688
Owens, A. P., Mackman, N. (2011). Microparticles in Hemostasis and Thrombosis. Circ. Res. 108, 1284–1297. doi: 10.1161/CIRCRESAHA.110.233056.
Parsons, M. E. M., McParland, D., Szklanna, P. B., Guang, M. H. Z., O’Connell, K., O’Connor, H. D., et al. (2017). a protocol for improved precision and increased confidence in nanoparticle tracking analysis concentration measurements between 50 and 120 nm in biological fluids. Front. Cardiovasc. Med. 4, 68. doi: 10.3389/fcvm.2017.00068
Pawlowski, C. L., Li, W., Sun, M., Ravichandran, K., Hickman, D., Kos, C., et al. (2017). Platelet microparticle-inspired clot-responsive nanomedicine for targeted fibrinolysis. Biomaterials 128, 94–108. doi: 10.1016/j.biomaterials.2017.03.012
Perez-Pujol, S., Marker, P. H., Key, N. S. (2007). Platelet microparticles are heterogeneous and highly dependent on the activation mechanism: studies using a new digital flow cytometer. Cytometry. A 71, 38–45. doi: 10.1002/cyto.a.20354
Pontiggia, L., Steiner, B., Ulrichts, H., Deckmyn, H., Forestier, M., Beer, J. H. (2006). Platelet microparticle formation and thrombin generation under high shear are effectively suppressed by a monoclonal antibody against GPIbα. Thromb. Haemost. 96, 774–80. doi: 10.1160/TH06-07-0367
Randriamboavonjy, V., Fleming, I. (2018). Platelet communication with the vascular wall: role of platelet-derived microparticles and non-coding RNAs. Clin. Sci. 132, 1875–1888. doi: 10.1042/CS20180580
Reininger, A. J., Heijnen, H. F. G., Schumann, H., Specht, H. M., Schramm, W., Ruggeri, Z. M. (2006). Mechanism of platelet adhesion to von Willebrand factor and microparticle formation under high shear stress. Blood 107, 3537–45. doi: 10.1182/blood-2005-02-0618
Rider, M. A., Hurwitz, S. N., Meckes, D. G. (2016). ExtraPEG: A Polyethylene Glycol-based method for enrichment of extracellular vesicles. Sci. Rep. 6, 23978. doi: 10.1038/srep23978
Robert, S., Poncelet, P., Lacroix, R., Arnaud, L., Giraudo, L., Hauchard, A., et al. (2009). Standardization of platelet-derived microparticle counting using calibrated beads and a Cytomics FC500 routine flow cytometer: a first step towards multicenter studies? J. Thromb. Haemost. 7, 190–7. doi: 10.1111/j.1538-7836.2008.03200.x
Rosińska, J., Łukasik, M., Kozubski, W. (2017). The Impact of Vascular Disease Treatment on Platelet-Derived Microvesicles. Cardiovasc. Drugs Ther. 31, 627–644. doi: 10.1007/s10557-017-6757-7
Serebruany, V. L., Malinin, A. I., Pokov, A. N., Hanley, D. F. (2008). Antiplatelet profiles of the fixed-dose combination of extended-release dipyridamole and low-dose aspirin compared with clopidogrel with or without aspirin in patients with type 2 diabetes and a history of transient ischemic attack: A randomized, single-blind, 30-day trial. Clin. Ther. 30, 249–259. doi: 10.1016/j.clinthera.2008.02.006
Serebruany, V. L., Malinin, A. I., Ziai, W., Pokov, A. N., Bhatt, D. L., Alberts, M. J., et al. (2005). Effects of Clopidogrel and Aspirin in combination versus aspirin alone on platelet activation and major receptor expression in patients after recent ischemic stroke. Stroke 36 (10), 2289–2292. doi: 10.1161/01.STR.0000181081.09262.e1
Shah, M. D., Bergeron, A. L., Dong, J.-F., López, J. A. (2008). Flow cytometric measurement of microparticles: Pitfalls and protocol modifications. Platelets 19, 365–372. doi: 10.1080/09537100802054107
Shai, E., Rosa, I., Parguiña, A. F., Motahedeh, S., Varon, D., García, Á. (2012). Comparative analysis of platelet-derived microparticles reveals differences in their amount and proteome depending on the platelet stimulus. J. Proteomics 76, 287–296.
Shantsila, E., Kamphuisen, P. W., Lip, G. Y. H. (2010). Circulating microparticles in cardiovascular disease: implications for atherogenesis and atherothrombosis. J. Thromb. Haemost. 8, 2358–68. doi: 10.1111/j.1538-7836.2010.04007.x
Shi, J., Gilbert, G. E., Church, W. R., Haley, P., Krishnaswamy, S. (2003). Lactadherin inhibits enzyme complexes of blood coagulation by competing for phospholipid-binding sites. Blood 101, 2628–36. doi: 10.1182/blood-2002-07-1951
Shih, C.-L., Chong, K.-Y., Hsu, S.-C., Chien, H.-J., Ma, C.-T., Chang, J. W.-C., et al. (2016). Development of a magnetic bead-based method for the collection of circulating extracellular vesicles. N. Biotechnol. 33, 116–122. doi: 10.1016/j.nbt.2015.09.003
Shirafuji, T., Hamaguchi, H., Higuchi, M., Kanda, F. (2009). Measurement of platelet-derived microparticle levels using an enzyme-linked immunosorbent assay in polymyositis and dermatomyositis patients. Muscle Nerve 39, 586–590. doi: 10.1002/mus.21311
Shirafuji, T., Hamaguchi, H., Kanda, F. (2008). Measurement of platelet-derived microparticle levels in the chronic phase of cerebral infarction using an enzyme-linked immunosorbent assay. Kobe J. Med. Sci. 54 (1), 55–61.
Simpson, R., Mathivanan, S. (2012). Extracellular microvesicles: the need for internationally recognised nomenclature and stringent purification criteria. J. Proteomics Bioinform. 05 (02), p1. doi: 10.4172/jpb.10000e10
Sims, P. J., Faioni, E. M., Wiedmer, T., Shattil, S. J. (1988). Complement proteins C5b-9 cause release of membrane vesicles from the platelet surface that are enriched in the membrane receptor for coagulation factor Va and express prothrombinase activity. J. Biol. Chem. 263, 18205–12.
Sims, P. J., Wiedmer, T., Esmon, C. T., Weiss, H. J., Shattil, S. J. (1989). Assembly of the platelet prothrombinase complex is linked to vesiculation of the platelet plasma membrane. Studies in Scott syndrome: An isolated defect in platelet procoagulant activity. J. Biol. Chem. 264 (29), 17049–17057.
Somajo, S., Koshiar, R. L., Norström, E., Dahlbäck, B. (2014). Protein S and factor V in regulation of coagulation on platelet microparticles by activated protein C. Thromb. Res. 134, 144–152. doi: 10.1016/j.thromres.2014.04.031
Suades, R., Padró, T., Vilahur, G., Badimon, L. (2012). Circulating and platelet-derived microparticles in human blood enhance thrombosis on atherosclerotic plaques. Thromb. Haemost. 108, 1208–1219. doi: 10.1160/TH12-07-0486
Suzuki, J., Umeda, M., Sims, P. J., Nagata, S. (2010). Calcium-dependent phospholipid scrambling by TMEM16F. Nature 468, 834–838. doi: 10.1038/nature09583
Swieringa, F., Spronk, H. M. H., Heemskerk, J. W. M., van der Meijden, P. E. J. (2018). Integrating platelet and coagulation activation in fibrin clot formation. Res. Pract. Thromb. Haemost. 2 (3), 450–460. doi: 10.1002/rth2.12107
Takano, K., Asazuma, N., Satoh, K., Yatomi, Y., Ozaki, Y. (2004). Collagen-induced generation of platelet-derived microparticles in whole blood is dependent on ADP released from red blood cells and calcium ions. Platelets 15, 223–9. doi: 10.1080/09537100410001682797
Tao, S.-C., Guo, S.-C., Zhang, C.-Q. (2017). Platelet-derived Extracellular Vesicles: An Emerging Therapeutic Approach. Int. J. Biol. Sci. 13, 828–834. doi: 10.7150/ijbs.19776
Tatischeff, I., Larquet, E., Falcón-Pérez, J. M., Turpin, P.-Y., Kruglik, S. G. (2012). Fast characterisation of cell-derived extracellular vesicles by nanoparticles tracking analysis, cryo-electron microscopy, and Raman tweezers microspectroscopy. J. Extracell. Vesicles 1, 19179. doi: 10.3402/jev.v1i0.19179
Théry, C., Witwer, K. W., Aikawa, E., Alcaraz, M. J., Anderson, J. D., Andriantsitohaina, R., et al. (2018). Minimal information for studies of extracellular vesicles 2018 (MISEV2018): a position statement of the International Society for Extracellular Vesicles and update of the MISEV2014 guidelines. J. Extracell. Vesicles 7, 1535750. doi: 10.1080/20013078.2018.1535750
Todorova, D., Simoncini, S., Lacroix, R., Sabatier, F., Dignat-George, F. (2017). Extracellular Vesicles in Angiogenesis. Circ. Res. 120, 1658–1673. doi: 10.1161/CIRCRESAHA.117.309681
Unsworth, A. J., Bye, A. P., Tannetta, D. S., Desborough, M. J. R., Kriek, N., Sage, T., et al. (2017). Farnesoid X Receptor and Liver X Receptor Ligands Initiate Formation of Coated Platelets. Arterioscler. Thromb. Vasc. Biol. 37, 1482–1493. doi: 10.1161/ATVBAHA.117.309135
Vajen, T., Mause, S., Koenen, R. (2015). Microvesicles from platelets: novel drivers of vascular inflammation. Thromb. Haemost. 114, 228–236. doi: 10.1160/TH14-11-0962
van der Pol, E., Coumans, F. A. W., Grootemaat, A. E., Gardiner, C., Sargent, I. L., Harrison, P., et al. (2014). Particle size distribution of exosomes and microvesicles determined by transmission electron microscopy, flow cytometry, nanoparticle tracking analysis, and resistive pulse sensing. J. Thromb. Haemost. 12, 1182–1192. doi: 10.1111/jth.12602
van der Pol, E., Harrison, P. (2017). From platelet dust to gold dust: physiological importance and detection of platelet microvesicles. Platelets 28, 211–213. doi: 10.1080/09537104.2017.1282781
van Eijndhoven, M. A., Zijlstra, J. M., Groenewegen, N. J., Drees, E. E., van Niele, S., Baglio, S. R., et al. (2016). Plasma vesicle miRNAs for therapy response monitoring in Hodgkin lymphoma patients. JCI insight 1, e89631. doi: 10.1172/jci.insight.89631
Varon, D., Shai, E. (2015). Platelets and their microparticles as key players in pathophysiological responses. J. Thromb. Haemost. 13, S40–S46. doi: 10.1111/jth.12976
Wang, G., Ma, K., Zhang, Y., Hu, Z., Liu, L., Lu, J., et al. (2019). Platelet microparticles contribute to aortic vascular endothelial injury in diabetes via the mTORC1 pathway. Acta Pharmacol. Sin. 40, 468–476. doi: 10.1038/s41401-018-0186-4
Wolf, P. (1967). The Nature and Significance of Platelet Products in Human Plasma. Br. J. Haematol. 13, 269–288. doi: 10.1111/j.1365-2141.1967.tb08741.x
Xu, R., Greening, D. W., Zhu, H.-J., Takahashi, N., Simpson, R. J. (2016). Extracellular vesicle isolation and characterization: toward clinical application. J. Clin. Invest. 126, 1152–1162. doi: 10.1172/JCI81129
Yano, Y., Shiba, E., Kambayashi, J., Sakon, M., Kawasaki, T., Fujitani, K., et al. (1993). The effects of calpeptin (a calpain specific inhibitor) on agonist induced microparticle formation from the platelet plasma membrane. Thromb. Res. 71, 385–96. doi: 10.1016/0049-3848(93)90163-I
Yuana, Y., Böing, A. N., Grootemaat, A. E., van der Pol, E., Hau, C. M., Cizmar, P., et al. (2015). Handling and storage of human body fluids for analysis of extracellular vesicles. J. Extracell. Vesicles 4, 29260. doi: 10.3402/jev.v4.29260
Yuana, Y., Oosterkamp, T. H., Bahatyrova, S., Ashcroft, B., Garcia Rodriguez, P., BERTINA, R. M., et al . (2010). Atomic force microscopy: a novel approach to the detection of nanosized blood microparticles. J. Thromb. Haemost. 8, 315–323. doi: 10.1111/j.1538-7836.2009.03654.x
Zaldivia, M. T. K., McFadyen, J. D., Lim, B., Wang, X., Peter, K. (2017). Platelet-derived microvesicles in cardiovascular diseases. Front. Cardiovasc. Med. 4, 74. doi: 10.3389/fcvm.2017.00074
Zhang, Y., Liu, X., Liu, L., Zaske, A. M., Zhou, Z., Fu, Y., et al. (2013). Contact-and agonist-regulated microvesiculation of human platelets. Thromb. Haemost. 110, 331–339. doi: 10.1160/TH12-11-0853
Keywords: platelets, microvesicles, aspirin, thromboxane, P2Y12 inhibitors, atherothrombosis
Citation: Taus F, Meneguzzi A, Castelli M and Minuz P (2019) Platelet-Derived Extracellular Vesicles as Target of Antiplatelet Agents. What Is the Evidence? Front. Pharmacol. 10:1256. doi: 10.3389/fphar.2019.01256
Received: 25 July 2019; Accepted: 30 September 2019;
Published: 08 November 2019.
Edited by:
Paola Patrignani, Università degli Studi G. d’Annunzio Chieti e Pescara, ItalyReviewed by:
Emanuela Marcantoni, New York University, United StatesMelania Dovizio, Università degli Studi G. d’Annunzio Chieti e Pescara, Italy
Patricia B. Maguire, University College Dublin, Ireland
Copyright © 2019 Taus, Meneguzzi, Castelli and Minuz. This is an open-access article distributed under the terms of the Creative Commons Attribution License (CC BY). The use, distribution or reproduction in other forums is permitted, provided the original author(s) and the copyright owner(s) are credited and that the original publication in this journal is cited, in accordance with accepted academic practice. No use, distribution or reproduction is permitted which does not comply with these terms.
*Correspondence: Pietro Minuz, pietro.minuz@univr.it
†These authors have contributed equally to this work