- 1Vascular Pharmacology and Metabolism Group (FARMAVASM), Department of Pharmacology, School of Medicine, Universidad Autónoma de Madrid, Madrid, Spain
- 2Instituto de Investigaciones Sanitarias del Hospital Universitario La Paz (IdiPAZ), Madrid, Spain
- 3Laboratory of Vascular Pathology and Diabetes, FIIS-Fundación Jiménez Díaz, Universidad Autónoma Madrid, Madrid, Spain
- 4Spanish Biomedical Research Centre in Diabetes and Associated Metabolic Disorders (CIBERDEM) Network, Madrid, Spain
- 5German Diabetes Center, Institute for Clinical Diabetology, Leibniz Center for Diabetes Research at Heinrich Heine University Düsseldorf, Düsseldorf, Germany
COVID-19 outbreak, caused by severe acute respiratory syndrome (SARS)-CoV-2 coronavirus has become an urgent health and economic challenge. Diabetes is a risk factor for severity and mortality of COVID-19. Recent studies support that COVID-19 has effects beyond the respiratory tract, with vascular complications arising as relevant factors worsening its prognosis, then making patients with previous vascular disease more prone to severity or fatal outcome. Angiotensin-II converting enzime-2 (ACE2) has been proposed as preferred receptor for SARS-CoV-2 host infection, yet specific proteins participating in the virus entry are not fully known. SARS-CoV-2 might use other co-receptor or auxiliary proteins allowing virus infection. In silico experiments proposed that SARS-CoV-2 might bind dipeptidyl peptidase 4 (DPP4/CD26), which was established previously as receptor for MERS-CoV. The renin–angiotensin–aldosterone system (RAAS) component ACE2 and DPP4 are proteins dysregulated in diabetes. Imbalance of the RAAS and direct effect of soluble DPP4 exert deleterious vascular effects. We hypothesize that diabetic patients might be more affected by COVID-19 due to increased presence ACE2 and DPP4 mediating infection and contributing to a compromised vasculature. Here, we discuss the role of ACE2 and DPP4 as relevant factors linking the risk of SARS-CoV-2 infection and severity of COVID-19 in diabetic patients and present an outlook on therapeutic potential of current drugs targeted against RAAS and DPP4 to treat or prevent COVID-19-derived vascular complications. Diabetes affects more than 400 million people worldwide, thus better understanding of how they are affected by COVID-19 holds an important benefit to fight against this disease with pandemic proportions.
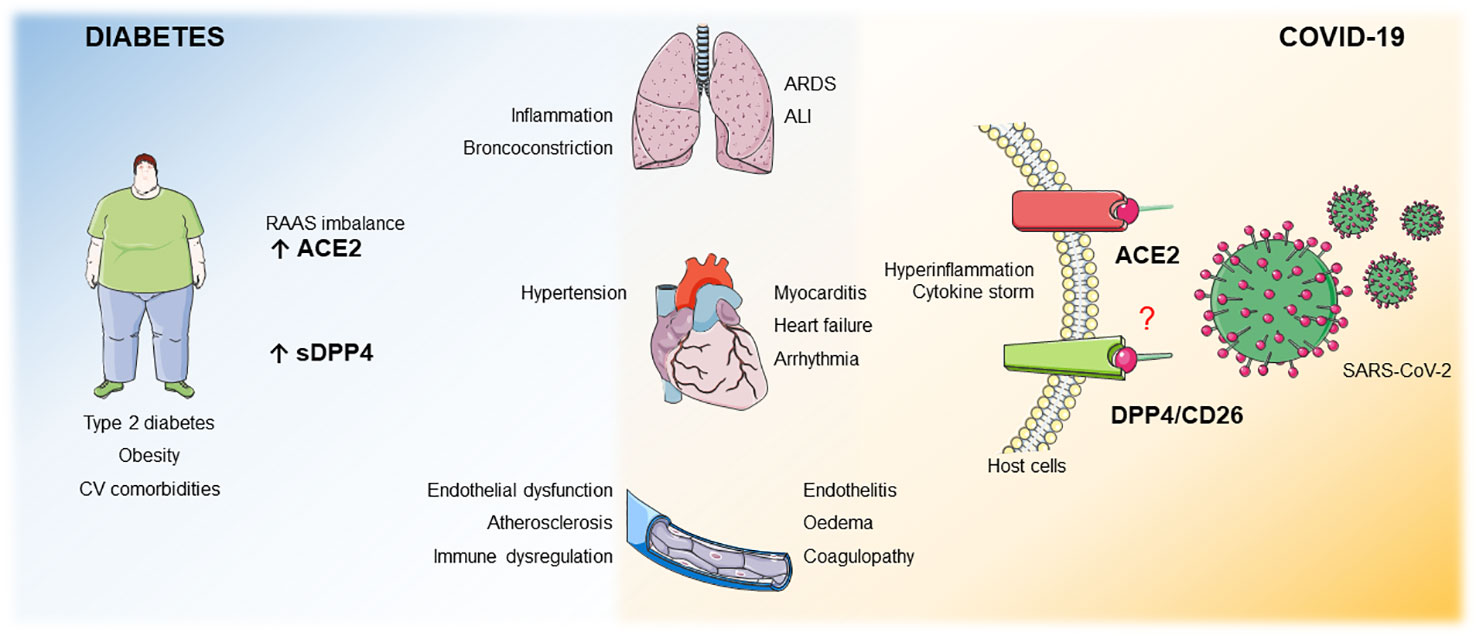
Graphical Abstract Patients with obesity, T2DM or CV comorbidities display an imbalance in the RAAS system and upregulated sDPP4 levels. These 2 factors per se increase the risk for bronchoconstriction, lung inflammation, heart failure, endothelial dysfunction, atherosclerosis and immune dysregulation. ACE2 is a receptor for SARS-CoV-2 and DPP4 has been poposed as potential co/receptor. We hypothesize that in patients with these comorbidities, where both ACE2 and sDPP4 are enhanced, infection with SARS-CoV-2 may result in increased COVID-19 severity with pulmonar complications such as ALI, ARDS and CV complications such as heart failure, arrhythmia, myocarditis, endothelitis, oedema and coagulopathy.
Introduction
Diabetes as Promoter of Severity and Mortality in COVID-19
The recent outbreak of COVID-19 pneumonia in China (Guan et al., 2020; Huang et al., 2020) has become an urgent health and economic challenge due to its pandemic proportions. Therefore, there is a current race for developing strategies to treat or prevent COVID-19 infection. COVID-19 is caused by the new severe acute respiratory syndrome coronavirus 2 (SARS-CoV-2). During the last 20 years other two betacoronaviruses bursted, namely SARS-CoV and middle east respiratory syndrome (MERS)-CoV, yet without such pandemic impact.
Similarly to former influenza infections, there is increasing evidence that diabetes is an important risk factor for the severity and mortality of COVID-19 (Hill et al., 2020; Klonoff and Umpierrez, 2020; Zhou et al., 2020). A meta-analysis pointed towards hypertension and cardiovascular (CV) disease and diabetes as the most prevalent cardiometabolic comorbidities in COVID-19 hospitalized patients (Li B. et al., 2020). The first large cohort of hospitalized patients with COVID-19 in Europe confirmed arterial hypertension, followed by chronic heart disease and diabetes as the main comorbidities upon hospitalisation. While at intensive care unit (ICU) the most frequent comorbidities were hypertension, obesity and diabetes (Borobia, 2020; Vaduganathan et al., 2020). Furthermore, recent studies suggest that obesity may be related to increased COVID-19 severity (Petrilli et al., 2020; Simonnet et al., 2020) even in younger patients (Lighter et al., 2020).
Being CV diseases the main cause of morbi-mortality in type 2 diabetic patients, it is not surprising that besides being comorbidities, obesity, type 2 diabetes mellitus (T2DM) and CV diseases have been suggested as risk factors for severity in COVID-19 in several meta-analysis (Wang B. et al., 2020; Zhang D. et al., 2020). In fact, in a retrospective cohort study including 72.314 COVID-19 patients, those with previous CV comorbidities displayed five-fold higher mortality risk (Wu and McGoogan, 2020).
Since initial epidemiological data pointed towards COVID-19 specially affecting older patients where diabetes, CV diseases and obesity are frequent comorbidities, it is under discussion whether these comorbidities and especially diabetes actually increase the risk of infection or only the severity or if they are just co-existing preconditions more frequently found in the severely affected patients (Ceriello et al., 2020; Fadini et al., 2020).
Pathophysiology of COVID-19: Pulmonar and Cardiovascular Complications
Acute respiratory distress syndrome (ARDS) is the main death cause of COVID-19 (Huang et al., 2020). Autopsy and radiography findings detected acute lung inflammation in patients, characterized by accumulation of infiltrated leukocytes (Li L. et al., 2020). In fact, one of the main causes for ARDS is the cytokine storm, that results in an excessive inflammatory response. Although macrophages do not allow SARS-CoV replication, interaction of SARS-CoV with these cells leads to overproduction of pro‐inflammatory cytokines potentially leading to acute lung injury (ALI) and ARDS (He et al., 2006). Besides macrophages, the role of activated endothelial cells is increasingly acknowledged to be implicated in the inflammatory response in ARDS (Villar et al., 2019), as alveolar–capillary barrier disruption is the key determinant for the progression of severe hypoxemia shown in COVID-19 patients (Li L. et al., 2020). Several epidemiological studies suggest that type 2 diabetic patients display increased risk of developing pulmonary hypertension and worse survival (Kolahian et al., 2019).
Although COVID-19 is primarily acknowledged as a respiratory disease, lung damage can be attributable to a great extent to an initial CV cause such as embolism or pulmonary hypertension. Not only CV complications can be a key cause of death in COVID-19, but those patients with previous CV diseases are at increased risk of death (Guo et al., 2020; Zhou et al., 2020).
In fact, CV impairment manifestations are arising as relevant secondary complications during SARS-CoV-2 infection. On one hand, it has been observed that previous CV conditions worsen upon COVID-19 diagnosis (Guo et al., 2020), although cerebrovascular accidents have intriguingly been also reported in healthy young patients with no history of chronic conditions (Oxley et al., 2020).
The main CV complications reported in COVID-19 are myocardial infarction, arrhythmia, stroke and arterial and venous thromboembolism, including pulmonary embolism (E S Cardiology, 2020; Wang D. et al., 2020). The ability of SARS-CoV-2 to directly infect other cells beyond the lung epithelium causing myocarditis and endothelitis might be the underlying explanation, but also an exacerbated immune and/or coagulation response. SARS-CoV-2 can induce, as other SARS-CoVs, direct damage on the cardiomyocytes, in line to reported interstitial monoculear inflammatory cells infiltration in the myocardium of COVID-19 positive patients (Xu et al., 2020). In fact, cardiac injury has been reported in about 20% of the analysed patients (Guo et al., 2020; Zhou et al., 2020).
A retrospective case series analysing 187 patients in Wuhan observed that patients with underlying CV disease were the ones presenting more severe heart damage, as indicated by elevated troponin T (TnT) and N-terminal pro-brain natriuretic peptide (NT-pro-BNP) levels (Chen et al., 2020; Guo et al., 2020). Indeed, high NT-pro-BNP levels in critially ill patients was also associated with acute respiratory symptoms and increased inflammatory status (Yang C. et al., 2020). Equally, patients with no preexisting heart conditions have shown signs of cardiac damage (Li B. et al., 2020).
Endothelitis was also evidenced by postmortem electron analysis of viral inclusion structures in renal endothelial cells (Varga et al., 2020). In fact, SARS-CoV-2 was able to directly infect engineered human blood vessel organoids in vitro (Monteil et al., 2020). Accumulated inflammatory cells and apoptotic bodies near vascular beds (Varga et al., 2020) lead to altered vascular permeability (E S Cardiology, 2020), an important sign of endothelial dysfunction. Endothelial dysfunction is a hallmark of CV disease found in hypertension, diabetes and obesity. However, SARS-CoV-2 might impair endothelium functionality not only on those patients with previous weakened vasculature, but also in healthy individuals. There are case-reports of patients under 50 presenting sudden strokes even with mild manifestations of COVID-19 onset (Oxley et al., 2020).
Coagulopathy has been diagnosed in several fatal and non-fatal cases, with a number of thrombotic parameters altered in COVID-19 patients, including anti-thrombin activity (AT), pro-thrombin time (PT) and D-dimer (Wang D. et al., 2020; Zhang D. et al., 2020). Reported higher D-dimer levels paralleled massive elevation of pro-coagulant von Willebrand factor and factor VIII, indicating endothelial activation (Escher et al., 2020). Endothelial activation leads to chemokine and cytokine release resulting in recruitment of immune cells, perpetuating and spreading inflammation to neighbouring tissues. In fact, a retrospective analysis of the coagulation features in 183 patients determined that fatal COVID-19 cases displayed disseminated intravascular coagulation (Tang et al., 2020), which is strictly linked to endothelial dysfunction and reinforces the sense of SARS-CoV-2 deteriorating endothelium in the lung and beyond.
Thus, CV complications might represent cause and effect of pulmonary manifestations of COVID-19. Microvascular dysfunction of lung capillaries implies the development of pulmonary oedema due to increased vascular permeability, which also allows SARS-CoV-2 to invade other vascular beds (Varga et al., 2020). In the same way, clots coming from peripheral vessels can migrate to the lungs or even the brain and cause pulmonary thromboembolism or strokes. In fact, evidence in both animals and humans has demonstrated that fibrinolytic therapy in ARDS and ALI improves survival (Wang J. et al., 2020). Therefore, vascular function preservation in COVID-19 treatment, and more specifically, in diabetic patients might improve the disease prognosis.
Angiotensin-converting enzyme-2 (ACE2) is a pivotal protein in the protecting branch of the renin-angiotensin-aldosterone system (RAAS), as it metabolizes angiotensin (Ang) II into its physiological antagonist Ang-(1-7), which oposses its detrimental vascular effects (Santos et al., 2018). In fact, type 2 diabetic patients display an umbalanced AngII/Ang-(1-7) ratio (Srivastava et al., 2019). Analogously, DPP4 upregulation has been associated with vascular pathophysiology in diabetes and obesity (Röhrborn et al., 2015; Wronkowitz et al., 2015). We hypothesize that enhanced circulating levels of soluble DPP4 and misbalanced ACE2 expression found in obesity and T2DM may contribute to the severity of COVID-19 related to these disease/comorbidities. Furthermore, due to their vascular effects, both DPP4 and ACE2/Ang II axis arise as potential therapeutic targets to ARDS and CV complications in COVID-19 that might be pharmacologically scoped, as it will be detailed below.
Mechanisms of SARS-CoV-2 Entry Into Host Cells
Similarly to SARS-CoV, it has been proposed that SARS-CoV-2 enters human cells using ACE2 as receptor (Zhang H. et al., 2020). Once attached to ACE2 via the receptor binding domain (RBD) in the viral spike protein, it is primed by the host serine protease TMPRSS2, which ultimately allows fusion of viral and cellular membranes. Although ACE2 is ubiquosly expressed, with higher expression in the CV system, within the lung it is specifically found in alveolar epithelial cells and lung endothelial cells (Santos et al., 2018).
In the lung, SARS-CoV infects mainly pneumocytes and macrophages (Shieh et al., 2005). The peptidases in the lung epithelium and endothelium have been proposed as primary sites for coronavirus infection once the viral infection progresses in the respiratory tract. In this line, it has been suggested that the use of peptidases by coronaviruses to enter host cells might rely more on their abundance than on their proteolytic activity (Li et al., 2003). However, the specific proteins that facilitate SARS-CoV-2 entry in host cells remain unclear.
It has been suggested that other betacoronavirures require the participation of diverse surface proteins for human cells invasion. Thus, sialic acid receptors and C-type lectin receptor CD209/DC-SIGN, expressed on the surface of macrophages and dendritic cells, participate in SARS-CoV invasion into immune cells in vivo (Li et al., 2003; Kuba et al., 2005). In vitro the transmembrane glycoprotein CD147/basigin has been described to mediate SARS-CoV invasion. Interestingly, the interaction of CD147 and SARS-CoV-2 RBD has been observed and successfully prevented by competitive anti-CD147 treatment (Wang K. et al., 2020).
On the other hand, MERS-CoV binds to human DPP4/CD26 to infect host cells (Raj et al., 2013). A very recent study predicting the structure of the SARS-CoV-2 spike glycoprotein and its glycan shield pattern suggests that DPP4/CD26 may also act as receptor for SARS-CoV-2 (Vankadari and Wilce, 2020). In favor of this hypothesis lays the highest co-expression of DPP4 with ACE2 (Qi et al., 2020). In fact, unique residues of SARS-CoV-2 glycoprotein predicted to bind ACE2 can also interact with DPP4 (Vankadari and Wilce, 2020).
Since MERS-CoV binds to DPP4, a transgenic mouse model expressing human DPP4 on relevant cells as epithelial and alveolar pulmonary cells has been used to study the impact of diabetes on MERS-CoV infection. MERS-CoV-infected diabetic mice expressing DPP4 suffered more severe symptoms for longer time than corresponding infected controls (Kulcsar et al., 2019). Therefore, we hypothesize that DPP4 might represent a link between diabetes and morbi-mortality/severity of COVID-19.
RAAS and sDPP4 in Diabetes and Other Comorbidities
Cardiovascular Effects of ACE-Ang II-AT1R Axis Upregulation and Ang-(1-7)/Mas Axis Dowregulation
The RAAS is a key player in the regulation of CV homeostasis in both health and disease. Ang II is the primary effector of RAAS, upregulated in the context of vascular pathophysiology exerting vasoconstrictor, pro-oxidant, pro-inflammatory, pro-fibrotic and proliferative effects, mostly through AT1 receptors (Romero et al., 2019). ACE2 is a pivotal protein in the protecting branch of RAAS, as it metabolizes Ang II into Ang-(1-7) and Ang I into Ang-(1-9) which is in turn metabolized as Ang-(1-7) by angiotensin-converting enzyme (ACE). Ang-(1-7) opposes to Ang II-induced detrimental vascular effects participating in vasodilator, anti-inflammatory, anti-oxidant, anti-proliferative, anti-thrombotic and anti-senescence responses through Mas receptors (Santos et al., 2018; Romero et al., 2019). Moreover, Ang-(1-7) stimulates pleiotropic cytoprotective pathways (Nrf2-HO-1 axis) (Romero et al., 2019) (Figure 1).
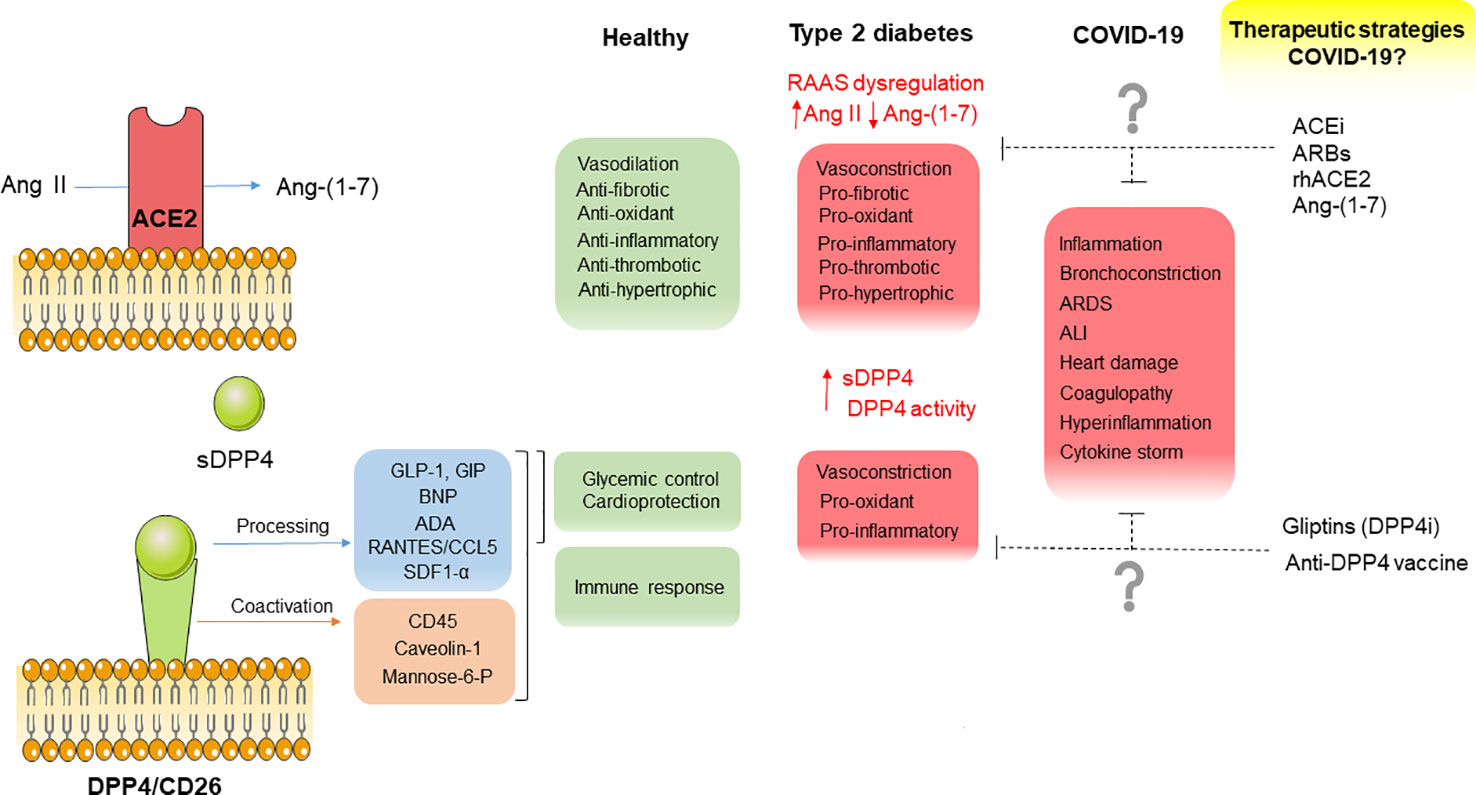
Figure 1 Diagram summarizing the main physio- and pathophysiological functions of ACE2 and DPP4, their potential contribution to CV complications in COVID-19 as well as potential therapeutic strategies interfering with these 2 factors to counteract CV complications in COVID-19 and type 2 diabetic patients.
Under physiological conditions, the RAAS peptides are produced in a balanced manner, whereas misbalance of the RAAS has been implicated in arterial hypertension and diabetes. It has been proposed that increased Ang II can contribute to hyperglycaemia and dyslipidaemia, impairment of vascular function and inflammation. ACE2 deficiency increased vascular inflammation and atherosclerosis in ApoE knockout mice (Thomas et al., 2010). In fact, ACE2-Ang-(1-7)/Mas axis was shown to participate in the reduction of obesity-induced inflammation and chronic renal failure (Santos et al., 2018). In addition, Ang-(1-7) can exert anti-inflammatory effects over RAAS-independent pro-inflammatory stressors as interleukin (IL)-1β (Villalobos et al., 2016; Romero et al., 2019).
SARS-CoV spike glycoprotein injection into mice decreased ACE2 expression levels, worsening lung injury (Kuba et al., 2005). Similarly, SARS-CoV-2-ACE2 binding induced ACE2 internalization (Zhang H. et al., 2020), resulting in Ang II accumulation and insufficient Ang-(1-7) synthesis. Importantly, ACE2 is also directly down-regulated by pro-inflammatory cytokines (Lang et al., 2006). ACE2 serves both as entry receptor and protective mediator in the lung (Monteil et al., 2020), in concordance with data observing decreased ACE2 expression and activity in human idiopathic pulmonary fibrosis (Li et al., 2008). Taking that ACE2 is highly expressed in the endothelium and heart (Santos et al., 2018), decreased ACE2 levels may also lead to imbalanced RAAS signalling and potential disruption of CV homeostasis in COVID-19 patients.
Cardiovascular Effects of sDPP4 Upregulation
DPP4/CD26 is a serine protease cleaving a wide variety of substrates including the incretin hormones glucagon-like peptide 1 (GLP-1) and gastric inhibitory peptide (GIP), cytokines and growth factors (Röhrborn et al., 2015). DPP4 can be found as a membrane-bound form or be shedded as a soluble form (sDPP4) that maintains its enzymatic activity (Röhrborn et al., 2015). We hypothesize that enhanced circulating sDPP4 may promote T2DM-related severity of COVID-19.
In the context of obesity and T2DM, both AT and liver have been proposed as relevant sources of sDPP4 in humans, although the main source remains under discussion. Our group identified sDPP4 as a novel adipose-derived factor (Lamers et al., 2011), whose circulating levels are enhanced in visceral fat from obese and insulin-resistant patients and correlate with BMI (Sell et al., 2013). While sDPP4 plasma activity and levels have been associated with liver apoptosis, fibrosis, fat content, and NAFLD (Baumeier et al., 2017; Romacho et al., 2020).
DPP4 can promote local and systemic inflammation through its immunomodulatory activity. Thus, DPP4 triggers T cell activation, proliferation and cytokine production alone (Focosi et al., 2020) or through the interaction with immune partners on antigen presenting cells as CD45, caveolin-1, manose-6 phosphate receptor, or adenosine deaminase (ADA) (Röhrborn et al., 2015; Williams et al., 2015) (Figure 1). DPP4 expression is enhanced on blood T lymphocytes from type 2 diabetic patients and correlates with insulin resistance and glycated hemoglobin (Lee et al., 2013). sDPP4 treatment enhanced LPS-induced tumor necrosis factor (TNF)-α and IL-6 secretion in activated monocytes. Besides its upregulation in T2DM and obesity, DPP4 expression is increased in senescent cells (Kim et al., 2017).
Immunomodulatory effects of DPP4 have been also demonstrated in animal models. Exogenous injected sDPP4 increased monocyte migration in vivo in a model of low-density-lipoprotein receptor-deficient mice. Interestingly, upregulation of DPP4 in obese and diabetic animals have evidenced also its role on immune responses dysregulation. In this regard, genetic depletion of DPP4 improved adipose tissue inflammation in dark agouti rats (Röhrborn et al., 2015; Romacho et al., 2020).
Some DPP4 substrates with CV impact are upregulated in obesity and/or T2DM as well as in COVID-19 such as CXCL5/RANTES and BNP (Niraula et al., 2018; Adela et al., 2019). In fact, high BNP levels were positively correlated with the incidence of CV events and mortality in HIV-infected patients, and was suggested as an indicator of patients condition deterioration from mild to severe prognosis also in COVID-19 (Yang C. et al., 2020).
DPP4 is also known to interact with extracellular matrix (ECM) proteins, participating in both cell migration and tissue remodelling. By binding to fibronectin, DPP4 can promote T cell helper migration and accummulation in areas with increased ECM proteins, such as damaged blood vessels (Aroor et al., 2013). DPP4 also interacts with fibroblast activation protein (FAP)-α/seprase leading to migration and invasion of endothelial cells into collagen matrices (Ghersi et al., 2006). Moreover, stromal cell-derived factor alpha (SDF-1α) is also a substrate of DPP4, and inhibition of DPP4 has been observed to increase SDF-1α/CXCR4-induced mobilization of endothelial cells from bone marrow to injured vascular sites (Aroor et al., 2013).
Via its interaction with ADA, DPP4 activates plasminogen-2 leading to increased plasmin levels which contributes to degradation of ECM proteins and the activation of matrix metalloproteinase (MMP)-4, which facilitates immune cells migration and diapedesis (Kameoka et al., 1993; Röhrborn et al., 2015). It has been recently proposed that plasmin among other proteases may cleave a new furin site in the spike protein of SARS-CoV-2, leading to an increase in infectivity and severity. This excess of plasmin may contribute to the hyperfibrionolysis resulting in enhanced D-dimer in severe patients (Ji et al., 2020).
Importantly, besides its immunomodulatory effects, sDPP4 can exert direct deleterious effects on the vascular wall. sDPP4 directly promoted human smooth muscle cell (hVSMC) proliferation and inflammation via NF-κB activation resulting in the upregulation of pro-inflammatory cytokines such as monocyte chemoattractant protein (MCP)-1, IL-6 and IL-8, through a novel mechanism mediated by the activation of the protease activated receptor (PAR)-2 (Wronkowitz et al., 2014). DPP4 is itself expressed in the vasculature (Zhong et al., 2015). In hVSMC DPP4 shedding is stimulated by hypoxia in parallel to MMP-1 expression (Röhrborn et al., 2014). Moreover, PAR2 activation induced by sDPP-4 triggered endothelial dysfunction in mesenteric microvessels through thromboxane A2 release mediated by cyclooxygenase (COX) and the thromboxane A2 receptor (TP) activation (Romacho et al., 2016).
Thus we hypothesize that DPP4 expression may contribute to T2DM-related severity of SARS-CoV-2 infection. Furthermore, due to its vascular effects, sDPP4 arises as a potential contributor to ARDS severity by potentially inducing inflammation and bronchoconstriction.
Therapeutic Potential of RAAS-Targeted Drugs in Diabetic Patients With COVID-19
ACEis and ARBs
Despite the initial concerns about ACE inhibitors (ACEi) and AT1 receptor blockers (ARB) treatment potentially predisposing patients to SARS-CoV-2 infection and/or increased COVID-19 severity, the European Medicines Agency advised to continue those prescriptions (EMA/143324/2020; EMA/284513/2020). ACEi/ARBS are primarily prescribed for treating hypertension and associated left ventricular dysfunction, employed in about 35% of the hypertensive population in China (Vaduganathan et al., 2020).
SARS-CoV-2-ACE2 binding leads to ACE2 downregulation and concomitant misbalance of Ang II/Ang-(1-7) ratio towards Ang II-induced detrimental effects. Ang II binding to AT1 receptors increases pulmonary vascular permeability contributing to lung pathology, as previously shown in relation to SARS-CoV lethality (Kuba et al., 2005). Some reports observed that ACEi fail to inhibit ACE2 (Turner et al., 2002) and suggested that small peptide specific ACE2 inhibitors as DX600 as more efficient inhibiting SARS-CoV cell entry in vitro (Guy et al., 2005). Nevertheless, ACEi or AT1R may induce ACE2 upregulation as detected both in animal models at mRNA and protein level (Sukumaran et al., 2011), as well as urinary excretion in olmesartan-treated hypertensive patients (Furuhashi et al., 2015). Taking that increased ACE2 expression also leads to increased Ang-(1-7) production, chronic ACEi and ARB may protect COVID-19 patients. A former study showed that ARB prevented ALI damage in SARS-CoV-infected mice (Kuba et al., 2005). Accordingly, previous studies have shown that ACEi treatment is associated with a diminished risk of pneumonia in both type 1 and type 2 diabetic patients (van de Garde et al., 2007).
In this line, recent studies have demonstrated that the risk of severe COVID-19 was decreased in patients taking ARB in comparison to no-hypertensive patients (Lu et al., 2020; Meng et al., 2020). Interestingly, ACEi/ARB-treated COVID-19 positive patients displayed lower concentrations of CRP and procalcitonin (Yang G. et al., 2020), suggesting that the benefit of ACEi and ARB might rely on their anti-inflammatory effects. Indeed, ARB treatment in rats reduced plasma TNF-α, IL-1β and IL-6 levels and increase IL-10 (Simões e Silva et al., 2013). The ongoing clinical trial BRACE-CORONA (NCT04364893) will compare the impact of temporary discontinuation of ACEi or ARB on mortality and days of hospital stay on patients with SARS-CoV-2 infection (Lopes et al., 2020).
Ang-(1-7) Analogues
Restoring the balance between the RAAS peptides may be an efficient pharmacological approach against COVID-19. The loss of protective Ang-(1-7) as a result of reduced ACE2 availability, may exaggerate the hyper-inflammatory environment in the lung and beyond. Ang-(1-7) supplementation may reveal as an effective pharmacological strategy to attenuate severe CV complications in COVID-19, as we have recently hypothesized (Peiró and Moncada, 2020). In animal models of ALI and ARDS, Ang-(1-7) has demonstrated anti-inflammatory and anti-fibrotic effects via, at least in part, the G protein-coupled receptor Mas (Klein et al., 2013; Santos et al., 2018). Accordingly, administration of Ang-(1-7) and its synthetic analogue AVE 00991 have been reported to reduce leukocyte adhesion to the microvascular endothelium in a model of arthritis (Simões e Silva et al., 2013).
Indeed, the COVID-ARA2 (NCT04337190) and ATCO clinical trial (NCT04332666) will evaluate Ang II blockade and Ang-(1-7) supplementation in the context of lung disease in COVID-19 patients.
rhACE2 as Decoy Factor
It has been demonstrated that recombinant human ACE2 (rhACE2), already tested in phase 2 clinical trials for ARDS/ALI treatment (NCT01597635), blocks SARS-CoV-2 infection in Vero cells and binding to both human blood vessels and human kidney organoids (Monteil et al., 2020). Indeed, the recombinant sACE2 drug will be used in an interventional clinical trial over 200 COVID-19 patients (APN01-COVID-19, NCT04335136).
However, rhACE2 has been suggested not only to hamper SARS-CoV-2 entry into host cells but also potentially to protect from lung injury and vascular dysfunction. ACE2 administration lowered blood pressure and improved endothelial function in an Ang-(1-7)-mediated manner in a model of spontaneously hypertensive stroke-prone rats (SHSPR) (Focosi et al., 2020). In this line, direct activation of ACE2 by NCP-2454 improved pulmonary arterial compliance in a model of pulmonary hypertension (Haga et al., 2015). Moreover, administration of rhACE2 normalized Ang II levels in isolated human hearts with dilated cardiomyopathy (Basu et al., 2017).
Therapeutic Potential of Targeting DPP4 in Diabetic Patients With COVID-19
Gliptins
During COVID-19 infection vascular functionality is endangered. Therefore, preservation of vascular integrity might be a key priority. In case DPP4 represents a link between diabetes and the severity of COVID-19, DPP4i may hold additional therapeutic value to protect diabetic patients suffering COVID-19, as recently especulated (Drucker, 2020; Iacobellis, 2020).
DPP4i, the so-called gliptins, are antidiabetic drugs based on the control of glucose homeostasis through inhibition of DPP4 enzymatic activity. DPP4 cleaves and inactivates the incretin hormones GLP-1 and GIP, which account for up to 60–70% of postprandial insulin release. This way DPP4i prolong the half life of incretins. Besides its action on incretins, gliptins have been proposed to exert other off-target effects including CV effects. Regarding DPP4 inhibition as a therapeutic strategy to treat and prevent CV diseases in obese and/or type 2 diabetic patients there is still an ongoing discussion. Importantly, gliptins are preferred as add-on therapy in individuals with diabetes coursing with previous chronic kidney disease or additional CV disease (Hanssen and Jandeleit-Dahm, 2019).
CV safety of gliptins has been confirmed in several CV outcome trials (SAVOR TIMI 53, NCT01107886; EXAMINE, NCT00968708; TECOS, NCT00790205; CARMELINA, NCT01897532). In relation to their glycemic action, gliptins have demonstrated no risk of hypoglycemic episodes, neutral influence on body weight and a significant reduction of glycated hemoglobin levels (HbA1c), which are important factors associated with reduced CV risk and mortality (Zoungas et al., 2010). Moreover, DPP4i have positive effects over surrogate vascular endpoints, namely blood pressure, lipemia and endothelial function, albeit these CV outcome trials failed in demonstrating additional benefits of gliptins on major CV events (Scheen, 2018). However, gliptins are widely used as T2DM treatment. It has been shown that combined metfomin and gliptin therapy significantly decreased the relative risk of non-fatal CV events, CV mortality and all-cause mortality, in comparison to other oral antihyperglycemic drugs as sulfonylureas (Wang et al., 2017).
Preclinical studies have shed light on the mechanisms by which gliptins might improve CV functionality. Increased bioavailability of substrates of DPP4 such as GLP-1 confers indirect cardioprotective effects to gliptins. Beyond GLP-1, DPP4 inhibition increases the effects of other substrates with cardiovascular impact. T2DM patients trated with sitagliptin displayed higher SDF-1α plasma levels resulting in increased endothelial progenitor cells (EPCs), which play a key role in vascular repair (Fadini et al., 2010). In vitro, SDF-1α was also able to improve blood flow in a model of peripheral artery disease (Segers et al., 2011). Equally, an increase in BNP improved the regulation of natriuresis and the vasodilatory responses (Brandt et al., 2006).
In our hands, both hVSMC proliferation and inflammation as well as ex vivo endothelial dysfunction exerted by sDPP4 were equally prevented by the experimental and clinically available DPP4i K579 and linagliptin, respectively (Wronkowitz et al., 2014; Romacho et al., 2016) That suggests that these drugs may add CV benefit beyond regulating glucose homeostasis and in a GLP-1-independent manner.
In animal models of diabetes, gliptins have also proven protective effects. Vildagliptin-treated streptozotocin (STZ)-induced diabetic rats presented reduced oxydative stress and ICAM-1 and plasminogen activator inhibitor type-1 (PAI-1) expression (Maeda et al., 2012). In db/db mice linagliptin improved cardiac dysfunction by reducing the activation of the Nlrp3/ASC inflammasome and the upregulation of collagen-1 and collagen-3 (Birnbaum et al., 2019). Similarly, sitagliptin also improved cardiac function in mice (Picatoste et al., 2013). Anti-oxidant properties of DPP4i have been also shown under acute inflammation in a mouse model of LPS-induced sepsis (Beckers et al., 2017).
In type 2 diabetic patients, sitagliptin treatment reduced the molecular markers of inflammation as CRP and IL-6 in mononuclear cells (Makdissi et al., 2012), as well as circulating levels of TNF-α, IL-1β, IL-6, intracellular adhesion molecules and CRP (Tremblay et al., 2014). Sitagliptin also impoved the flow-mediated vasodilation in diabetic adults (Barchetta et al., 2019). Importantly, sitaglipitin conferred cardioprotection in diabetic patients with chronic kidney disease (CKD) reducing the Ang II/Ang-(1-7) ratio (Beraldo et al., 2019).
Gliptins can preserve endothelial function by their reported anti-inflammatory, anti-oxidant and potentially protective effects on the vascular system (Avogaro and Fadini, 2018), which are beneficial aspects in the fight against COVID-19. Additionally, a meta-analysis of large randomized clinical trials demonstrated that gliptin treatment did not increase the risk of general infections in type 2 diabetic patients (Yang et al., 2016).
Besides offering potential CV protection, gliptins might be also employed to restrain SARS-CoV-2 binding to host cells. DPP4i ability to hamper coronavirus entry to host cells has been studied. Sitagliptin, vildagliptin or saxagliptin could not block MERS-CoV access to Vero cells (Raj et al., 2013). Contrarily, sitagliptin was able to hamper MERS-CoV infection in macrophages by reversing the immunosuppressive capacity of the virus (Al-Qahtani et al., 2017).
All approved gliptins are competitive reversible inhibitors of DPP4 enzymatic activity (Deacon, 2011). However, gliptins can be categorized in 3 classes based on the subsites within the DPP4 molecule they occupy. Class 1 comprises peptidomimetic DPP4i with the most basic binding to DPP4 such as vildagliptin and saxagliptin. While class 2 inhibitors (alogliptin and linagliptin) and class 3 inhibitors (sitagliptin and teneligliptin) display an increased number of interacting points. The more interacting points the higher inhibitory potency (Nabeno et al., 2013). DPP4 extracelullar structure consists of two domains comprised of a eight γ-bladded β-propeller domain and a C-terminal α,β-hydrolase domain with the catallytic triad (Asp708, His740, Ser630) (Röhrborn et al., 2015). Conserved aminoacids on DPP4 catalytic site are displayed in pockets S1 and S2. However, the catalytic pocket does not colocalize with the proposed binding sites of SARS-CoV-2 to DPP4 (Vankadari and Wilce, 2020). Nevertheless, previous structural studies regarding MERS-CoV-DPP4 interaction demonstrated that residues in the β-propeller domain of DPP4 (Arg54–Asn497) were essential for virus S1 glycoprotein binding, and some of them are also shared by SARS-CoV-2 (Vankadari and Wilce, 2020).
The residue Asp542 was ascertained as a binding point of SARS-CoV-2 to DPP4, as it was also demonstrated for MERS-CoV (Vankadari and Wilce, 2020). Interestingly, class 1 and 2 gliptins (saxagliptin, alogiptin and linagliptin) are able to bind near this residue (Tyr547) in a zone beyond DPP4 pockets S1 and S2 called S1’ subsite (Nabeno et al., 2013). Uracil ring of alogliptin and linagliptin cause a conformational change on DPP4 S1’ subsite hampering its enzymatic activity (Nabeno et al., 2013). Therefore, this type of xhantine-based non-peptidomimetic gliptins could potentially impair SARS-CoV-2 binding to one of its preferred sites in DPP4. In addition, linagliptin, and to a lesser extent vildagliptin, have been predicted to bind ACE2 with same or even better binding energy than they have for DPP4 (Abouelkheir and El-Metwally, 2019), suggesting additional benefit as SARS-CoV-2 therapy. Whether if more complex class 3 gliptins could exert a steric hindrance to SARS-CoV-2 acess into DPP4 needs further investigation. In this line, a very recent approved clinical trial SIDIACO (NCT 04365517) will study the clinical response of T2DM patients with COVID-19 treated with sitagliptin.
DPP4 immunomodulatory function raises the question whether DPP4i might alter the immune response in diabetic patients (Shao et al., 2020). The pharmakokinetic and pharmacodynamic properties of DPP4i allow that DPP4 enzymatic activity in tissues and circulation is not completey blocked (Drucker, 2020). Both DPP4 inhibition with des-fluro sitagliptin and genetic deletion of DPP4 in mice did not alter T-cell dependent immune responses (Vora et al., 2009). Sitagliptin, vildagliptin and saxagliptin did not alter innate immune response triggered by Toll-like receptor (TLR) activation in terms of secretion, co-stimulation, T cell proliferation and migration in human cells in vitro and in mice in vivo (Bergman et al., 2006).
An initial meta-analysis reported that although DPP4i is not associated with an increased risk for respiratory infections they could increase the risk of urinary tract and nasopharyngitis infection (Amori et al., 2007). A more recent meta-analysis assessing the impact of long term use of sitagliptin in T2DM patients determined that there was not an enhanced risk of infection (Gooßen and Gräber, 2012). Another study compared the risk of respiratory tract infections among glucose-lowering therapies and found no increased risk linked to DPP4i in T2DM patients (Gamble et al., 2018). However the potential induction of leucopenia (Pitocco et al., 2010), angioedema (Gosmanov and Fontenot, 2009) or cough in asthma (Baraniuk and Jamieson, 2010) by DPP4i represent potential pitfalls to their use as therapy in COVID-19 (Pitocco et al., 2020). Although 14 years after the approval of DPP4i there have not been major safety issues related to long term use of gliptins. Nevertheless further clinical research will help determine if DPP4i can affect infection risk, T cell development and immune homeostasis in T2DM (Klemann et al., 2016).
sDPP4 as Soluble Decoy Factor
Exogenous administration of sDPP4 inhibited MERS-CoV infection in VERO cells (Raj et al., 2013). Therefore, in case it is demonstarted that SARS-CoV-2 can bind DPP4, exogenous acute administration of sDPP4 as a decoy factor to compete for binding of the virus to endogenous DPP4 in COVID-19 target tissues could be explored.
sDPP4 load benefit might not only rely on virus trapping but also on the blockade of detrimental pathways affecting/worsening immune responses and importantly, to potentially prevent COVID-19 effects on the vasculature. In this line, exogenous administration of sDPP4 was effective to bind to ADA and prevent the formation of endogenous DPP4/CD26-ADA complex in human dendritic cells/macrophages resulting in impeded activation and proliferation of T-cells in vitro (Zhong et al., 2013). Disruption of RANTES/CCL5-CCR5 axis with the anti-CCR5 antibody leronlimab reduced IL-6 plasma leves and plasma viral load in COVID-19 patients (Patterson et al., 2020). It has been proposed that sDPP4 may directly truncate CCL5/RANTES impeding its union to CCR5 (Iwata et al., 1999) therefore sDPP4 may potentially cause the same effect as leronlimab treatment, leading to an improved immune response and reduced cytokine storm.
In the case of diabetic patients where significantly increased sDPP4 plasma concentration are already present (Sell et al., 2013), the potential risk-benefit of exogenous administration of sDPP4 as decoy factor should be carefully investigated.
Anti-DPP4 Vaccine
Another therapeutic approach proven succcesful to regulate plasma DPP4 activity in mice is anti-DPP4 vaccination. Anti-DPP4 vaccine did not cause any side effect on immune cell activation nor immune-mediated attack towards DPP4-expressing cells or tissues. Furthermore it was able to reach comparable effects to gliptin treatment in regard to glycaemia control and GLP-1 plasma levels in mice models of both type 1 and type 2 diabetes (Pang et al., 2014; Li et al., 2017). In this line, a humanized IgG1 monoclonal antibody (YS110) with high affinity for CD26 was evaluated in a phase I clinical trial, with demonstrated no effect on T-cell proliferation and cytokine production in vitro on human CD26-positive lymphocytes (Angevin et al., 2017). Interestingly, YS110 also inhibited MERS-CoV infection (Ohnuma et al., 2013). However, it has been discussed that in vivo administration of anti-DPP4 antibodies could neutralize plasma sDPP4 before it can coate cellular DPP4 and impede virus entry. In this situation, intranasal application of sDPP4 or anti-DPP4 antibodies has been presented as a plausible solution to overcome such effects (Xia et al., 2014).
On the other hand, DPP4/CD26-related signaling was successfully blocked by the soluble fusion protein Caveolin-Ig, which demonstrated additional immunosuppresive effects (Ohnuma et al., 2009). Tissue-factor pathway inhibitor (TFPI) is a biological blocker of DPP4. Morever, due to its anticoagulant properties (Mast, 2016), TFPI could be seen as a positive aspect in the context of COVID-19 treatment, albeit potential clinical applications have to be carefully evaluted due to its reported role in cancer (Fei et al., 2017).
Combined Therapeutic Potential Targeting RAAS and DPP4
DPP4 signalling and RAAS dysregulation bidirectional crosstalk has been proposed previously. Ang II was able to induce DPP4 activity in vitro and in vivo (Aroor et al., 2016). Equally, DPP4 inhibition counteracted RAAS overestimulation in a model of renal ablated rats, where sitagliptin treatment increased Ang-(1-7) and ACE2 expression and activity and reduced AT1 receptor expression and Ang II levels (Beraldo et al., 2019). Thus it has been proposed that DPP4i can attenuate the progression of CV disease by opposing RAAS negative effects.
ACE2 and DPP4 present common features as endopeptidases even sharing a wide variety of experimental inhibitors (Abouelkheir and El-Metwally, 2019). Hence, the idea of combining inhibitory properties of ACEi and DPP4i against COVID-19 could be appealing. However, it has been observed that DPP4i therapy, when combined with high-dose ACEi, could counter the blood pressure lowering effect of ACEi (Aroor et al., 2013). Combination of ACEi and DPP4i seems unreasonable due to reported production of angioedema upon coadministration (Brown et al., 2009). Angioedema is a potentially fatal adverse reaction as characteristic swelling is generally located around face and neck endangering block of upper airways. Both proteases ACE2 and DPP4 have the capacity to proteolyze bradykinin and substance P leading to accumulation of such vasoactive kinins inducing vasodilatation and increase in vascular permeability (Brown et al., 2009). Contrarily, single ACEi or gliptin-associated angioedema is rare (0.1–0.7%) and in some cases disappeared after changing to a different type in the same drug class.
On the other hand, it has been suggested that combination of ARB and DPP4i holds a more effectve therapy for the management of hypertension, renal function and glycaemic control in T2DM (Aroor et al., 2013). The combination of linagliptin and telmisartan induce sinergistic protective effects in the CV functionality of nephrectomized rats (Beraldo et al., 2019).
Therefore, against SARS-CoV-2 the combination of virus entry blockers (ACEi or DPP4i) and virus capturing decoy factors (rhACE2, sDPP4) would be a better option. However, new perspectives into de design of multitarget drugs holds an important therapeutic oportunity as demonstrated by the synthesis of an enalapril-sitagliptin merged compound, which exhibited dual inhibitiory action against human ACE and DPP4 (Abouelkheir and El-Metwally, 2019), with expected reduced drug-drug interactions and undesirable side effects. Moreover, a milk-derived heptapeptide was found to bind and inhibit both ACE and DPP4 activity (Ashok et al., 2019).
Conclusion
Obese and type 2 diabetic patients are at higher risk of SARS-CoV-2 severity since both conditions favour CV complications per se. Therefore, in the meantime vaccines are being developed and anti-viral drugs are investigated, therapeutic strategies to fight COVID-19-induced CV alterations are urgently needed.
It is well known that in T2DM and the metabolic syndrome there is a counterbalance in the RAAS system. Therefore, strategies aiming to regulate this counterbalance may be a useful pharmacological strategy to prevent endothelial cell activation and lung damage in COVID-19 (Table 1). Some studies suggest that ARB and ACEi may also contribute to increase Ang-(1-7) and reduce the risk of severity of COVID-19. Another potential strategy is to provide direct Ang-(1-7) supplementation with analogues to attenuate severe cardiovascular complications in COVID-19 as recently published by us (Peiró and Moncada, 2020). The role of ACE2 as SARS-CoV-2 receptor, may lead to reduced ACE2 availability and potentially resulting in hyperinflammation. The use of rhACE2 as decoy receptor deserves further investigation.
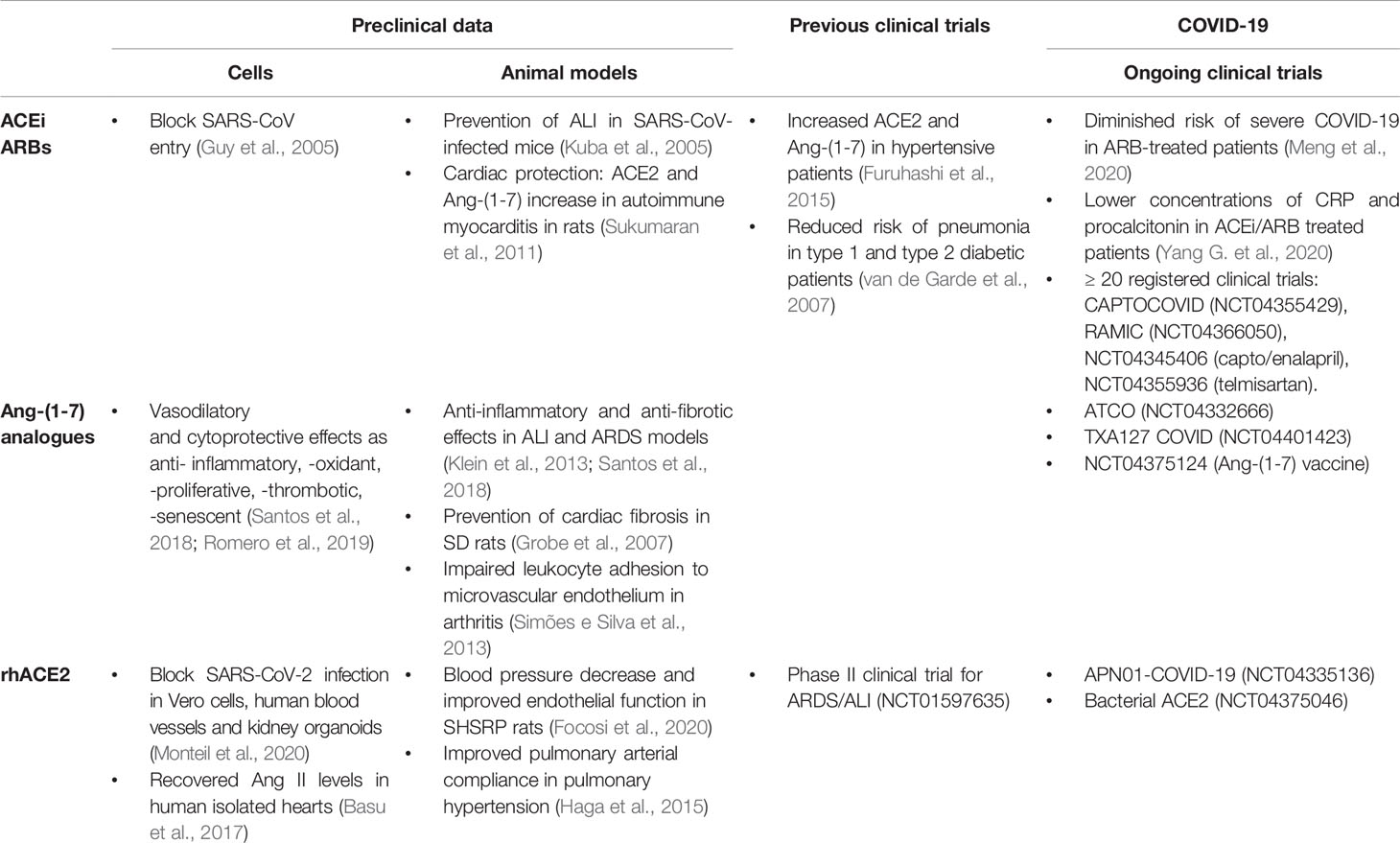
Table 1 Summarized effects of RAAS-targeted drugs (ACEi, ARBS, Ang-(1-7) analogues and rhACE2) reported in preclinical (in vivo and ex vivo) and clinical research.
Similarly, in the case it is confirmed that DPP4 plays a role in SARS-CoV-2 infection as co-receptor, the use of sDPP4 as decoy receptor could also be explored. However, in obesity and T2DM sDPP4 levels are upregulated, sDPP4-induced immunomodulatory actions might be altered and there is a reduced bioavailability of beneficial substrates, facing COVID-19 CV consequences might be a difficult task. Other potential therapeutic approaches worth exploring are DPP4i in order to determine if they can block virus entry, as described for sitagliptin in MERS. Irregardless of DPP4 role as co-receptor, gliptins might help prevent CV complications in COVID-19 due to their anti-inflammatory effects at vascular level. Anti-DPP4 vaccine has been proven effective in mice to control glucose homeostasis and might represent another potential approach.
In conclusion, further preclinical research and clinical data will help to better understanding of the SARS-CoV-2 virus (Table 2), and will allow to determine if any of these potential therapeutic strategies is useful.
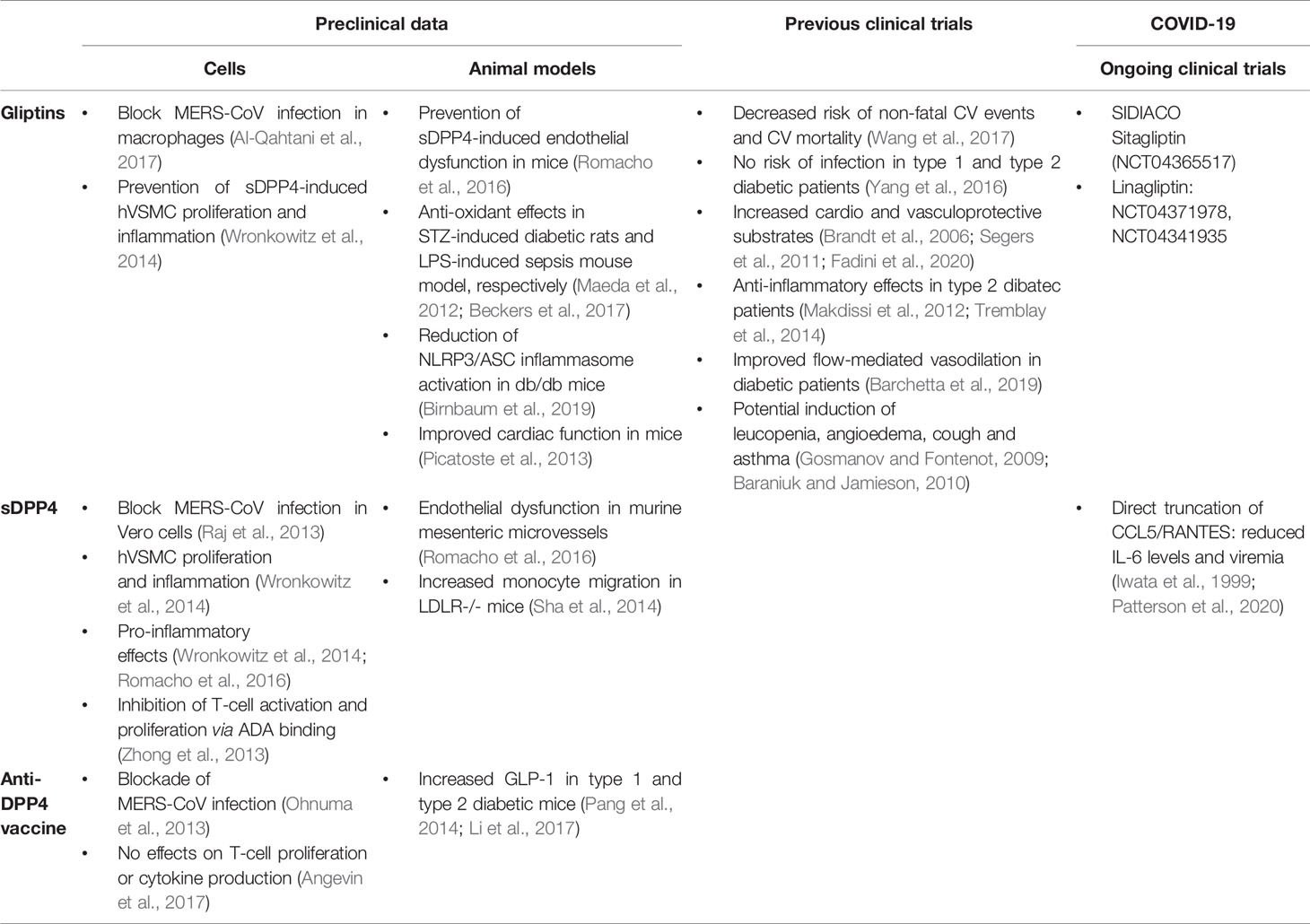
Table 2 Summarized effects of sDPP4-targeted drugs (gliptins, sDPP4 and DPP4 vaccine) reported in preclinical (in vivo and ex vivo) and clinical research.
Author Contributions
IV coordinated and wrote the manuscript. TR conceived, coordinated, and wrote the manuscript. CP, OL, CS-F, and JE wrote the manuscript. All authors discussed the main ideas and provided intellectual input of the complete version of the manuscript. All authors contributed to the article and approved the submitted version.
Funding
IV is the recipient of a FPU fellowship (FPU16/02612). TR and JE are supported by KomIT-Center of Competence for Innovative Diabetes Therapy- funded by EFRE-NRW. CP and CS-F are supported by a grant from Plan Nacional de I+D (SAF2017-84776-R).
Conflict of Interest
The authors declare that the research was conducted in the absence of any commercial or financial relationships that could be construed as a potential conflict of interest.
Abbreviations
ACE2, angiotensin converting enzyme 2; ACEi, angiotensin converting enzyme 2 inhibitors; ALI, acute lung injury; Ang II, angiotensin II; Ang-(1-7), angiotensin-(1-7); ARB, angiotensin receptor 1 blockers; ARDS, acute respiratory distress syndrome; AT, anti-thrombin; ATR1, angiotensin receptor 1; ATR2, angiotensin receptor 2; BNP, brain natriuretic peptide; CCL5, chemokine (C-C Motif) ligand 5; COVID-19, coronavirus disease-19; CRP, C-reactive protein; CV, cardiovascular; DPP4, dipeptidyl peptidase 4; DPP4i, dipeptidyl peptidase 4 inhibitors; GIP, gastric inhibitory peptide; GLP-1, glucagon-like peptide-1; IL, interleukin; ICU, intensive care unit; LDLR, low-density-lipoprotein receptor; MERS, Middle East respiratory syndrome; Mrc1, macrophage mannose receptor 1; NO, nitric oxide; NT-pro-BNP, N-terminal pro-brain natriuretic peptide; PT, pro-thrombin; RAAS, renin-angiotensin aldosterone system; RANTES, regulated upon activation, normal T cell expressed and presumably secreted; RBD, receptor binding domain; SARS-CoV-2, severe acute respiratory syndrome coronavirus-2; STZ, streptozotocin; rhACE2, recombinant human angiotensin converting enzyme 2; sDPP4, soluble dipeptidyl peptidase 4; T2DM, type 2 diabetes mellitus; TnT, troponin T; VCAM-1, vascular cell adhesion molecule-1.
References
Abouelkheir, M., El-Metwally, T. H. (2019). Dipeptidyl peptidase-4 inhibitors can inhibit angiotensin converting enzyme. E. J. Pharmacol. 862, 172638. doi: 10.1016/j.ejphar.2019.172638
Adela, R., Reddy, P. N. C., Ghosh, T. S., Aggarwal, S., Yadav, A. K., Das, B., et al. (2019). Serum protein signature of coronary artery disease in type 2 diabetes mellitus. J. Trans. Med. 17, 17. doi: 10.1186/s12967-018-1755-5
Al-Qahtani, A. A., Lyroni, K., Aznaourova, M., Tseliou, M., Al-Anazi, M. R., Al-Ahdal, M. N., et al. (2017). Middle east respiratory syndrome corona virus spike glycoprotein suppresses macrophage responses via DPP4-mediated induction of IRAK-M and PPARγ. Oncotarget 8, 9053–9066. doi: 10.18632/oncotarget.14754
Amori, R. E., Lau, J., Pittas, A. G. (2007). Efficacy and Safety of Incretin Therapy in Type 2 Diabetes: Systematic Review and Meta-analysis. JAMA 298, 194–206. doi: 10.1001/jama.298.2.194
Angevin, E., Isambert, N., Trillet-Lenoir, V., You, B., Alexandre, J., Zalcman, G., et al. (2017). First-in-human phase 1 of YS110, a monoclonal antibody directed against CD26 in advanced CD26-expressing cancers. B. J. Cancer 116, 1126–1134. doi: 10.1038/bjc.2017.62
Aroor, A., McKarns, S., Nistala, R., DeMarco, V., Gardner, M., Garcia-Touza, M., et al. (2013). DPP-4 Inhibitors as Therapeutic Modulators of Immune Cell Function and Associated Cardiovascular and Renal Insulin Resistance in Obesity and Diabetes. Cardioren Med. 3, 48–56. doi: 10.1159/000348756
Aroor, A., Zuberek, M., Duta, C., Meuth, A., Sowers, J. R., Whaley-Connell, A., et al. (2016). Angiotensin II Stimulation of DPP4 Activity Regulates Megalin in the Proximal Tubules. Int. Med. Mol. Sci. 17, 780. doi: 10.3390/ijms17050780
Ashok, A., Brijesha, N., Aparna, H. S. (2019). Discovery, synthesis, and in vitro evaluation of a novel bioactive peptide for ACE and DPP-IV inhibitory activity. E. J. Med. Chem. 180, 99–110. doi: 10.1016/j.ejmech.2019.07.009
Avogaro, A., Fadini, G. P. (2018). The pleiotropic cardiovascular effects of dipeptidyl peptidase-4 inhibitors. B. J. Clin. Pharmacol. 84, 1686–1695. doi: 10.1111/bcp.13611
Baraniuk, J. N., Jamieson, M. J. (2010). Rhinorrhea, cough and fatigue in patients taking sitagliptin. Alergy Asthma Clin. Immunol. 6, 8. doi: 10.1186/1710-1492-6-8
Barchetta, I., Ciccarelli, G., Barone, E., Cimini, F. A., Ceccarelli, V., Bertoccini, L., et al. (2019). Greater circulating DPP4 activity is associated with impaired flow-mediated dilatation in adults with type 2 diabetes mellitus. Metab. Cardiovasc. Dis. 29, 1087–1094. doi: 10.1016/j.numecd.2019.07.010
Basu, R., Poglitsch, M., Yogasundaram, H., Thomas, J., Rowe, B. H., Oudit, G. Y. (2017). Roles of Angiotensin Peptides and Recombinant Human ACE2 in Heart Failure. JACC 69, 805–819. doi: 10.1016/j.jacc.2016.11.064
Baumeier, C., Schlüter, L., Saussenthaler, S., Laeger, T., Rödiger, M., Alaze, S. A., et al. (2017). Elevated hepatic DPP4 activity promotes insulin resistance and non-alcoholic fatty liver disease. Mol. Metab. 6, 1254–1263. doi: 10.1016/j.molmet.2017.07.016
Beckers, P., Gielis, J., Schil, P., Adriaensen, D. (2017). Lung ischemia reperfusion injury: the therapeutic role of dipeptidyl peptidase 4 inhibition. Ann. Trasn. Med. 5, 129–135. doi: 10.21037/ATM.2017.01.41
Beraldo, J., II, Benetti, A., Borges-Júnior, F. A., Arruda-Junior, D. F., Martins, F. L., Jensen, L., et al. (2019). Cardioprotection Conferred by Sitagliptin Is Associated with Reduced Cardiac Angiotensin II/Angiotensin-(1-7) Balance in Experimental Chronic Kidney Disease. Int. J. Mol. Sci. 20, 1940. doi: 10.3390/ijms20081940
Bergman, A. J., Stevens, C., Zhou, Y. Y., Ramael, S., Wagner, J. A., Herman, G. A. (2006). Pharmacokinetic and pharmacodynamic properties of multiple oral doses of sitagliptin, a dipeptidyl peptidase-IV inhibitor: A double-blind, randomized, placebo-controlled study in healthy male volunteers. Clin. Ther. 28, 55–72. doi: 10.1016/j.clinthera.2006.01.015
Birnbaum, Y., Tran, D., Bajaj, M., Ye, Y. (2019). DPP-4 inhibition by linagliptin prevents cardiac dysfunction and inflammation by targeting the Nlrp3/ASC inflammasome. Basic Res. Cardiol. 114, 1–22. doi: 10.1007/s00395-019-0743-0
Borobia, A. M., Carcas, A. J., Arnalich, F., Álvarez-Sala, R., Monserrat-Villatoro, J., Quintana, M., et al (2020). A cohort of patients with COVID-19 in a major teaching hospital in Europe. J. Clin. Med. 9, 1733. doi: 10.3390/jcm9061733
Brandt, I., Lambeir, A., Ketelslegers, J., Vanderheyden, M., Scharpe, S., De Meester, I. (2006). Dipeptidyl-Peptidase IV Converts Intact B-Type Natriuretic Peptide into Its des-SerPro Form. Med. Chem. 52, 82–87. doi: 10.1373/clinchem.2005.057638
Brown, N. J., Byiers, S., Carr, D., Maldonado, M., Warner, B. A. (2009). Dipeptidyl Peptidase-IV Inhibitor Use Associated With Increased Risk of ACE Inhibitor-Associated Angioedema. Hypertension 54, 516–523. doi: 10.1161/HYPERTENSIONAHA.109.134197
Ceriello, A., Pantea Stoian, A., Rizzo, M. (2020). COVID-19 and diabetes management: what should be considered? Diabetes Res. Clin. Pract. 163, 108151. doi: 10.1016/j.diabres.2020.108151
Chen, C., Chen, C., Yan, J. T., Zhou, N., Zhao, J. P., Wang, D. W. (2020). Analysis of myocardial injury and underlying cardiovascular diseases in critically ill patients with novel coronavirus pneumonia. Chin J. Cariovasc. Dis. 48. doi: 10.3760/cma.j.cn112148-20200225-00123
Deacon, C. F. (2011). Dipeptidyl peptidase-4 inhibitors in the treatment of type 2 diabetes: a comparative review. Diabetes Obes. Metab. 13, 7–18. doi: 10.1111/j.1463-1326.2010.01306.x
Drucker, D. (2020). Coronavirus infections and type 2 diabetes-shared pathways with therapeutic implications. Endocrin. Rew. 41, 457–470. doi: 10.1210/endrev/bnaa011
E S Cardiology (2020). European Society of Cardiology Guidance for the Diagnosis and Management of Heart Disease During COVID-19 (Washington, D.C: Targeted News Service).
Escher, R., Breakey, N., Lämmle, B. (2020). Severe COVID-19 infection associated with endothelial activation. Thromb. Res. 190, 62. doi: 10.1016/j.thromres.2020.04.014
Fadini, G. P., Boscaro, E., Albiero, M., Menegazzo, L., Frison, V., de Kreutzenberg, S., et al. (2010). The Oral Dipeptidyl Peptidase-4 Inhibitor Sitagliptin Increases Circulating Endothelial Progenitor Cells in Patients With Type 2 Diabetes. Diabetes Care 33, 1607–1609. doi: 10.2337/dc10-0187
Fadini, G. P., Morieri, M. L., Longato, E., Avogaro, A. (2020). Prevalence and impact of diabetes among people infected with SARS-CoV-2. J. Endocrin. Invest. 43, 867–869. doi: 10.1007/s40618-020-01236-2
Fei, X., Wang, H., Yuan, W., Wo, M., Jiang, L. (2017). Tissue Factor Pathway Inhibitor-1 Is a Valuable Marker for the Prediction of Deep Venous Thrombosis and Tumor Metastasis in Patients with Lung Cancer. BioMed. Rest Int. 2017, 8983763. doi: 10.1155/2017/8983763
Focosi, D., Tuccori, M., Maggi, F. (2020). Ace Inhibitors and AT1R Blockers for COVID-2019: Friends or Foes? Preprint 20200440151. doi: 10.20944/preprints202004.0151.v1
Furuhashi, M., Moniwa, N., Mita, T., Fuseya, T., Ishimura, S., Ohno, K., et al. (2015). Urinary Angiotensin-Converting Enzyme 2 in Hypertensive Patients May Be Increased by Olmesartan, an Angiotensin II Receptor Blocker. AJHYPE 28, 15–21. doi: 10.1093/ajh/hpu086
Gamble, J., Donnan, J. R., Chibrikov, E., Twells, L. K., Midodzi, W. K., Majumdar, S. R. (2018). Comparative Safety of Dipeptidyl Peptidase-4 Inhibitors Versus Sulfonylureas and Other Glucose-lowering Therapies for Three Acute Outcomes. Sci. Rep. 8, 15142–15110. doi: 10.1038/s41598-018-33483-y
Ghersi, G., Zhao, Q., Salamone, M., Yeh, Y., Zucker, S., Chen, W. (2006). The Protease Complex Consisting of Dipeptidyl Peptidase IV and Seprase Plays a Role in the Migration and Invasion of Human Endothelial Cells in Collagenous Matrices. Cancer Res. 66, 4652–4661. doi: 10.1158/0008-5472.CAN-05-1245
Gooßen, K., Gräber, S. (2012). Longer term safety of dipeptidyl peptidase-4 inhibitors in patients with type 2 diabetes mellitus: systematic review and meta-analysis. Diabetes Obes. Metab. 14, 1061–1072. doi: 10.1111/j.1463-1326.2012.01610.x
Gosmanov, A. R., Fontenot, E. C. (2009). SitagliptinAssociated Angioedema. Hypertension 54, 516–523. doi: 10.1161/HYPERTENSIONAHA.109.134197
Grobe, J. P., Mecca, A. P., Lingis, M., Shenoy, V., Bolton, T. A., Machado, J. M., et al. (2007). Prevention of angiotensin II-induced cardiac remodeling by angiotensin-(1–7). AMJP 292, 736–742. doi: 10.1152/ajpheart.00937.2006
Guan, W., Ni, Z., Hu, Y., Hu, Y., Liang, Z., Liang, W., et al. (2020). Clinical Characteristics of Coronavirus Disease 2019 in China. NEJM 382, 1708–1720. doi: 10.1056/NEJMoa2002032
Guo, T., Fan, Y., Chen, M., Wu, X., Zhang, L., He, T., et al. (2020). Cardiovascular Implications of Fatal Outcomes of Patients With Coronavirus Disease 2019 (COVID-19). JAMA Cardiol. 5, 811–818. doi: 10.1001/jamacardio.2020.1017
Guy, J. L., Jackson, R. M., Jensen, H. A., Hooper, N. M., Turner, A. J. (2005). Identification of critical active-site residues in angiotensin-converting enzyme-2 (ACE2) by site-directed mutagenesis. FEBS 272, 3512–3520. doi: 10.1111/j.1742-4658.2005.04756.x
Haga, S., Tsuchiya, H., Hirai, T., Hamano, T., Mimori, A., Ishizaka, Y. (2015). A novel ACE2 activator reduces monocrotaline-induced pulmonary hypertension by suppressing the JAK/STAT and TGF-β cascades with restored caveolin-1 expression. Exp. Lung Res. 41, 21–31. doi: 10.3109/01902148.2014.959141
Hanssen, N. M., Jandeleit-Dahm, K. A. (2019). Dipeptidyl peptidase-4 inhibitors and cardiovascular and renal disease in type 2 diabetes: What have we learned from the CARMELINA trial? Diabetes Vasc. Dis. Res. 16, 303–309. doi: 10.1177/1479164119842339
He, L., Ding, Y., Zhang, Q., Che, X., He, Y., Shen, H., et al. (2006). Expression of elevated levels of pro-inflammatory cytokines in SARS-CoV-infected ACE2+ cells in SARS patients: relation to the acute lung injury and pathogenesis of SARS. J. Pathol. 210, 288–297. doi: 10.1002/path.2067
Hill, M. A., Mantzoros, C., Sowers, J. R. (2020). Commentary: COVID-19 in patients with diabetes. Metabol. Clin. Exp. 107, 154217. doi: 10.1016/j.metabol.2020.154217
Huang, C., Wang, Y., Li, X., Ren, L., Zhao, J., Hu, Y., et al. (2020). Clinical features of patients infected with 2019 novel coronavirus in Wuhan, China. Lancet 395, 497–506. doi: 10.1016/S0140-6736(20)30183-5
Iacobellis, G. (2020). COVID-19 and diabetes: Can DPP4 inhibition play a role? Diabetes Res. Clin. Pract. 162, 108125. doi: 10.1016/j.diabres.2020.108125
Iwata, S., Yamaguchi, N., Munakata, Y., Ikushima, H., Lee, J. F., Hosono, O., et al. (1999). CD26/dipeptidyl peptidase IV differentially regulates the chemotaxis of T cells and monocytes toward RANTES: possible mechanism for the switch from innate to acquired immune response. Inter. Immunol. 11, 417–426. doi: 10.1093/intimm/11.3.417
Ji, H., Zhao, R., Matalon, S., Matthay, M. A. (2020). Elevated Plasmin(ogen) as a Common Risk Factor for COVID-19 Susceptibility. Physiol. Rev. 100, 1065–1075. doi: 10.1152/physrev.00013.2020
Kameoka, J., Tanaka, T., Nojima, Y., Schlossman, S. F., Morimoto, C. (1993). Direct Association of Adenosine Deaminase with a T Cell Activation Antigen, CD26. Science 261, 466–469. doi: 10.1126/science.8101391
Kim, K. M., Noh, J. H., Bodogai, M., Martindale, J. L., Yang, X., Indig, F. E., et al. (2017). Identification of senescent cell surface targetable protein DPP4. Genes Dev. 31, 1529–1534. doi: 10.1101/gad.302570.117
Klein, N., Gembardt, F., Supé, S., Kaestle, S. M., Nickles, H., Erfinanda, L., et al. (2013). Angiotensin-(1–7) Protects From Experimental Acute Lung Injury. Crit. Care Med. 41, 334–343. doi: 10.1097/CCM.0b013e31828a6688
Klemann, C., Wagner, L., Stephan, M., von Hörsten, S. (2016). Cut to the chase: a review of CD26/dipeptidyl peptidase-4’s (DPP4) entanglement in the immune system. Clin. Exp. Immunol. 185, 1–21. doi: 10.1111/cei.12781
Klonoff, D. C., Umpierrez, G. E. (2020). COVID-19 in patients with diabetes: risk factors that increase morbidity. Metabolims 108, 154224. doi: 10.1016/j.metabol.2020.154224
Kolahian, S., Leiss, V., Nürnberg, B. (2019). Diabetic lung disease: fact or fiction? Rev. Endocr. Metab. Disord. 20, 303–319. doi: 10.1007/s11154-019-09516-w
Kuba, K., Imai, Y., Rao, S., Gao, H., Guo, F., Guan, B., et al. (2005). A crucial role of angiotensin converting enzyme 2 (ACE2) in SARS coronavirus-induced lung injury. Nat. Med. 11, 875–879. doi: 10.1038/nm1267
Kulcsar, K. A., Coleman, C. M., Beck, S. E., Frieman, M. B. (2019). Comorbid diabetes results in immune dysregulation and enhanced disease severity following MERS-CoV infection 4. JCI Insight. 4, e131774. doi: 10.1172/jci.insight.131774
Lamers, D., Famulla, S., Wronkowitz, N., Hartwig, S., Lehr, S., Ouwens, D. M., et al. (2011). Dipeptidyl Peptidase 4 Is a Novel Adipokine Potentially Linking Obesity to the Metabolic Syndrome. Diabetes 60, 1917–1925. doi: 10.2337/db10-1707
Lang, A. L., Osterhaus, A., Haagmans, B. L. (2006). Interferon-γ and interleukin-4 downregulate expression of the SARS coronavirus receptor ACE2 in Vero E6 cells. Virology 353, 474–481. doi: 10.1016/j.virol.2006.06.011
Lee, S. A., Kim, Y. R., Yang, E. J., Kwon, E., Kim, S. H., Kang, S. H., et al. (2013). CD26/DPP4 Levels in Peripheral Blood and T Cells in Patients With Type 2 Diabetes Mellitus. J. Clin. Endocrinol. Metab. 98, 2553–2561. doi: 10.1210/jc.2012-4288
Li, W., Moore, M. J., Vasilieva, N., Sui, J., Kee Wong, S. (2003). Angiotensin-converting enzyme 2 is a functional receptor for the SARS coronavirus. Nature 426, 450–454. doi: 10.1038/nature02145
Li, X., Molina-Molina, M., Abdul-Hafez, V. U., Amal, , X. A., Uhal, B. D. (2008). Angiotensin converting enzyme-2 is protective but downregulated in human and experimental lung fibrosis. Am. J. Physiol. Lung Cell Mol. Physiol. 295, 178–185. doi: 10.1152/ajplung.00009.2008
Li, Z., Fang, J., Jiao, R., Wei, X., Ma, Y., Liu, X., et al. (2017). A novel multi-epitope vaccine based on Dipeptidyl Peptidase 4 prevents streptozotocin-induced diabetes by producing anti-DPP4 antibody and immunomodulatory effect in C57BL/6J mice. BioMed. Pharmacother. 89, 1467–1475. doi: 10.1016/j.biopha.2017.01.089
Li, B., Yang, J., Zhao, F., Zhi, L., Wang, X., Liu, L., et al. (2020). Prevalence and impact of cardiovascular metabolic diseases on COVID-19 in China. Clin. Res. Cardiol. 109, 531–538. doi: 10.1007/s00392-020-01626-9
Li, L., Huang, Q., Wang, D. C., Ingbar, D. H., Wang, X. (2020). Acute lung injury in patients with COVID-19 infection. Clin. Trans. Med. 10. doi: 10.1002/ctm2.16
Lighter, J., Phillips, M., Hochman, S., Sterling, S., Johnson, D., Francois, F., et al. (2020). Obesity in patients younger than 60 years is a risk factor for Covid-19 hospital admission. Clin. Infect. Dis. ciaa415. doi: 10.1093/cid/ciaa415
Lopes, R. D., Scarlatelli Macedo, A. V., Melo de Barros e Silva, P. D., Junqueira Moll-Bernardes, R., Feldman, A., Saba Arruda, G. D., et al. (2020). Continuing versus suspending angiotensinconvertingenzymeinhibitorsandangiotensin receptor blockers: Impact on adverse outcomes in hospitalized patients with severe acute respiratory syndrome coronavirus 2 (SARS-CoV-2)–The BRACE CORONA Trial. Eur. Heart J. Cariovasc. Pharmacother. 226, 49–59. doi: 10.1016/j.ahj.2020.05.002
Lu, R., Zhao, X., Li, J., Niu, P., Yang, B., Wu, H., et al. (2020). Genomic characterisation and epidemiology of 2019 novel coronavirus: implications for virus origins and receptor binding. Lancet 395, 565–574. doi: 10.1016/S0140-6736(20)30251-8
Maeda, S., Matsui, T., Yamagishi, S. (2012). Vildagliptin inhibits oxidative stress and vascular damage in streptozotocin-induced diabetic rats. Int. J. Cardiol. 158, 171–173. doi: 10.1016/j.ijcard.2012.04.087
Makdissi, A., Ghanim, H., Vora, M., Green, K., Abuaysheh, S., Chaudhuri, A., et al. (2012). Sitagliptin Exerts an Antinflammatory Action. J. Clin. Endocrinol. Metab. 97, 3333–3341. doi: 10.1210/jc.2012-1544
Mast, A. (2016). Tissue Factor Pathway Inhibitor: Multiple Anticoagulant Activities for a Single Protein. Atheros. Thromb. Vasc. Biol. 36, 9–14. doi: 10.1161/ATVBAHA.115.305996
Meng, J., Xiao, G., Zhang, J., He, X., Ou, M., Bi, J., et al. (2020). Renin-angiotensin system inhibitors improve the clinical outcomes of COVID-19 patients with hypertension. Emerg. Microb. Infect. 9, 757–760. doi: 10.1080/22221751.2020.1746200
Monteil, V., Kwon, H., Prado, P., Hagelkrüys, A., Wimmer, R. A., Stahl, M., et al. (2020). Inhibition of SARS-CoV-2 infections in engineered human tissues using clinical-grade soluble human ACE2. Cell 181, 905–913. doi: 10.1016/j.cell.2020.04.004
Nabeno, M., Akahoshi, F., Kishida, H., Miyaguchi, I., Tanaka, Y., Ishii, S., et al. (2013). A comparative study of the binding modes of recently launched dipeptidyl peptidase IV inhibitors in the active site. Biochem. Biophys. Res. Commun. 434, 191–196. doi: 10.1016/j.bbrc.2013.03.010
Niraula, A., Thapa, S., Kunwar, S., Lamsal, M., Baral, N., Maskey, R. (2018). Adenosine deaminase activity in type 2 diabetes mellitus: does it have any role? MBC Endo. Disord. 18, 58. doi: 10.1186/s12902-018-0284-9
Ohnuma, K., Uchiyama, M., Hatano, R., Takasawa, W., Endo, Y., Dang, N. H., et al. (2009). Blockade of CD26-mediated T cell costimulation with soluble caveolin-1-Ig fusion protein induces anergy in CD4 +T cells. Biochem. Biophys. Res. Commun. 386, 327–332. doi: 10.1016/j.bbrc.2009.06.027
Ohnuma, K., Haagmans, B. L., Hatano, R., Raj, V. S., Mou, H., Iwata, S., et al. (2013). Inhibition of Middle East respiratory syndrome coronavirus infection by anti-CD26 monoclonal antibody. J. Virol. 87, 13892. doi: 10.1128/JVI.02448-13
Oxley, T. J., Mocco, J., Majidi, S., Kellner, C. P., Shoirah, H., Singh, I. P., et al. (2020). Large-Vessel Stroke as a Presenting Feature of Covid-19 in the Young. NEJM 38220, e60. doi: 10.1056/NEJMc2009787
Pang, Z., Nakagami, H., Osako, M. K., Koriyama, H., Nakagami, F., Tomioka, H., et al. (2014). Therapeutic vaccine against DPP4 improves glucose metabolism in mice. Proceed Int. Acad. Sci. 111, E1256. doi: 10.1073/pnas.1322009111
Patterson, B. K., Seethamraju, H., Dhody, K., Corley, M. J., Kazempour, K., Lalezari, J., et al. (2020). Disruption of the CCL5/RANTES-CCR5 Pathway 1 Restores Immune 2 Homeostasis and Reduces Plasma Viral Load in Critical COVID-19. Preprint 2020.05.02.20084673. doi: 10.1101/2020.05.02.20084673
Peiró, C., Moncada, S. (2020). Substituting Angiotensin-(1-7) to Prevent Lung Damage in SARS- CoV2 Infection? Circulation 141, 1665–1666. doi: 10.1161/CIRCULATIONAHA.120.047297
Petrilli, C., Jones, S. A., Yang, J. Y., Rajagopalan, H., O’Donnell, K., Chernyak, Y., et al. (2020). Factors associated with hospitalization and critical illness among 4,103 patients with Covid-19 disease in New York City. BMJ 369, m1966. doi: 10.1101/2020.04.08.20057794.this
Picatoste, B., Ramírez, E., Caro-Vadillo, A., Iborra, C., Egido, J., Tuñón, J., et al. (2013). Sitagliptin Reduces Cardiac Apoptosis, Hypertrophy and Fibrosis Primarily by Insulin-Dependent Mechanisms in Experimental type-II Diabetes. Potential Roles of GLP-1 Isoforms. PloS One 8, e78330. doi: 10.1371/journal.pone.0078330
Pitocco, D., Zaccardi, F., Martini, F., Scavone, G., Musella, T., Caputo, S., et al. (2010). Severe leucopenia associated with Sitagliptin use. Dibetes Res. Clin. Pract. 91, e30–e32. doi: 10.1016/j.diabres.2010.10.004
Pitocco, D., Tartaglione, L., Viti, L., Di Leo, M., Pontecorvi, A., Caputo, S. (2020). SARS-CoV-2 and DPP4 inhibition: Is it time to pray for Janus Bifrons? Dibetes Res. Clin. Pract. 163, 108162. doi: 10.1016/j.diabres.2020.108162
Qi, F., Quian, S., Zhang, S., Zhang, Z. (2020). Single cell RNA sequencing of 13 human tissues identify cell types and receptors of human coronaviruses. Biochem. Biophys. Res. Commun. 526, 135–140. doi: 10.1016/j.bbrc.2020.03.044
Raj, V. S., Mou, H., Smits, S. L., Dekkers, D. H. W., Müller, M. A., Dijkman, R., et al. (2013). Dipeptidyl peptidase 4 is a functional receptor for the emerging human coronavirus. Nature 495, 251–254. doi: 10.1038/nature12005
Röhrborn, D., Eckel, J., Sell, H. (2014). Shedding of dipeptidyl peptidase 4 is mediated by metalloproteases and up-regulated by hypoxia in human adipocytes and smooth muscle cells. FEBS Lett. 588, 3870–3877. doi: 10.1016/j.febslet.2014.08.029
Röhrborn, D., Wronkowitz, N., Eckel, J. (2015). DPP4 in diabetes. Front. Immunol. 6, 386. doi: 10.3389/fimmu.2015.00386
Romacho, T., Vallejo, S., Villalobos, L., Wronkowitz, N., Indrakusuma, I., Sell, H., et al. (2016). Soluble dipeptidyl peptidase-4 induces microvascular endothelial dysfunction through proteinase-activated receptor-2 and thromboxane A2 release. Hypertension 34, 869–876. doi: 10.1097/HJH.0000000000000886
Romacho, T., Sell, H., Indrakusuma, I., Roehrborn, D., Castañeda, T. R., Jelenik, T., et al. (2020). DPP4 deletion in adipose tissue improves hepatic insulin sensitivity in diet-induced obesity. Am. J. Physiol. Endocrinol. Metab. 318, E590–E599. doi: 10.1152/ajpendo.00323.2019
Romero, A., San Hipólito-Luengo, Á, Villalobos, L. A., Vallejo, S., Valencia, I., Michalska, P., et al. (2019). The angiotensin-(1-7)/Mas receptor axis protects from endothelial cell senescence via klotho and Nrf2 activation. Anging Cell. 18, e12913. doi: 10.1111/acel.12913
Santos, R. A. S., Sampaio, W. O., Alzamora, A. C., Motta-Santos, D., Alenina, N., Bader, M., et al. (2018). The ACE2/Angiotensin-(1–7)/MAS Axis of the Renin-Angiotensin System: Focus on Angiotensin-(1–7). Physiol. Rev. 98, 505–553. doi: 10.1152/physrev.00023.2016
Scheen, A. (2018). Cardiovascular Effects of New Oral Glucose-Lowering Agents: DPP-4 and SGLT-2 Inhibitors. Circ. Res. 122, 1439–1459. doi: 10.1161/CIRCRESAHA.117.311588
Segers, V. F. M., Revin, V., Wu, W., Qiu, H., Yan, Z., Lee, R. T., et al. (2011). Protease-Resistant Stromal Cell–Derived Factor-1 for the Treatment of Experimental Peripheral Artery Disease. Circulation 123, 1306–1315. doi: 10.1161/CIRCULATIONAHA.110.991786
Sell, H., Bl̈her, M., Klöting, N., Schlich, R., Willems, M., Ruppe, F., et al. (2013). Adipose Dipeptidyl Peptidase-4 and Obesity. Diabetes Care 36, 4083–4090. doi: 10.2337/dc13-0496
Sha, Z., Kampfrath, T., Deiuliis, J. A., Zhong, J., Pineda, C., Ying, Z., et al. (2014). Chronic DPP4-4 inhibition reduced atherosclerosis and inflammation via effects on monocyte recruitment and chemotaxis. Circulation 124, 2338–2349. doi: 10.1161/CIRCULATIONAHA.111.041418
Shao, S., Xu, Q. Q., Yu, X., Pan, R., Chen, Y. (2020). Dipeptidyl peptidase 4 inhibitors and their potential immune modulatory functions. Pharmacol. Ther. 107503. doi: 10.1016/j.pharmthera.2020.107503
Shieh, W. J., Hsiao, C. H., Paddock, C., Guarner, J., Golsmith, C. S., Tatti, K., et al. (2005). Immunohistochemical, in situ hybridization, and ultrastructural localization of SARS-associated coronavirus in lung of a fatal case of severe acute respiratory syndrome in Taiwan. Hum. Pathol. 36, 303–309. doi: 10.1016/j.humpath.2004.11.006
Simões e Silva, A., Silveira, K., Ferreira, A., Teixeira, M. (2013). ACE2, angiotensin-(1-7) and Mas receptor axis in inflammation and fibrosis B. J. Pharmacol. 169, 477–492. doi: 10.1111/bph.12159
Simonnet, A., Chetboun, M., Poissy, J., Raverdy, V., Noulette, J., Duhamel, A., et al. (2020). High prevalence of obesity in severe acute respiratory syndrome coronavirus-2 (SARS-CoV-2) requiring invasive mechanical ventilation. B. J. Pharmacol. 28, 1195–1199. doi: 10.1002/oby.22831
Srivastava, P., Badhwar, S., Chandran, D. S., Jaryal, A. K., Jyotsna, V. P., Deepak, K. K. (2019). Imbalance between Angiotensin II - Angiotensin (1-7) system is associated with vascular endothelial dysfunction and inflammation in type 2 diabetes with newly diagnosed hypertension. Clin. Res. Rev. 13, 2061–2068. doi: 10.1016/j.dsx.2019.04.042
Sukumaran, V., Veeraveedu, P. T., Gurusamy, N., Yamaguchi, K., Lakshmanan, A. P., Ma, M., et al. (2011). Cardioprotective Effects of Telmisartan against Heart Failure in Rats Induced By Experimental Autoimmune Myocarditis through the Modulation of Angiotensin-Converting Enzyme-2/Angiotensin 1-7/Mas Receptor Axis. Int. J. Biol. Sci. 7, 1077–1092. doi: 10.7150/ijbs.7.1077
Tang, N., Li, D., Wang, X., Sun, Z. (2020). Abnormal coagulation parameters are associated with poor prognosis in patients with novel coronavirus pneumonia. J. Thrombos Heamost. 18, 844–847. doi: 10.1111/jth.14768
Thomas, M., Pickering, R., Tsorotes, D., Koitka, A., Sheehy, K., Bernardi, S., et al. (2010). Genetic Ace2 Deficiency Accentuates Vascular Inflammation and Atherosclerosis in the ApoE Knockout Mouse. Circ. Res. 107, 888–897. doi: 10.1161/CIRCRESAHA.110.219279
Tremblay, A. J., Lamarche, B., Deacon, C. F., Weisnagel, S. J., Couture, P. (2014). Effects of sitagliptin therapy on markers of low-grade inflammation and cell adhesion molecules in patients with type 2 diabetes. Metabolism 63, 1141–1148. doi: 10.1016/j.metabol.2014.06.004
Turner, A. J., Tipnis, S. R., Guy, J. L., Rice, G. H., Nigel, M. (2002). ACEH/ACE2 Is a Novel Mammalian Metallocarboxypeptidase and a Homologue of Angiotensin-Converting Enzyme Insensitive to ACE Inhibitors. Can. J. Physiol. Pharmacol. 80, 346–353. doi: 10.1139/y02-021
Vaduganathan, M., Vardeny, O., Michel, T., McMurray, J. J. V., Pfeffer, M. A., Solomon, S. D. (2020). Renin–Angiotensin–Aldosterone System Inhibitors in Patients with Covid-19. NEJM 382, 1653–1659. doi: 10.1056/NEJMsr2005760
van de Garde, E. M. W. A., Souverein, P. C., Hak, E., Deneer, V. H., van den Bosch, J. M. M., Leufkens, H. G. (2007). Angiotensin-converting enzyme inhibitor use and protection against pneumonia in patients with diabetes. Hypertension 25, 235–239. doi: 10.1097/HJH.0b013e328010520a
Vankadari, N., Wilce, J. A. (2020). Emerging COVID-19 coronavirus: glycan shield and structure prediction of spike glycoprotein and its interaction with human CD26. Emerg. Microb. Infect. 9, 601–604. doi: 10.1080/22221751.2020.1739565
Varga, Z., Flammer, A. J., Steiger, P., Haberecker, M., Andermatt, R., Zinkernagel, A. S., et al. (2020). Endothelial cell infection and endotheliitis in COVID-19. Lancet 395, 1417–1418. doi: 10.1016/S0140-6736(20)30937-5
Villalobos, L. A., San Hipólito-Luengo, Á, Ramos-González, M., Cercas, E., Vallejo, S., Romero, A., et al. (2016). The Angiotensin-(1-7)/Mas Axis Counteracts Angiotensin II-Dependent and -Independent Pro-inflammatory Signaling in Human Vascular Smooth Muscle Cells. Front. Pharmacol. 7, 482. doi: 10.3389/fphar.2016.00482
Villar, J., Zhang, H., Slutsky, A. S. (2019). Lung Repair and Regeneration in ARDS. Chest 155, 587–594. doi: 10.1016/j.chest.2018.10.022
Vora, K. A., Porter, G., Peng, R., Cui, Y., Pryor, K., Eiermann, G., et al. (2009). Genetic ablation or pharmacological blockade of dipeptidyl peptidase IV does not impact T cell-dependent immune responses. BMC Immunol. 10, 19. doi: 10.1186/1471-2172-10-19
Wang, F., He, Y., Zhang, R., Zeng, Q., Zhao, X. (2017). Combination therapy of metformin plus dipeptidyl peptidase-4 inhibitor versus metformin plus sulfonylurea and their association with a decreased risk of cardiovascular disease in type 2 diabetes mellitus patients. Medicine 96, e7638. doi: 10.1097/MD.0000000000007638
Wang, B., Li, R., Lu, Z., Huang, Y. (2020). Does comorbidity increase the risk of patients with COVID-19: evidence from meta-analysis. Aging 12, 6049–6057. doi: 10.18632/aging.103000
Wang, D., Hu, B., Hu, C., Zhu, F., Liu, X., Zhang, J., et al. (2020). Clinical Characteristics of 138 Hospitalized Patients With 2019 Novel Coronavirus–Infected Pneumonia in Wuhan, China. JAMA 323, 1061–1069. doi: 10.1001/jama.2020.1585
Wang, J., Hajizadeh, N., Moore, E. E., McIntyre, R. C., Moore, P. K., Veress, L. A., et al. (2020). Tissue plasminogen activator (tPA) treatment for COVID-19 associated acute respiratory distress syndrome (ARDS): A case series. J. Thromb. Haemost. 18, 1752–1755. doi: 10.1111/jth.14828
Wang, K., Chen, W., Zhou, Y. S., Lian, J. Q., Zhang, Z., Du, P., et al. (2020). SARS-CoV-2 invades host cells via a novel route: CD147-spike protein. Preprint 2020.03.14.988345. doi: 10.1101/2020.03.14.988345
Williams, K., Vieira De Ribeiro, A. J., Prakoso, E., Veillard, A., Shackel, N. A., Brooks, B., et al. (2015). Circulating dipeptidyl peptidase-4 activity correlates with measures of hepatocyte apoptosis and fibrosis in non-alcoholic fatty liver disease in type 2 diabetes mellitus and obesity: A dual cohort cross-sectional study. Diabetes 7, 809–819. doi: 10.1111/1753-0407.12237
Wronkowitz, N., Görgens, S. W., Romacho, T., Villalobos, L. A., Sánchez-Ferrer, C. F., Peiró, C., et al. (2014). Soluble DPP4 induces inflammation and proliferation of human smooth muscle cells via protease-activated receptor 2. Biochem. Biophys. Acta 1842, 1613. doi: 10.1016/j.bbadis.2014.06.004
Wronkowitz, N., Romacho, T., Sell, H., Eckel, J. (2015). Adipose Tissue Dysfunction and Inflammation in Cardiovascular Disease. Front. Horm. Res. 43, 79–92. doi: 10.1159/000360560
Wu, Z., McGoogan, J. M. (2020). Characteristics of and Important Lessons From the Coronavirus Disease 2019 (COVID-19) Outbreak in China: Summary of a Report of 72 314 Cases From the Chinese Center for Disease Control and Prevention. JAMA 323, 1239. doi: 10.1001/jama.2020.2648
Xia, S., Liu, Q., Wang, Q., Sun, Z., Su, S., Du, L., et al. (2014). Middle East respiratory syndrome coronavirus (MERS-CoV) entry inhibitors targeting spike protein. Virus Res. 194, 200–210. doi: 10.1016/j.virusres.2014.10.007
Xu, Z., Shi, L., Wang, Y., Zhang, J., Huang, L., Zhang, C., et al. (2020). Pathological findings of COVID-19 associated with acute respiratory distress syndrome. Lancet Resp. Med. 8, 420–422. doi: 10.1016/S2213-2600(20)30076-X
Yang, W., Cai, X., Han, X., Ji, L. (2016). DPP-4 inhibitors and risk of infections: a meta-analysis of randomized controlled trials. Diabetes Metab. Res. Rev. 32, 391–404. doi: 10.1002/dmrr.2723
Yang, C., Yang, J., Zhang, J. (2020). More clinical warning indicators should be explored for monitoring COVID-19 patients’ condition. Int. J. Cardiol. 310. doi: 10.1016/j.ijcard.2020.04.010
Yang, G., Tan, Z., Zhou, L., Yang, M., Peng, L., Liu, J., et al. (2020). Angiotensin II Receptor Blockers and Angiotensin-Converting Enzyme Inhibitors Usage is Associated with Improved Inflammatory Status and Clinical Outcomes in COVID-19 Patients With Hypertension. Can. Med. A. J. 183, E309–E311. doi: 10.1503/cmaj.110366
Zhang, D., Xie, J., Yan, X., Zhou, X., Liu, Z., Wang, J., et al. (2020). Coagulopathy and Antiphospholipid Antibodies in Patients with Covid-19. B. J. Surg. 106, 949. doi: 10.1002/bjs.11197
Zhang, H., Penninger, J. M., Li, Y., Zhong, N., Slutsky, A. S. (2020). Angiotensin-converting enzyme 2 (ACE2) as a SARS-CoV-2 receptor: molecular mechanisms and potential therapeutic target. Inten Care Med. 46, 586–590. doi: 10.1007/s00134-020-05985-9
Zhong, J., Rao, X., Deiuliis, J., Braunstein, Z., Narula, V., Hazey, J., et al. (2013). A Potential Role for Dendritic Cell/Macrophage-Expressing DPP4 in Obesity-Induced Visceral Inflammation. Diabetes 62, 149–157. doi: 10.2337/db12-0230
Zhong, J., Maiseyeu, A., Davis, S. N., Rajagopalan, S. (2015). DPP4 in cardiometabolic disease: recent insights from the laboratory and clinical trials of DPP4 inhibition. Circ. Res. 116, 1491–1504. doi: 10.1161/CIRCRESAHA.116.305665
Zhou, F., Yu, T., Du, R., Fan, G., Liu, Y., Liu, Z., et al. (2020). Clinical course and risk factors for mortality of adult inpatients with COVID-19 in Wuhan, China: a retrospective cohort study. Lancet 395, 1054–1062. doi: 10.1016/S0140-6736(20)30566-3
Keywords: COVID-19, SARS-CoV-2, diabetes, cardiovascular disease, angiotensin converting enzyme 2, dipeptidyl peptidase 4, gliptins, ACEi
Citation: Valencia I, Peiró C, Lorenzo Ó, Sánchez-Ferrer CF, Eckel J and Romacho T (2020) DPP4 and ACE2 in Diabetes and COVID-19: Therapeutic Targets for Cardiovascular Complications? Front. Pharmacol. 11:1161. doi: 10.3389/fphar.2020.01161
Received: 05 June 2020; Accepted: 17 July 2020;
Published: 07 August 2020.
Edited by:
Dieter Steinhilber, Goethe University Frankfurt, GermanyReviewed by:
Lu Cai, University of Louisville, United StatesKristen Bubb, Monash University, Australia
Chris R. Triggle, Weill Cornell Medicine, Qatar
Copyright © 2020 Valencia, Peiró, Lorenzo, Sánchez-Ferrer, Eckel and Romacho. This is an open-access article distributed under the terms of the Creative Commons Attribution License (CC BY). The use, distribution or reproduction in other forums is permitted, provided the original author(s) and the copyright owner(s) are credited and that the original publication in this journal is cited, in accordance with accepted academic practice. No use, distribution or reproduction is permitted which does not comply with these terms.
*Correspondence: Tania Romacho, tania.romacho@ddz.de