- School of Health and Wellbeing, University of Southern Queensland, Toowoomba, QLD, Australia
Obesity is a global epidemic, placing socioeconomic strain on public healthcare systems, especially within the so-called Western countries, such as Australia, United States, United Kingdom, and Canada. Obesity results from an imbalance between energy intake and energy expenditure, where energy intake exceeds expenditure. Current non-invasive treatments lack efficacy in combating obesity, suggesting that obesity is a multi-faceted and more complex disease than previously thought. This has led to an increase in research exploring energy homeostasis and the discovery of a complex bidirectional communication axis referred to as the gut-brain axis. The gut-brain axis is comprised of various neurohumoral components that allow the gut and brain to communicate with each other. Communication occurs within the axis via local, paracrine and/or endocrine mechanisms involving a variety of gut-derived peptides produced from enteroendocrine cells (EECs), including glucagon-like peptide 1 (GLP1), cholecystokinin (CCK), peptide YY3−36 (PYY), pancreatic polypeptide (PP), and oxyntomodulin. Neural networks, such as the enteric nervous system (ENS) and vagus nerve also convey information within the gut-brain axis. Emerging evidence suggests the human gut microbiota, a complex ecosystem residing in the gastrointestinal tract (GIT), may influence weight-gain through several inter-dependent pathways including energy harvesting, short-chain fatty-acids (SCFA) signalling, behaviour modifications, controlling satiety and modulating inflammatory responses within the host. Hence, the gut-brain axis, the microbiota and the link between these elements and the role each plays in either promoting or regulating energy and thereby contributing to obesity will be explored in this review.
Obesity: An Increasing Problem
Obesity is one of the most rapidly escalating epidemics faced by global public-health systems, in particular, those belonging to developed Westernised societies, such as Australia, United States, United Kingdom, and Canada. In the 1970s, overweight and obesity were uncommon with less than 15% of Australians being described in this category (Hayes et al., 2017). By 1995, the rate of overweight and obesity had increased to approximately 20% (Tolhurst et al., 2016; Hayes et al., 2017). Australia now possesses one of the highest incidence of overweight and obesity worldwide, affecting 63.4% of adults and 29.5% of people aged less than seventeen (Grima and Dixon, 2013; Tolhurst et al., 2016). Additionally, 44.5% of adults and between 70.1 and 91.7% of people 17 or under do not meet the minimum daily physical activity requirements and approximately 40% of the nation acquire their daily energy intake from “junk” food, which is described as a Westernised-diet high in both saturated and trans fats and simple carbohydrates and, therefore, hyper-caloric (Tolhurst et al., 2016). Obesity occurs when there is increased fat deposition following an imbalance between energy consumption and expenditure, where consumption exceeds expenditure. Extending from this simplistic definition, obesity is a consequence of multifaceted interactions among genetic, environmental, socio-economic, psychological, and dietary factors, thus making obesity a complex disease to understand and combat (Moran and Shanahan, 2014; Bauer et al., 2016).
Obesity is characterised by the presence of parameters indicating increased adiposity, low-grade inflammation, dysbiosis, increased neurogenic tone and hormonal imbalances (Buhmann et al., 2014; Moran and Shanahan, 2014; Bauer et al., 2016). These obesogenic factors give rise to comorbidities (Table 1), which in turn increase morbidity and mortality. Therefore, obesity determinants, as well as the associated costs, which are in excess of $8 billion per year in Australia alone, and unsuccessful non-invasive treatment interventions have resulted in an increase in research aimed at improving weight-loss approaches (Grima and Dixon, 2013; Buhmann et al., 2014). Currently, bariatric surgeries such as Roux-en-Y gastric bypass, laparoscopic sleeve gastrectomy and laparoscopic adjustable gastric banding, are the most effective treatments in increasing and sustaining long-term weight loss. However, it is relatively unknown why bariatric surgeries are successful. It is suggested that changes in the systemic and local concentrations of gut-derived peptides and the altered responses that are subsequently generated at the sites of action, in addition to changes in vagal firing and, therefore, signalling to the brain may be the key to understanding the success of bariatric surgeries (Santo et al., 2016; Yavuz et al., 2017). Consequently, a large degree of knowledge regarding the interplay between the central nervous system (CNS) and the gastrointestinal tract (GIT), and more recently the gut microbiota, with regard to energy homeostasis has been generated. Hence this review will focus on exploring the link between the gut-brain-microbiota axis and the role each aspect of this axis plays in either promoting or regulating energy, thus contributing to the obesogenic state.
The CNS, in particular, the brain, has the elaborate task of interpreting continuous information provided to it by neural networks and chemical messengers with respect to the body's energy state. It uses this information to initiate an appropriate reaction to maintain homeostasis. These signals vary throughout time and the responses alter depending on what type of food has been ingested. Although foodstuffs are first encountered by the oral microbiome, the GIT remains one of the primary sites where they are first sampled. Therefore, the gut becomes responsible for generating the majority of inputs communicated to the CNS regarding the content and size of a meal, thus establishing a complex bi-directional communication system, referred to as the gut-brain axis (Bauer et al., 2016; Gribble and Reimann, 2016).
The Gut-Brain Axis: Connections from the Gut to the Brain
The gut-brain axis is a complex neurohumoral communication network imperative for maintaining metabolic homeostasis. It is comprised of the CNS, enteric nervous system (ENS), the autonomic nervous system (ANS) and its associated sympathetic and parasympathetic branches, neuroendocrine and immunological systems, in addition to the gut microbiota, which will be discussed below (Grenham et al., 2011). Axis communication is formed through sensory information being converted into neural, hormonal and immunological signals, which are relayed back and forth from the CNS to the gut and vice versa (Mayer et al., 2015). Whilst there is increasing evidence that changes in intestinal immune-signalling convey shifts in gut-facilitated energy homeostasis, the majority of recognised axial effects on energy homeostasis are a consequence of neural and hormonal gut-derived signals, as the GIT possesses over 500 million neurons and is capable of producing an array of hormones (Monje, 2017). Hence, due to the large degree of innervation supplying the GIT, preabsorptive foodstuffs can initiate signals to the CNS regarding macronutrient content and caloric value through individualised nutrient-specific sensory mechanisms located throughout the GIT (Hamr et al., 2015). These signals are subsequently conveyed to various regions of the brain, such as the brainstem and hypothalamus. The higher-order processing of these centres consequently initiates a series of reactions that result in both acute and chronic deviations in energy consumption and expenditure, thus maintaining metabolic homeostasis pre- and post-prandial (Buhmann et al., 2014).
Gut hormones are released by enteroendocrine cells (EECs), which initiate the majority of signalling and communication within the gut-brain axis in response to preabsorptive nutrients. These cells are located throughout the epithelium of the GIT, with many containing an apical cell membrane covered in microvilli, which open to and directly contact the luminal contents (Gribble and Reimann, 2016). An overview of EEC function is provided below (Figure 1).
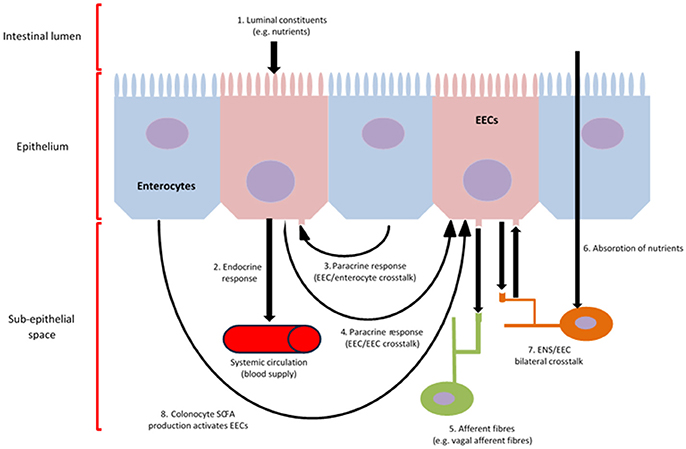
Figure 1. EEC function and communication. Intracellular metabolism and activation of chemoreceptors located on the apical cell membrane of EECs, result in calcium influx, which induces the synthesis and release of gut hormones into the sub-epithelial space (1, 4) (Psichas et al., 2015). Various gut-derived hormones are synthesised and secreted in response to luminal constituents and released from EECs systemically to induce an effect on various tissues throughout the body, such as the brain, via, metabolic, local, paracrine (3) and/or endocrine (2) action, as well as the activation of afferent neurons innervating the GIT wall (5, 6, 7, 8) (Psichas et al., 2015). Further, EEC/ENS crosstalk can result from the direct absorption of nutrients through the intestine (7). The production of SCFA by the microbiome, which can be subsequently utilised by colonocytes as an energy source, can activate EECs, thus contributing to gut-brain activation (8).
The bulk of digestion and nutrient absorption occurs within the stomach and small intestine. Therefore, these organs are highly innervated as they are the primary sites responsible for nutrient-sensing. This dense area of innervation originates from the vagal and splanchnic nerves (Bauer et al., 2016). Here the quantity of afferent fibres outnumbers the quantity of efferent fibres, indicating a fundamental role of neuronal gut-to-brain signalling (Prechtl and Powley, 1990; Berthoud et al., 1995). Vagal fibres in particular, extend into the lamina propria of the intestinal villi, terminate at the basolateral cell membrane of EECs and express receptors for gut hormones such as ghrelin, leptin, cholecystokinin (CCK), glucagon-like peptide 1 (GLP1), and peptide YY3−36 (PYY), thus leading to receptor activation and subsequent neuronal stimulation (Dockray, 2013). Additionally, ENS neurons, which possess receptors for various gut hormones, may indirectly activate vagal and spinal afferents (Amato et al., 2010; Richards et al., 2014). Whilst the ENS controls intestinal function locally via reflex actions, it cannot be dismissed from playing a role in transmitting nutrient-derived signals to vagal afferents, thus contributing to the gut-brain axis (Costa et al., 2000; Sayegh et al., 2004). Intrinsic ENS neurons are proximally located to both EECs and various afferent nerve terminals; stimulated by intestinal nutrient infusion; and stimulate vagal afferent fibres in the gut (Sayegh et al., 2004; Ritter, 2011). Whilst the exact mechanisms have not been completely elucidated and the notion that the ENS can function independently of CNS involvement is still favoured, it is clear from these studies that the gut-brain neuronal-signalling axis is initiated by nutrient-induced gut hormone secretion.
Upon food consumption, sensory information is conveyed from the gastrointestinal vagal and/or somatosensory (spinal) afferent fibres to the nucleus tractus solitarius (NTS). More specifically, vagal afferents converge in the NTS of the dorsal vagal complex within the brainstem, and somatosensory afferents synapse with neurons in the posteromarginal nucleus of the spinal dorsal horn, which then project to the NTS (Zittel et al., 1994; Schwartz et al., 2000). The NTS, in turn, integrates and carries these gut-derived signals to the hypothalamus (Craig, 1996; Schwartz et al., 2000). Using c-Fos—a marker used to represent increased neuronal activity—Zittel et al. (1994) demonstrated that its expression in the NTS increased upon nutrient infusion within the gut, whilst high-dose capsaicin treatment, which acts as a neurotoxin, decreased c-Fos expression and blocked gut-brain vagal communication (Mönnikes et al., 1997). Additionally, Campos et al. (2013) reported that NTS neurons were stimulated by vagal afferents by the activation of n-methyl-D-aspartate (NMDA) receptors in afferent terminals, which subsequently led to neurotransmitter release via phosphorylation of extracellular signal-related kinases 1/2 and synapsin 1. Babic et al. (2009) established that other NTS neurons are activated via vagal afferents stimulating pro-opiomelanocortin (POMC) and catecholaminergic neurons. These NTS neurons have been linked to contributing to satiety via signalling melanocortin receptors within the hypothalamus (Figure 2). Interestingly, a deficiency in melanocortin-receptor 4 has been demonstrated to contribute to obesity (Farooqi et al., 2003). Whilst, more studies in this area are needed to confirm the exact mechanisms as to how these different receptors and neurons interact, it may lead to a potential and more advanced understanding of how NTS subset populations contribute to energy homeostasis. Furthermore, the findings implicating POMC and catecholaminergic neuron stimulation via a vagal pathway, as well the presence of NMDA receptors within the NTS, may assist in understanding the pathways that link food consumption with behavior modifications, given that these neurons release neurotransmitters, such as dopamine, which are linked to reward, arousal, motivation and emotion.
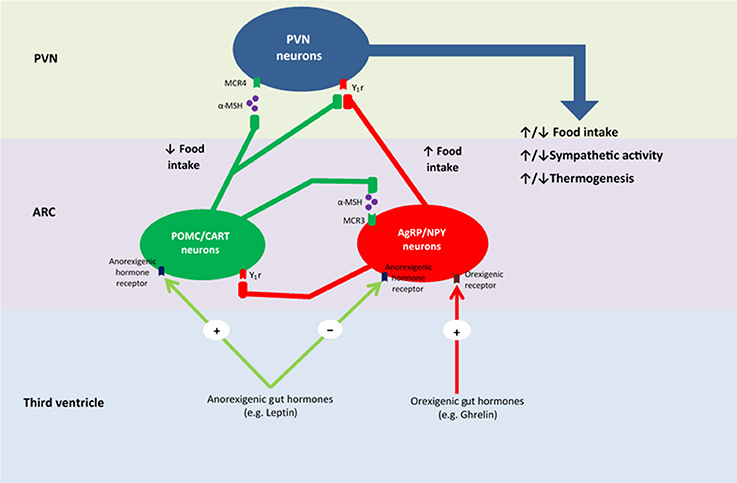
Figure 2. Proposed mechanism of energy homeostasis within the hypothalamus. PVN, paraventricular nucleus; ARC, arcuate nucleus; MCR4, melanocortin 4 receptor; α-MSH, α- melanocortin-stimulating hormone; MCR3, melanocortin 3 receptor; Y1r, neuropeptide Y receptor type 1; POMC, pro-opiomelanocortin; CART, cocaine- and amphetamine-regulated transcript; NPY, neuropeptide Y; AgRP, agouti-related protein.
NTS neurons project and terminate at several higher-order centres of the brain, including the melanocortin system incorporating the hypothalamus (Suzuki et al., 2012). The hypothalamus performs the fundamental role of integrating peripheral humoral signals that transduce information regarding nutrient consumption and energy expenditure, as well information relayed from the NTS and other superior regions of the brain (Bauer et al., 2016). Of particular importance with respect to feeding behaviour and energy homeostasis are the arcuate (ARC), paraventricular, ventromedial and dorsomedial nuclei, as well as the lateral hypothalamic area (Cone et al., 2001; Suzuki et al., 2012). These hypothalamic areas are unified by circuits that regulate energy homeostasis. However, the majority of studies have focused on the ARC and its role in relation to energy homeostasis (Suzuki et al., 2012; Buhmann et al., 2014). Hence, further studies are required to explore the exact role that the residual hypothalamic regions convey in relation to feeding behaviour and energy homeostasis. Nonetheless, it is clear that nutrient-sensing occurs in the gut and triggers an array of neural and/or humoral pathways that contribute to the bi-directional communication system referred to as the gut-brain axis, which subsequently regulates energy balance.
The ARC responds to peripheral and central appetite signals via tightly-regulated neurotransmitter release from two separate neuronal populations, POMC and agouti-related protein (AgRP) neurons. AgRP neurons, located in the medial ARC, release the inhibitory neurotransmitters AgRP and neuropeptide Y (NPY) (Cone et al., 2001; Suzuki et al., 2012). These neurotransmitters act to stimulate hunger and appetite, as well as decrease energy expenditure, thus contributing to excessive food consumption and weight-gain (Dryden et al., 1995; Ollmann et al., 1997; Enriori et al., 2007). POMC neurons in the lateral ARC release POMC, which stimulates the release of α- melanocortin-stimulating hormone (α-MSH) and cocaine-and-amphetamine-regulated transcript (Suzuki et al., 2012). These neurotransmitters are antagonistic of AgRP and NPY and act by decreasing appetite and hunger, thus inhibiting food intake, as well as increasing energy expenditure, thus contributing to weight-loss (Cowley et al., 2001; Nakhate et al., 2011). Therefore, energy homeostasis involves a delicate balance between these two neuronal populations.
Adding to the complexity of hypothalamic function is that gut hormones, which increase in concentration post-prandial, have direct access to the ARC (van der Kooy, 1984). The ARC and the NTS are proximally located in an area of the brain that possesses an incomplete barrier, thus contributing to a leaky blood-brain-barrier (Bauer et al., 2016). This area is referred to as the area postrema (AP). Lesioning the AP and vagotomy diminish the effects of multiple gut hormones, thus indicating that gut hormones directly influence these brain regions once released systemically (van der Kooy, 1984; Date et al., 2002). Batterham et al. (2002) demonstrated an example of this by peripheral injection of PYY into the general circulation. PYY binds to Y2 receptor, which Batterham et al. (2002) localised to the ARC by demonstrating an increase in c-FOS immunoreactivity. Additionally, Seeley et al. (1994) established that chronic decerebrate rats, who only had the brainstem intact, had suppressed food intake and increased energy expenditure in response to intestinal nutrient infusion. This early 1990s study provides support for latter findings that the NTS may receive gut-derived signals systemically due to a leaky blood-brain-barrier (Bauer et al., 2016). It is also interesting that these rats had increased energy expenditure, given their decerebrate state (Seeley et al., 1994). This may implicate the brainstem in regulating energy expenditure through its role as a motor output cortex. More elaborate studies combining motor output with intestinal sampling are warranted. Additionally, this may assist in providing a link to dietary intake and exercise and the role that each of these factors play in relation to energy homeostasis.
Gut Hormones and the Role of the Gut-Brain Axis in Energy Homeostasis
Whilst neural connection has been explored, it is also imperative to mention the stomach's role in nutrient intake. The stomach is one of the first organs to generate a feedback signal to the melanocortin system. When food enters the GIT, as a bolus, the stomach becomes stretched, triggering a feedback-loop to the brain to cease eating. The gastric emptying of foodstuffs into the duodenum occurs once the pyloric sphincter is summoned to relax. Once the nutrients enter the duodenum, the rate of emptying decreases, thus augmenting gastric distension and limiting the amount of food consumed.
The rate of gastric emptying is decreased by vagal activation and the release of gut hormones, such as CCK, PYY, and GLP1 (Cooke and Clark, 1976; Talsania et al., 2005; Suzuki et al., 2012). This negative feedback signal was demonstrated by Davis and Smith (1990), who established that food intake diminishes within 6 min of feeding in fasting re-fed rats, thus preventing excessive food consumption. Phillips and Powley (Phillips and Powley, 1996) demonstrated in a rat model that stomach distension induces a feedback signal to cease excessive ingestion in less than 3 min rather than the content of the food by occluding the pyloric sphincter to prevent gastric emptying and by using saline in lieu of foodstuffs. These studies indicate that neurons supplying the stomach express mechanoreceptors, which are activated by stomach distension (stretch), contribute to relaying a limited information to the brain and provide limited assistance in nutrient-sensing and long-term energy homeostasis (Bauer et al., 2016). Emerging evidence reveals that taste receptors are found in the stomach and may contradict previous studies with regard to the type of sensory information relayed to the CNS and how the CNS integrates this information and conveys it to the rest of the gut (Young et al., 2009; Depoortere, 2014). In contrast, historical studies demonstrating that sham feeding—a process where foodstuffs that enter the stomach do not reach the small intestine and are bypassed directly to the colon or removed directly from the stomach—is inhibited through intestinal nutrient infusion, thus demonstrating that nutrients in the intestine can suppress food intake irrespective of gastric emptying and relay information to the gut-brain axis via neurohormoral mechanisms (Gibbs et al., 1981; Reidelberger et al., 1983). Hence, the role of gut hormones in relation to the control of food intake with regard to energy homeostasis will be explored. Since the effects of glucagon, insulin, leptin and ghrelin are extensive and well documented elsewhere, they will not be revised in this review and their functions are summarised in Table 2 (Sakata and Sakai, 2010; Dimitriadis et al., 2011; Jones et al., 2012; Pan et al., 2014).
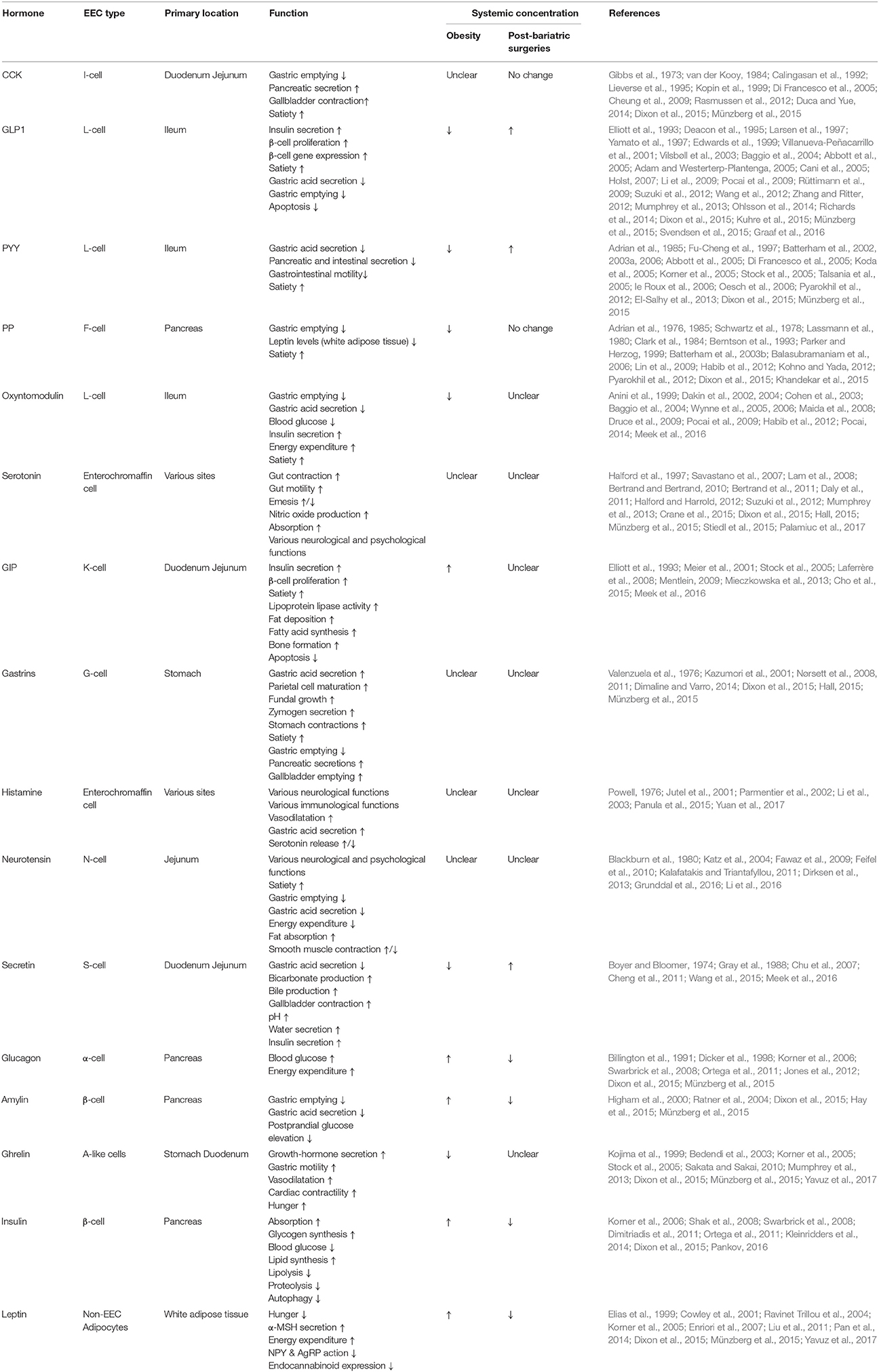
Table 2. The sites of production, functions and systemic serum concentrations of different gut hormones during obesity and post-bariatric surgeries.
Cholecystokinin
CCK was the initial gut hormone to be implicated in appetite control and was shown to be secreted post-prandial from EECs within the duodenum and jejunum (Gibbs et al., 1973). Its release is stimulated by fat and protein ingestion and its concentration augments within 15 min post-prandial (Lieverse et al., 1995; Buhmann et al., 2014). CCK possesses a short half-life of few minutes and consequently has limited time to induce its effects by acting upon CCK-1 and CCK-2 receptors located throughout tissues of GIT and the CNS, including the vagal nerve, NTS and hypothalamus (Buhmann et al., 2014; Lo et al., 2014). CCK increases gallbladder and gastrointestinal motility and secretion, in addition to playing a significant role in initiating the gut-brain axis to control food intake, energy expenditure and glucose utilisation (Cheung et al., 2009; Suzuki et al., 2012). Peripheral administration of CCK in animal studies regulates food intake in a dose-dependent manner and administration of CCK-1 receptor antagonists in conjunction with fatty-acid and protein consumption impedes the stimulation of vagal afferents lining the small intestine as well as the regulatory effects of CCK on food ingestion (Calingasan et al., 1992; Cox et al., 1996; Duca and Yue, 2014). Hence, these studies implicate CCK as a specific mediator of fat and protein satiation. Additionally, repeated doses of CCK into the systemic circulation and sporadic CCK infusion during feeding, initially decreases the amount of foodstuffs ingested, but over time, a tolerance to CCK develops and the quantity and frequency of ingestion increases (Kopin et al., 1999; Buhmann et al., 2014). This desensitising effect may explain the failed attempts to utilise CCK-derivatives, such as GI 181771X, as an effective weight-loss treatment (Castillo et al., 2004; Kim et al., 2011).
CCK administration conveys glucose-regulating effects, via increased vagal firing, which in turn induces the NDMA neurons of the NTS to increase hepatic vagal firing to signal the liver to decrease gluconeogenesis (Rasmussen et al., 2012). When rats are placed on a high-fat, high-carbohydrate diet, they develop CCK-resistance in response to the increased levels (Daly et al., 2011). The exact mechanisms how CCK administers its glucoregulatory effects and how CCK resistance develops remain unclear. However, these findings may provide an explanation as to why CCK-derivatives induce pancreatitis and contribute to developing an impaired utilisation of glucose. Further studies outlining the molecular physiology that CCK conveys on other organs, such as the liver requires further research.
Glucagon-Like Peptide 1 (GLP1)
GLP1 is a neuropeptide released predominantly from EECs of the ileum and colon in response to carbohydrate, lipid and/or protein ingestion (Elliott et al., 1993; Adam and Westerterp-Plantenga, 2005). It is synthesised by post-translational processing of the preproglucagon gene in the CNS and the GIT, exerting its effects via activating the GLP1 receptor, which is a type of GPCR expressed extensively throughout the CNS, GIT, and pancreas (Larsen et al., 1997; Yamato et al., 1997). Systemic and central GLP1 administration stimulates satiety centres in the brain, in particular, the ARC, paraventricular, NTS and AP to decrease hunger (Larsen et al., 1997; Abbott et al., 2005). Hence GLP1 is considered to be a pivotal factor leading to satiation. It is synthesised and released within 15 min post-prandial, which is intriguing given that intestinal L-cells are located distally in the ileum (Elliott et al., 1993; Bauer et al., 2016). Therefore, GLP1 release may be a reflex response involving vagal fibres located within the duodenum, given that these fibres are involved in early nutrient-sensing. Whilst this hypothesis has yet to be validated, recent studies have demonstrated the presence of GLP1-secreting EECs within the duodenum, indicating that GLP1 release may occur in two stages or in response to the hypothesised reflex (Svendsen et al., 2015). Whilst more elaborate studies involving nutrient infusion into sections of the small intestine are needed in order to determine the exact site/s GLP1 is secreted from, what is clear is that its release is relative to the energy intake and to all types of macromolecules to induce satiety.
GLP1 is a powerful incretin (a blood glucose-regulating peptide) that stimulates the GLP1 receptor of pancreatic β-cells to release insulin (Buhmann et al., 2014). Additionally, enhanced levels of GLP1 upregulate pancreatic β-cell gene expression of insulin promoter factor 1, thus promoting their development and impeding their apoptosis, which in turn contributes to improved glucose utilisation within the body (Villanueva-Peñacarrillo et al., 2001; Suzuki et al., 2012). Finally, GLP1 decreases the rate of gastric emptying into the duodenum and hinders gastric acid secretion, which in turn increases gastric distension, limits excessive food consumption, enhances satiety and positively contributes to energy homeostasis (Edwards et al., 1999).
Whilst the effects conveyed by GLP1 are potent, they are often brief as GLP1 is vulnerable to rapid degradation and inactivation through the catalytic function of dipeptidyl peptidase IV (DPPIV) (Deacon et al., 1995; Holst, 2007). Only 10% of intestinal-derived GLP1 reach the systemic circulation, indicating that it conveys its effects in a paracrine manner (Vilsbøll et al., 2003; Holst, 2007; Kuhre et al., 2015). Additionally, peripheral administration of GLP1 in conjunction with the removal of the vagus nerve impedes the effects of GLP1, whilst intravascular infusion of GLP1 continues to convey its effects in the presence of vagotomy and/or high doses of the neurotoxin capsaicin (Rüttimann et al., 2009; Zhang and Ritter, 2012). This indicates that the GLP1 receptor is located within the brain and that GLP1 may elicit higher-order functions, as a neurotransmitter, which are yet to be determined. Whilst GLP1 stimulates specific regions of the brain, such as the brainstem, to enhance motor output and/or thermogenesis, further studies are needed to determine the mechanism/s involving GLP1 and higher-order neural function with regard to energy homeostasis and food behaviour patterns (Li et al., 2009; Graaf et al., 2016). Additionally, Ohlsson et al. (Ohlsson et al., 2014) demonstrated that GLP1 concentrations rise rapidly post-prandial within the lymph and that these concentrations are sustained for longer intervals, as DPPIV is expressed at lower concentrations within the lymph than the general circulation. Hence, this may provide another pathway as to how GLP1 exerts its effects centrally and peripherally, as well as the role it may possess with respect to immune-signalling and the inflammatory state associated with obesity. Either way, future studies that aim to extend GLP1 function and/or mimic its function through the use of GLP1 receptor agonists, such as exenatide, may offer promise in relation to increasing satiety and regulating energy homeostasis and, therefore, treating obesity.
Peptide YY3−36 (PYY)
PYY is a small peptide belonging to the pancreatic-peptide family and, like GLP1, is secreted by intestinal L-cells post-prandial (Batterham et al., 2002, 2006). It is released in response to intestinal nutrient-sensing and in volumes that reflect the amount of energy consumed (Oesch et al., 2006). PYY is secreted with 15 min post-prandial, in a manner emulating GLP1 with regard to duodenal nutrient-sensing, increased vagal-firing and/or chemically-derived reactions (Fu-Cheng et al., 1997). Unlike CCK and GLP1 whose concentrations diminish rapidly, PYY concentrations remain elevated for several hours post-prandial (Batterham et al., 2003a). Hence, PYY effects may be prolonged and exhibited in a more endocrine fashion in comparison to CCK and GLP1.
PYY is present throughout the entire GIT, from the oesophagus through to the rectum (Adrian et al., 1985). PYY binds to the Y2 receptor and, in turn, decreases food intake, as studies using rodents lacking this receptor and PYY knockout mice become polyphagic and, consequently, gain weight (Batterham et al., 2006; le Roux et al., 2006). Additionally, PYY elicits activation of the NTS and POMC neurons in the ARC, via peripheral and central administration, indicating the presence of Y2 receptors on the vagus nerve, within the NTS and in the ARC (Batterham et al., 2002, 2006; Koda et al., 2005; le Roux et al., 2006). PYY exerts its effects by inhibiting NPY neurons, as Y2 receptors are expressed abundantly by these neurons in the ARC and their activation consequently impedes the orexigenic effects of NPY (Dryden et al., 1995; Broberger et al., 1997). Therefore, PYY may possess a pivotal role in energy homeostasis by regulating food intake and suppressing excessive consumption in an endocrine fashion, via activation of the POMC neurons and inhibition of NPY within the melanocortin system (Bauer et al., 2016). Additionally, studies indicate that obese subjects possess lower post-prandial PYY concentrations, whilst other studies suggested that there are vast differences between healthy and obese individuals with regard to fasting PYY concentrations (Batterham et al., 2003a; Korner et al., 2005; Stock et al., 2005). Augmented PYY concentrations are associated with gastrointestinal diseases such as inflammatory bowel disease and chronic destructive pancreatitis, in addition to prolonged appetite loss (El-Salhy et al., 2013). Additionally, sustained concentrations of PYY and CCK in the elderly are concomitant with delayed gastric emptying and reduced cholecystic contractility (Di Francesco et al., 2005; Buhmann et al., 2014). The mechanism as to why this occurs remains elusive. However, increased concentrations, which assist in long-term satiety and therefore a reduced energy-intake, may be linked to malnutrition in the elderly (Di Francesco et al., 2005; Buhmann et al., 2014). Hence, further studies are needed to determine the long-term effects of raised PYY concentrations before Y2 receptor agonist and/or PYY derivatives can be utilised as effective anti-obesogenic treatment.
Pancreatic Polypeptide
Pancreatic polypeptide (PP) belongs to the pancreatic-peptide family and is secreted by specialised F-cells within the pancreatic islets of Langerhans (Khandekar et al., 2015). Its release, like PYY, is proportional to caloric intake, where foods high in fat trigger an increased response (Guyenet and Schwartz, 2012). It is released systemically during the preabsorptive and post-prandial state, suggesting that its secretion emulates GLP1 and PYY with regard to duodenal nutrient-sensing, increased vagal-firing and/or chemically-derived reactions (Schwartz et al., 1978; Khandekar et al., 2015). Its concentration has been demonstrated to be sustained and elevated for up to 6 h post-prandial, thus suggesting an endocrine action (Adrian et al., 1976). Furthermore, PP is released in the colon and rectum of the bovine gut, where it acts as an exocrine hormone (Pyarokhil et al., 2012). This function has not been validated in human studies, hence it is unknown if it exerts an exocrine function within the human.
PP acts upon the Y4 receptor within the AP, NTS, and the ARC and concurrently induces gallbladder relaxation and inhibits pancreatic secretion, as it acts as a CCK antagonist, in addition to delaying gastric emptying, which leads to a rapidity of satiety and a decreased food consumption (Parker and Herzog, 1999; Balasubramaniam et al., 2006; Lin et al., 2009). The role PP plays with respect to appetite suppression is enhanced by studies demonstrating a difference in PP concentrations in anorexic and obesogenic states, where it is increased and diminished respectively (Batterham et al., 2003b). Whilst PP is a potent appetite suppressant, a seminal study conducted by Clark et al. (1984) demonstrated that central administration of PP stimulated appetite and led to an enhanced food intake. Whilst there is conflicting evidence that does not support these findings, the findings by Clark et al. (1984) have yet to be refuted and further studies are needed to determine if central-acting PP appeases or stimulates appetite. Moreover, studies in Prader-Willi syndrome and obese patients established a diminished level of PP post-prandial in comparison to healthy individuals and that intravenous PP injection in these patients led to a significant decline in food consumption (Lassmann et al., 1980; Berntson et al., 1993). PP-overexpressing mice have an increased incidence of mortality, which is resultant of a reduction in maternal milk consumption (Kohno and Yada, 2012). Whilst this can be portrayed as extreme, it validates the potential potency of PP with respect to satiation. Further, Obinepitide (7TM Pharma), a potent synthetic analogue of PP and a Y4 receptor agonist, has demonstrated decreases in both food intake and weight loss (Davenport and Wright, 2014). Although its use has been reported to be well tolerated with minimal adverse side effects, trials have only been conducted for 28 days (Davenport and Wright, 2014). Hence further studies are needed to establish the chronic effects that PP may possess before Y4 receptor agonists and PP-derived agents are utilised as potential anti-obesogenic treatments.
Oxyntomodulin
Oxyntomodulin is a peptide hormone secreted in response to nutrient ingestion. It is synthesised, like GLP1, by post-translational processing of the preproglucagon peptide within EECs of the gut and the CNS (Cohen et al., 2003; Baggio et al., 2004; Habib et al., 2012). Its secretion occurs concurrently with GLP1 and PYY and reaches its peak concentration within 30 min post-prandial before it is rapidly degraded by DPPIV (Anini et al., 1999; Druce et al., 2009). Oxyntomodulin binds to GLP1 receptors within the GIT, the pancreas and the ARC, to induce a decrease in gastric acid secretion and food consumption, as outlined previously in the GLP1 section of this review (Baggio et al., 2004; Dakin et al., 2004; Pocai et al., 2009). Central and peripheral oxyntomodulin administration enhances satiety and therefore decreases food consumption in rodent and human models, as well as increasing energy expenditure (Dakin et al., 2002, 2004; Cohen et al., 2003; Baggio et al., 2004; Wynne et al., 2005). Additionally, oxyntomodulin binds to glucagon receptors within the pancreas, lowers blood glucose concentrations and improves glucose utilisation (Maida et al., 2008). Whilst oxyntomodulin binds to the GLP1 receptor and is released concurrently with GLP1, the exact mechanism as to how oxyntomodulin functions as a potent incretin by binding to the glucagon receptor is largely unknown (Pocai et al., 2009; Pocai, 2014). It may act antagonistically to glucagon and induce an insulinotropic effect through a local, paracrine effect and/or it may activate higher centres of the brain via the hypothalamus. Since it has been reported to augment energy expenditure, it may also activate catecholaminergic and/or POMC neurons, as well as increase vagal activity at the site of brown fat, leading to increased thermogenesis (Dakin et al., 2002; Wynne et al., 2006; Pocai, 2014). Whilst these hypotheses are plausible, further studies are required to elucidate the mechanism/s involved in its incretin and thermogenic abilities before analogues are used in the treatment of obesity.
Serotonin
Enterochromaffin cells, which are specialised EECs, produce and secrete gut-derived serotonin in response to food intake (Bertrand and Bertrand, 2010). Serotonin induces its effects by acting locally and systemically on various 5-HT receptors such as the 5-HT2 receptor family and the 5-HT4 receptor expressed on vagal afferent fibres and other neurons within the CNS, as well as cells within the GIT, heart, and adrenal glands (Halford and Harrold, 2012; Li et al., 2015; Stiedl et al., 2015). Serotonin analogues, such as lorcaserin, suppress appetite and decrease body weight, whilst serotonin receptor antagonists induce the contrary and increase appetite and therefore body weight (Halford et al., 1997; Savastano et al., 2007; Lam et al., 2008; Smith et al., 2010). Whilst serotonin diminishes appetite through effects on the CNS and assists in weight-loss, the contrary has been demonstrated, where animals fed a westernised diet that in turn became obese, possess increased concentrations of serotonin (Crane et al., 2015). Additionally, Crane et al. (2015) demonstrated that the inhibition of peripheral serotonin synthesis reduced obesity and metabolic dysfunction, as serotonin blunted the effects of β-adrenergic neurons supplying brown adipose tissue, which decreased thermogenesis. Furthermore, serotonin analogues have been recently withdrawn from the market due to many undesirable and severe side-effects, such as psychiatric disorders, cardiotoxicity, drug addiction and death (Onakpoya et al., 2016). Hence the effects that serotonin may convey with respect to energy homeostasis and food behaviours are complex, require substantial further investigation and are outside the scope of this review.
Endocannabinoid System
Bioactive lipids belonging to the endocannabinoid system, such as anandamide, have been demonstrated to play a role in the gut-brain axis. These molecules are synthesised and secreted within the GIT and act upon endocannabinoid receptors, mainly cannabinoid receptors 1 and 2 (CB1/CB2), which are GPCRs within the endocannabinoid system (Moran and Shanahan, 2014; Bauer et al., 2016). CB1 is distributed abundantly throughout the CNS and the peripheral nervous system and expressed in the liver, pancreas and adipose tissue, whilst CB2 is predominantly expressed by immune cells, in addition to the brain, pancreas, and adipose tissue (Mackie, 2008). The endocannabinoid regulates various physiological functions, such as regulating gut motility and appetite, which is interesting given that the administration of exogenous cannabinoids, such marijuana, convey orexigenic effects (Mackie, 2008; Moran and Shanahan, 2014). Hence, the development of CB1 antagonists, such as rimonabant and tarabant, were utilised to induce weight-loss in obese individuals, thus demonstrating the role the endocannabinoid system plays in increasing appetite (Christensen et al., 2007; Aronne et al., 2010; Cluny et al., 2011). However, these products were withdrawn from the market, as they conveyed severe psychological side-effects such as chronic depression (Aronne et al., 2010; Moran and Shanahan, 2014).
Obesity is concomitant with enhanced endocannabinoid tone, CB1 expression, and endocannabinoid concentrations within the plasma and adipose tissues (Izzo et al., 2009; Moran and Shanahan, 2014). These findings are supported by studies demonstrating that CB1 deficient mice are resistant to diet-induced obesity and possess enhanced leptin sensitivity, which acts to inhibit hunger and increase satiety (Ravinet Trillou et al., 2004; Cluny et al., 2011). Finally, anandamide is increased during food deprivation and induces hunger by inhibiting CB1-expressing vagal afferents, which consequently blockade vagal-firing to the CNS and, therefore, may lead to a diminished effect conveyed by other gut hormones (Gómez et al., 2002; Kentish and Page, 2015). Whilst this hypothesis is yet to be confirmed, the role endocannabinoids play in relation to appetite and energy homeostasis needs to be explored, particularly since the endocannabinoid system is intricately associated with stress, memory, immune function and mood (Mackie, 2008). Hence, factors affecting these physiological and psychological functions may be associated with various food behaviours, which may be amplified in obesity. Interestingly, there is new evidence that endocannabinoids, such as anandamide, bind to Transient Receptor Potential Vanilloid 1 (TRPV1), which is located abundantly throughout most cell types in the body (Puente et al., 2011; Abdel-Salam, 2014). This is of interest as TRPV1 activation, with the use of low-dose dietary capsaicin, increases thermogenesis, suppresses appetite, improves gastrointestinal function and enhances weight-loss (Kawabata et al., 2009; Ludy et al., 2011; Ono et al., 2011; Abdel-Salam, 2014; Janssens et al., 2014). Therefore, it is evident that there is a knowledge gap with respect to the endocannabinoids and association with other physiological receptors that need to be explored and that the association of the endocannabinoids and other physiological systems are intricate and complex.
Energy Expenditure
As outlined above, obesity is a consequence of enhanced energy consumption and a decline in energy expenditure. A sedentary lifestyle and diminished energy expenditure augments weight-gain (Grima and Dixon, 2013). Energy expenditure does not just involve physical activity, it also involves thermogenesis and basal metabolic rate, even though physical activity can increase both parameters (Melanson, 2017). There are various studies demonstrating that fat-loss can occur without an increase in physical activity, indicating that energy expenditure can occur without regimented exercise and by administration of a particular intervention (Panchal et al., 2012, 2013; Owen Bryn et al., 2014). These studies retrospectively challenge previous dogma that held exercise as the “gold-standard” in terms of regulating energy and therefore fat-loss (Melanson, 2017). Additionally, the evident rise in health and fitness centres throughout many westernised-countries, such as Australia, and use of these centres, further suggests that physical activity may not lead to enhanced fat-loss without being used in conjunction with an appropriate calorie-controlled diet (Australia, 2009). Hence, gut-derived neurohumoral signals within the gut-brain axis can activate energy-regulating cortices in response to nutrient consumption to influence energy expenditure, in addition to energy consumption, thus contributing to a favourable energy balance (Bauer et al., 2016).
Peripheral and central administration of GLP1, oxyntomodulin and PYY leads to enhanced energy expenditure by increasing thermogenesis and basal metabolic rate (Dakin et al., 2002; Blouet and Schwartz, 2012). Blouet and Schwartz (2012) demonstrated that intestinal lipid-sensing activates vagal afferent fibres to enhance brown adipose tissue (BAT) thermogenesis through a CCK-dependent pathway. This suggests that a gut-brain-BAT axis may exist and is further enhanced by studies that incorporate an intervention as a treatment to induce weight-loss (Blouet and Schwartz, 2012; Panchal et al., 2012, 2013; Brown et al., 2015). An example of this is low-dose dietary capsaicin, which increases gut-derived vagal firing, augments hormone secretions, enhances sympathetic tone, and activates BAT, leading to an increased basal metabolic rate and thermogenesis, which culminates in weight-loss (Kawabata et al., 2009; Ludy et al., 2011; Ono et al., 2011; Abdel-Salam, 2014; Janssens et al., 2014). Hence, exploration of this potential axis may assist in treating obesity and its associated comorbidities, via focusing on the thermogenic properties a treatment or dietary intervention may convey.
The Gut Microbiota
There is increasing evidence that the gut microbiota may influence adiposity and weight-gain through several inter-dependent pathways, including energy harvest and subsequent generation of metabolites, such as short-chain fatty-acids (SCFA), modification of host behaviour, satiety through the gut-brain axis and effects on inflammatory responses within the host (Moran and Shanahan, 2014).
There are in excess of 3.8–3.9 × 1013 bacterial cells colonising a healthy human body, a majority of which reside within the GIT and comprise the complex ecosystem referred to as the gut microbiota (Sender et al., 2016a,b). The microbial to human cell ratio has recently been revised to approximately 1.3:1 from the historical 10:1 ratio (Sender et al., 2016a,b). Nonetheless, these microbes form an intricate symbiotic relationship with the host, where the host provides a nutrient-dense environment for the microbiota and the microbiota, in turn, provides metabolic, protective and structural functions, which are not encoded for by the host's genome (Qin et al., 2010; Wang and Wang, 2016). The microbiota is thought to be comprised of more than 1000 different bacterial species (Figure 3) and this composition alters throughout the lifespan due to factors such as diet, antibiotic use, disease states, delivery method at birth and most elements that a modern lifestyle incorporates (Qin et al., 2010). Hence, its composition is not static and the changes to its structure are dynamic.
The gut microbiota conveys a vast impact on the host's metabolism and was first outlined in a seminal study conducted by Wostmann et al. (1983). Wostmann et al. (1983) demonstrated that mice lacking a microbiota (germ-free) possessed reduced adiposity, energy intake, and energy extraction from a standard rodent diet compared to conventionally-raised mice. Additionally, Wostmann et al. (1983) collated a series of studies outlining the developmental anomalies associated with germ-free rearing compared to a conventionally-raised upbringing (Table 3), which has been validated by recent studies (Heijtz et al., 2011; Cho et al., 2012; Al-Asmakh and Zadjali, 2015). This highlights the extent of the symbiotic relationship between the host and the microbiota and how microbiota modification can impact an individual's health status. Hence, manipulation of the microbiota with regard to studying the effects related to host physiology would be more meaningful than the use of germ-free rodents (Bauer et al., 2016). This is particularly valid with respect to metabolic studies, as high-fat high-carbohydrate feeding alters the microbiota's composition and diversity within a short period of time (David et al., 2014).
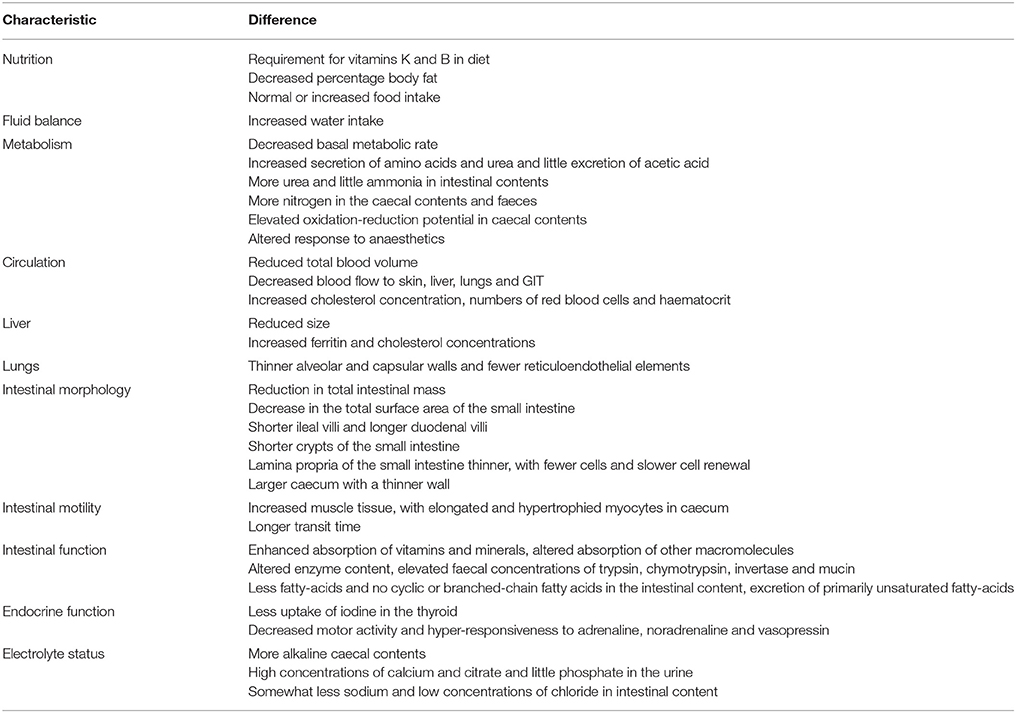
Table 3. Anatomical and physiological differences in germ-free mice compared to wild-type mice (Al-Asmakh and Zadjali, 2015).
De Filippo et al. (2010) compared and characterised the differences between healthy children from either a westernised-diet or a rural-diet and demonstrated distinct differences between the microbiota and consumed foodstuffs. Whilst De Filippo et al. (2010) examined children from Italy who were presumed to eat a westernised-diet, it did not consider that these children may have been reared on the “Mediterranean-diet,” which is considered as the “gold-standard” diet with respect to healthy eating (Sánchez-Villegas et al., 2016). What was clear were the differences between to the two cultures, the food consumed and, therefore, the microbiota composition. This study, as well as others, suggested that the westernised-diet and obesity, are associated with an increased ratio of bacteria belonging to the Firmicutes phylum compared to the Bacteroidetes phylum, which is reversed upon surgical and dietary interventions (Ley et al., 2006; Turnbaugh et al., 2009; De Filippo et al., 2010; Furet et al., 2010). Controversy exists with the Firmicute-to-Bacertoidetes ratio as a guide for determining the obese phenotype, as more recent studies have failed to validate this hypothesis (Zhang et al., 2009; Schwiertz et al., 2010; Finucane et al., 2014). Hence, differences at the genus and species level compared to the phyla level may be associated with changes in metabolic function (Bauer et al., 2016).
Duca et al. (2014) demonstrated distinct genera differences in germ-free rats when they were transplanted with the microbiota of either obese-prone or obese-resistant rats. Duca et al. (2014) observed 25 operational taxonomic units (OTUs) in the obese donors and recipients that were lacking entirely in the obese-resistant donors and recipients, and that these additional 25 OTUs possessed the ability to harvest extra energy from the diet. This study confirms the findings of earlier studies that suggested a possible link between obesity and a microbiome rich in genes responsible for the production of enzymes involved in energy harvesting from indigestible carbohydrates (Turnbaugh et al., 2009; Duca et al., 2014). The relationship between the microbiota composition, energy harvest and obesity is more complex than suggested, as studies have demonstrated that energy harvest and metabolite production, in the form of SCFA, are not correlated with increased weight-gain and that some SCFA may possess a beneficial role with respect to host metabolism and energy regulation (Tims et al., 2013; Bauer et al., 2016).
Short-Chain Fatty-Acids, Energy Harvest and Nutrient-Sensing
Approximately 60 g of dietary carbohydrates consumed daily part of the typical western diet are indigestible. The gut microbiota possesses specific glycoside hydrolases, enabling them to ferment and hydrolyse indigestible polysaccharides and produce SCFA as a metabolite within the distal colon (Moran and Shanahan, 2014). This function and subsequent generation of SCFA provides approximately 10% of the host's daily energy requirements (Schwiertz et al., 2010). Butyrate, propionate and acetate account for 95% of the biologically significant SCFA produced (Bauer et al., 2016). Colonocytes utilise butyrate as their primary energy source, the liver utilises propionate in gluconeogenesis after it has entered the portal circulation and acetate is circulated systemically to various peripheral tissues (Gao et al., 2009; Bauer et al., 2016). Butyrate production is typically attributed to Firmicutes, whilst propionate synthesis is generally associated with Bacteroidetes (Moran and Shanahan, 2014). In the obesogenic state, faeces contain an increased quantity of SCFA, in particular, propionate (Schwiertz et al., 2010). This increase in faecal SCFA content is proposed to be due to a change in microbiota composition, rather than differences in diet and/or SCFA absorption within the colon (Schwiertz et al., 2010; Rahat-Rozenbloom et al., 2014). Interestingly, Rahat-Rozenbloom et al. (2014) reported a higher proportion of Firmicutes than Bacteroidetes in the overweight cohort, which would correlate with an increase in butyrate production rather than propionate production. Hence, further studies are required to determine the differences between obese and lean individuals and why there is an increase in faecal SCFA content. Furthermore, it is interesting that SCFA, which have been demonstrated to possess anti-carcinogen properties, are increased in the obesogenic state, given that a high-fat high-carbohydrate diet is one predisposing factor attributed to the development of colorectal cancer (Bindels et al., 2012; Grima and Dixon, 2013; Irrazábal et al., 2014). Hence, further studies are required to determine the role SCFA play with respect to the development of colorectal cancer in obesity, as it could be argued that possessing a profile mirroring a slightly overweight state, where SCFA production is slightly increased, could be gastroprotective and that increased weight leading to obesity, may be detrimental.
SCFA also assist in regulating body weight, as administration of prebiotics, indigestible polysaccharides and oral and intestinal SCFA infusion lead to an enhanced metabolic state, a reduction in food consumption and a decrease in body weight (Pan et al., 2009; Bomhof et al., 2014). This occurs as prebiotics and supplements promote the growth and activity of favourable microbial species, whilst SCFA administration activates signalling pathways, resulting in an increase in gut hormone synthesis (Pan et al., 2009; Lin et al., 2012; Bomhof et al., 2014). Hence, SCFA can be considered as pivotal endogenous signalling molecules. SCFA bind and activate free fatty-acid receptors 2 and 3 (FFAR2/FFAR3), which are GPCRs located throughout the GIT, immune cells, liver and adipose tissue (Kasubuchi et al., 2015). Within the GIT, the expression of these receptors has been localised to EECs, in particular, L-cells (Kasubuchi et al., 2015). Once bound to these receptors, L-cells are signalled to synthesise and release gut hormones, such as GLP1 and PYY (Table 2). These findings are further enhanced by in vivo and in vitro studies, which have demonstrated that cell cultures and mice lacking FFAR2 and FFAR3 have impaired GLP1 and PYY release, even in the presence of SCFA infusion (Tolhurst et al., 2012). Additionally, FFAR3 is predominantly expressed within the peripheral nervous system, in particular, the ENS and ANS (Nøhr et al., 2015). Activation of these receptors within the sympathetic branch of the ANS regulate storage mechanisms within adipose tissue and influence energy expenditure via stimulating muscle and liver tissues to regulate glucose utilisation (Moran and Shanahan, 2014; Nøhr et al., 2015). Whilst the exact mechanisms involved are yet to be elucidated, it can be appreciated that the evidence conveyed thus far implicates SCFA synthesis by the microbiota to signal and stimulate the gut-brain axis.
Intestinal epithelial cells and their absorptive and secretory ability may be influenced by the gut microbiota by acting through the gut-brain axis (Bauer et al., 2016). Studies have demonstrated diminished concentrations of FFAR2 and FFAR3, increased expression of glucose transporters and sweet-taste receptors, increased sucrose consumption and absorption, and a diminished expression of the long-chain fatty-acid receptor GPCR 120 (GPR120) in germ-free mice (Duca et al., 2012; Swartz et al., 2012). GPR120 activation conveys the anti-inflammatory and insulin-sensitising effects of omega-3 fatty-acids, whilst its absence in GPR120 knock-out mice decreases fat metabolism and, therefore, increases the occurrence of obesity (Oh et al., 2010; Ichimura et al., 2012). Hence, germ-free mice possess diminished concentrations of CCK, GLP1 and PYY, which decreases the ability of germ-free mice to sense nutrients within the gut and send regulatory feedback signals through the gut-brain axis and, therefore, leads to enhanced food-intake (Duca et al., 2012; Swartz et al., 2012). Additionally, Fredborg et al. (2012) demonstrated that GPR120 can be upregulated and that GLP1 expression can decrease in the presence of specific bacterial strains in vitro. This suggests that changes in microbiota composition may alter intestinal nutrient-sensing and gut-hormone synthesis. While this hypothesis is yet to be established, further studies are needed to validate this and the role of receptors such as GPR120 and the mechanisms that their activation initiates.
Prebiotics, such as oligofructose, improve gut-barrier function, induce weight-loss and reduce food intake, by improving gut nutrient-sensing mechanisms that initiate these effects (Bauer et al., 2016). It has been suggested that these effects are driven by alterations in the gut microbiota composition. Studies have demonstrated diminished levels of specific types of bacteria and gut-derived peptides in the obesogenic state and used this premise in an attempt to restore these bacteria, in addition to increasing the circulating concentrations of gut hormones (Bauer et al., 2016). Prebiotic administration increases Akkermansia muciniphilia, Faecalibacterium prausnitzii, Bifidobacterium, and Lactobacilli, which in turn, have been linked to improvements in the gut-barrier function through a glucose-like peptide 2 (GLP2) mediated pathway and an increase in endocannabinoid signalling (Cani et al., 2009; Dewulf et al., 2012). GLP2 is co-secreted with GLP1 from EECs and its release has been positively associated with intestinal growth and function by increasing villus height, crypt-cell depth and proliferation and decreasing enterocyte cell-death (Rowland et al., 2011). Additionally, prebiotic treatment has been associated with improved EEC differentiation and concentrations of GLP1, GIP and PYY, which increase satiety, decrease food consumption, and decrease adiposity (Cani et al., 2005; Neyrinck et al., 2012). Whilst more studies are required to confirm the linkage between manipulation of gut microbiota composition and gut hormone production, there is evidence that the microbiota may influence nutrient-sensing by the GIT, production of gut hormones and stimulation of the gut-brain axis.
The gut microbiota may also communicate to adipose tissue via the endocannabinoid system. Various mouse models have demonstrated that the peripheral endocannabinoid system in the intestinal and adipose tissues, which possesses roles in regulating gut-barrier function and adipogenesis, are regulated by the gut microbiota (Muccioli et al., 2010). Prebiotic administration to select for an increase in Bifidobacterium within obese mice induce a decline in colonic CB1 expression and anandamide concentrations, in addition to increased colonic fatty-acid amide hydrolase (FAAH) expression (Muccioli et al., 2010; Moran and Shanahan, 2014). FAAH is the primary enzyme responsible for the degradation of anandamide. Decreases in these factors within the colon, as well as an increase in FAAH expression, suggests that the gut microbiota may selectively modulate colonic CB1 receptors, which subsequently moderates endocannabinoid tone (Muccioli et al., 2010). More studies are required to replicate these findings and subsequently elucidate how the microbiota may affect the endocannabinoid system and the increased tone associated with the obesogenic state. The increase in these colonic factors may be resultant of enhanced concentrations of gut-derived hormones rather than the direct composition of the microbiota or the microbiota may induce increased signalling of gut hormones to alter changes within the endocannabinoid system via vagal afferent stimulation. Determining the mechanism/s involved may convey additional insights on how the gut-brain axis and the microbiota are linked in relation to energy regulation and therefore can be targeted for the treatment of obesity.
Lipopolysaccharide, Inflammation and Microbiota Integration into the Gut-Brain Axis
Obesity is considered to be an inflammatory state, as it is characterised by the presence of chronic low-grade inflammation. It has been recently discovered that the westernised-diet, which is high in calories and can lead to obesity and various other obesity-related diseases (Table 1) is associated with elevated systemic lipopolysaccharide (LPS) concentrations (Cani et al., 2007; de La Serre et al., 2010). LPS is the pro-inflammatory component within the cell wall of gram-negative bacteria. LPS is thought to enter the systemic circulation through compromised intestinal epithelial functioning associated with high-fat diets and obesity. This process is termed metabolic endotoxaemia and is hypothesised to occur due to an unfavourable change in the gut microbiota composition, which induces the intestinal epithelium to increase the gap between the junctions formed by each cell, thus increasing gut permeability and permitting the translocation of macronutrients and other molecules, such as LPS (Figure 4) (Cani et al., 2007; de La Serre et al., 2010). The leakage of LPS into the circulation initiates a cascade of pro-inflammatory events throughout the host, especially within white adipose tissue.
LPS is a potent activator of toll-like receptor 4 (TLR4), whose activation results in the synthesis of inflammatory cytokines and subsequent activation of the innate immune system (Vaure and Liu, 2014). Caesar et al. (2015) reported increased systemic LPS concentration, TLR4 activation and white adipose tissue inflammation, as well as reduced insulin-sensitivity and alterations in the gut microbiota composition in mice fed a westernised-diet.
Increased LPS concentrations are concurrent with increased gut concentrations of TLR4 in obese-prone rats (DIO-P), whilst obese-resistant rats (DIO-R) do not express TLR4 within the gut (de La Serre et al., 2010, 2015). The DIO-P rats possessed increases in Clostridiales orders and a decrease in Bifidobacterium, which led to an increase in LPS concentration (de La Serre et al., 2010). de La Serre et al. (2015) reported decreased concentrations of intestinal alkaline phosphatase (IAP) within DIO-P rats, whilst the contrary was observed in the DIO-R rats. This is of significance as IAP is an enzyme native to the gut and responsible for the detoxification of LPS, thus suggesting that LPS may directly act on the gut. This hypothesis is further enhanced by Everard et al. (2014) who demonstrated that intestinal deletion of MyD88, a central adaptor molecule for the majority of toll-like receptors, including TLR4, partially protected obese-prone mice and germ-free mice who received a faecal microbiota transplant from obese-prone mice from diet-induced obesity and inflammation. Additionally, Everard et al. (2014) suggest that deletion of MyD88 may improve nutritional status and provide a therapeutic target for obesity. Further studies are needed to confirm this hypothesis, as TLR4 is also expressed by vagal afferents, thus indicating that LPS may initiate an inflammatory cascade within the neuronal circuitry pertaining to the gut-brain axis (de La Serre et al., 2015).
LPS conveys inhibitory effects on the interstitial cells of Cajal, which function as a pacemaker, creating a slow-wave potential leading to smooth muscle contraction in the gut (peristalsis) and regulation of the ENS (Zuo et al., 2013; Bauer et al., 2016). Inhibition of these cells are associated with gastrointestinal motility disorders, by altering the frequency of neurotransmitter release of neurons within the ENS, which may affect the release of gut hormones and, therefore, link the gut microbiota to impaired gut-brain axis signalling mechanisms (Zuo et al., 2013). Further studies are needed to confirm this hypothesis, as the mechanisms linking this particular region of the peripheral nervous system to the CNS and the gut-brain axis are unknown. Future studies could, for example, explore the option of restoring function to the interstitial cells of Cajal by possibly increasing IAP concentration through intestinal infusion to degrade LPS formation, which may assist in decreasing inflammatory bowel disorders associated with obesity.
The gut microbiota may directly communicate and alter CNS signalling mechanisms. Whilst it is still relatively unclear the impact the gut microbiota may have on CNS signalling, with respect to regulation of energy homeostasis, it is becoming evident that the gut microbiota can influence CNS-mediated stress and anxiety behaviours (Bauer et al., 2016). Studies have established differences between germ-free mice and specific pathogen-free mice, which include motor control, memory formation and anxiety due to central neurochemical changes, in particular, those involving brain-derived neurotrophic factor, serotonin, dopamine, noradrenaline, and NMDA receptor expression (Bercik et al., 2010; Heijtz et al., 2011; Neufeld et al., 2011; Steenbergen et al., 2015; Wang and Wang, 2016). Additionally, probiotic treatment, in particular, treatment selective for an increase in Bifidobacterium and Lactobacilli, reduces anxiety in mice with inflammatory bowel disease, enhances cognitive reactivity to depressed mood in humans and reduces depression scores in patients with irritable bowel syndrome, as well as improving hypothalamus-pituitary axis responses to acute psychological trauma (Ait-Belgnaoui et al., 2012; Steenbergen et al., 2015; Pinto-Sanchez et al., 2017). These alterations in behaviour appear to be correlated with vagal activity and diminished LPS-induced inflammation which improves gut barrier function and prevents gut leakage (Bercik et al., 2010). Whilst it can be proposed that specific-pathogenic bacteria, which increase in number during stress and high-fat feeding, release LPS which activates TLR4 on vagal afferents, thus signalling the hypothalamus and other higher-order centres of the brain to integrate and induce an appropriate behaviour, further studies are needed to confirm this hypothesis. Further, Lactobacillus rhamnosus CGMCC1.3724 supplementation amplified fat-loss in obese women, in addition to decreasing systemic concentrations of leptin and the relative abundance of Lachnospiraceae, which is a subfamily of the Firmicutes phylum (Sanchez et al., 2014). These findings were replicated in a later study using the same probiotic, in addition to an increase in both satiety and body self-esteem scores, as well as a decrease in both food cravings and depression (Sanchez et al., 2017). Additionally, the vagus nerve can be activated by non-pathogenic bacteria, such as Lactobacillus lactis, and this activation enhances sympathetic nervous system activities, whilst subdiaphragmatic vagotomy in the presence of Lactobacillus lactis attenuates these effects (Tanida et al., 2005; Forsythe and Kunze, 2013). A summary of these studies can be seen in Figure 5. The mechanism/s elucidating how non-pathogens activate the vagus nerve is yet to be determined and add further complexity into how this gut-brain-microbiota interaction may arise.
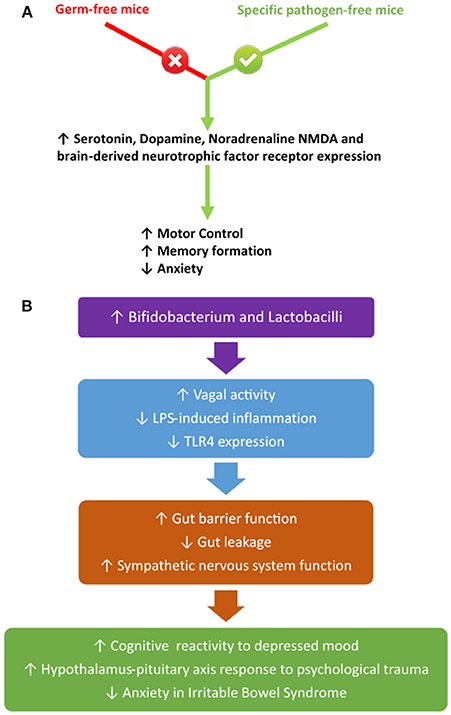
Figure 5. The influence of different bacterial species on the vagus nerve (A) and its systemic impact (B).
It is becoming more evident that the gut microbiota impacts CNS functions related to the regulation of energy homeostasis. Bäckhed et al. (2004) demonstrated a resistance to adiposity despite an increased food intake in germ-free mice, thus suggesting that the microbiota directly or indirectly influence the CNS. This finding was the premise for a more elaborate study conducted by Schéle et al. (2013), who compared the gene expression of food intake-regulating peptides and hypothalamic and brainstem feeding circuits between germ-free and normally-reared mice. Schéle et al. (2013) reported a diminished expression of GCG which codes for preproglucagon, the precursor peptide for GLP1, GLP2 and oxyntomodulin, within the brainstem and hypothalamus. They also reported diminished leptin-responsiveness in the conventionally-raised mice in comparison to the germ-free mice (Schéle et al., 2013). Additionally, when conventionally-raised mice were treated with leptin, they failed to suppress gene expression of the orexigenic peptides NPY and AgRP within the hypothalamus and brainstem, thus suggesting that the gut-microbiota can directly reduce the expression of anorexigenic peptides and subsequent pathways and affect energy homeostasis, thus leading to an increase in adiposity (Schéle et al., 2013, 2016). Future studies would benefit by determining how specific manipulations of the gut microbiota phenotype can influence the CNS and its role in regulating energy homeostasis and the development of obesity. Additionally, since altered concentrations of gut hormones have been linked to changes in higher neural functions, such as sleep, arousal and anxiety, future studies may be able to link the impact that the gut microbiota conveys on local and central neural-signalling pathways and how this pertains to energy regulation through a microbiota-gut-brain axis (Figure 6) (Forsythe and Kunze, 2013).
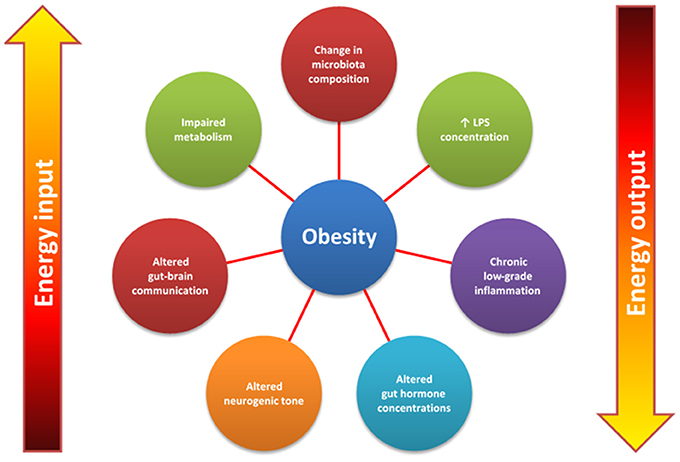
Figure 6. Summary of the effects of an altered microbiota on the gut-brain axis contributing to obesity. This figure summarises the different factors determinants, which have been mentioned throughout this review, that link the gut microbiota with the gut-brain axis in the development of obesity. These include a change in the microbiota composition, increased LPS concentrations culminating in an increase in gut permeability and chronic low-grade inflammation, as well as an increase in energy intake and decrease in energy expenditure.
Conclusion
Obesity is a global epidemic that causes socioeconomic strain on governments and public-healthcare systems. Current evidence suggests that life-expectancy will decline as the obesity rate surges and becomes uncontrolled (Olshansky et al., 2005; Grima and Dixon, 2013). Currently, there are limited efficacious treatments available to combat obesity, as current treatment options include alterations in lifestyle and diets, as well as surgical and pharmacological treatments with poorly understood mechanisms resulting in various side-effects (Bauer et al., 2016). Currently, the most effective and sustained treatment option is surgical intervention achieved through gastric bypass surgery, in which the mechanisms leading to its success are poorly understood (Grima and Dixon, 2013). However, analysing alterations in gut hormone concentrations, neuronal circuitry and gut microbiota composition (that is the components of the gut-brain-microbiota axis) post-surgery and why these factors change may assist in the development of new treatment strategies. Given that surgery can increase the population of EECs, which leads to an increased production of peptides and neuronal communication, as well increased post-prandial gut hormone secretion, it is imperative that the dimensions of the gut-brain axis are elucidated to assist in developing future treatments (Mumphrey et al., 2013; Bauer et al., 2016). Additionally, increasing energy expenditure, through the activation of the sympathetic branches of ANS and the possible gut-brain-BAT axis in addition to targeting neurohormone production may be effective in regulating energy balance. Furthermore, studies implicating the role of splanchnic and other somatosensory pathways are needed to be conducted so that it can be understood what role/s these fibres possess within the gut-brain axis, especially given the large presence of these afferents throughout the GIT. However, exploring the exact mechanisms related to this potential axis, ancillary neuronal pathways and fibres, and integrating the pathways involved in relation to the knowledge already obtained regarding the gut-brain axis may prove complex, but with persistence may provide a promising strategy for combating obesity.
Manipulation of the gut microbiota may provide a novel therapeutic strategy in combating obesity and its comorbidities (Bauer et al., 2016). Rapid and persistent shifts in the gut microbiota have been reported to be concomitant with improved metabolic parameters post-surgery (Liou et al., 2013; Osto et al., 2013). Additionally, rodent studies involving the microbiota transplant of post-surgery rodents to germ-free rodents have demonstrated to possess diminished adiposity and increased energy expenditure resulting from modified SCFA production and/or diminished LPS concentrations (Liou et al., 2013; Casselbrant et al., 2015). Whilst manipulating microbiota composition may provide a promising lead in developing anti-obesogenic treatments, more studies are required to elucidate the “ideal” microbiota phenotype with respect to the “healthy” state. Granted prebiotics and probiotics have provided promising insights into the role the microbiota plays within the gut-brain axis and the obesogenic state (Moran and Shanahan, 2014). It is unknown how long the changes in a favourable phenotype may take to occur. Given the “fast-pace” and “convenient” lifestyle associated with modern-living, the compliance associated with taking these supplements may be poor if an improvement is not seen rapidly. Additionally, capsules comprised of what is considered to be an ideal microbiota phenotype, and faecal microbiota transplants have shown promising results in rats and willing participants (Ley et al., 2006; Turnbaugh et al., 2009; Smits et al., 2013). Again, this treatment option may be limited in efficacy, solely due to the psychological aspects that may not have been taken into consideration, such as the simplistic viewpoint that this ultimately is the ingestion of faecal bacteria harvested from a healthy individual. Hence, public education and health promotion programmes would need to be implemented to increase compliance and efficacy. Additionally, these programs could assist in public education with respect to lifestyle choices and the development of the microbiota throughout the lifespan.
Whilst, understanding of the complex interactions associated with the gut-brain-microbiota axis and obesity are in their infancy and understanding of this axis is increasing rapidly, it provides a promising area for future treatments. These advances in knowledge and possible treatment options should complement rather than substitute research addressing the lifestyle and psychological factors that are associated with obesity. This will, therefore, optimistically provide an improved outcome and compliance for future endeavours in alleviating this epidemic.
Author Contributions
EB conceived and wrote the manuscript and EW offered advice and critically revised the manuscript. Both authors approved it for publication.
Funding
EB and EW are supported by internal funding from the University of Southern Queensland.
Conflict of Interest Statement
The authors declare that the research was conducted in the absence of any commercial or financial relationships that could be construed as a potential conflict of interest.
Acknowledgments
The authors would like to acknowledge the University of Southern Queensland for assistance in funding the production of this manuscript.
References
Abbott, C. R., Monteiro, M., Small, C. J., Sajedi, A., Smith, K. L., Parkinson, J. R. C., et al. (2005). The inhibitory effects of peripheral administration of peptide YY3–36 and glucagon-like peptide-1 on food intake are attenuated by ablation of the vagal–brainstem–hypothalamic pathway. Brain Res. 1044, 127–131. doi: 10.1016/j.brainres.2005.03.011
Adam, T. C., and Westerterp-Plantenga, M. S. (2005). Glucagon-like peptide-1 release and satiety after a nutrient challenge in normal-weight and obese subjects. Br. J. Nutr. 93, 845–851. doi: 10.1079/BJN20041335
Adrian, T. E., Bloom, S. R., Bryant, M. G., Polak, J. M., Heitz, P. H., and Barnes, A. J. (1976). Distribution and release of human pancreatic polypeptide. Gut 17, 940–944. doi: 10.1136/gut.17.12.940
Adrian, T. E., Ferri, G. L., Bacarese-Hamilton, A. J., Fuessl, H. S., Polak, J. M., and Bloom, S. R. (1985). Human distribution and release of a putative new gut hormone, peptide YY. Gastroenterology 89, 1070–1077. doi: 10.1016/0016-5085(85)90211-2
Ait-Belgnaoui, A., Durand, H., Cartier, C., Chaumaz, G., Eutamene, H., Ferrier, L., et al. (2012). Prevention of gut leakiness by a probiotic treatment leads to attenuated HPA response to an acute psychological stress in rats. Psychoneuroendocrinology 37, 1885–1895. doi: 10.1016/j.psyneuen.2012.03.024
Al-Asmakh, M., and Zadjali, F. (2015). Use of germ-free animal models in microbiota-related research. J. Microbiol. Biotechnol. 25, 1583–1588. doi: 10.4014/jmb.1501.01039
Amato, A., Cinci, L., Rotondo, A., Serio, R., Faussone-pellegrini, M. S., Vannucchi, M. G., et al. (2010). Peripheral motor action of glucagon-like peptide-1 through enteric neuronal receptors. Neurogastroenterol. Motility 22, 664–e203. doi: 10.1111/j.1365-2982.2010.01476.x
Anini, Y., Fu-Cheng, X., Cuber, J. C., Kervran, A., Chariot, J., and Roz,é, C. (1999). Comparison of the postprandial release of peptide YY and proglucagon-derived peptides in the rat. Pflügers Archiv. 438, 299–306. doi: 10.1007/s004240050913
Arendas, K., Qiu, Q., and Gruslin, A. (2008). Obesity in pregnancy: pre-conceptional to postpartum consequences. J. Obstet. Gynaecol. Can. 30, 477–488. doi: 10.1016/S1701-2163(16)32863-8
Aronne, L. J., Tonstad, S., Moreno, M., Gantz, I., Erondu, N., Suryawanshi, S., et al. (2010). A clinical trial assessing the safety and efficacy of taranabant, a CB1R inverse agonist, in obese and overweight patients: a high-dose study. Int. J. Obes. 34, 919–935. doi: 10.1038/ijo.2010.21
Aune, D., Sen, A., Norat, T., Janszky, I., Romundstad, P., Tonstad, S., et al. (2016). Body mass index, abdominal fatness, and heart failure incidence and mortality: a systematic review and dose–response meta-analysis of prospective studies. Circulation 133, 639–649. doi: 10.1161/CIRCULATIONAHA.115.016801
Australia, F. (2009). Let's Get Physical: The Economic Contribution of Fitness Centres in Australia. Access Economics.
Babic, T., Townsend, R. L., Patterson, L. M., Sutton, G. M., Zheng, H., and Berthoud, H.-R. (2009). Phenotype of neurons in the nucleus of the solitary tract that express CCK-induced activation of the ERK signaling pathway. Am. J. Physiol. Regul. Integr. Compar. Physiol. 296, R845–R854. doi: 10.1152/ajpregu.90531.2008
Bäckhed, F., Ding, H., Wang, T., Hooper, L. V., Koh, G. Y., Nagy, A., et al. (2004). The gut microbiota as an environmental factor that regulates fat storage. Proc. Natl. Acad. Sci. U.S.A. 101, 15718–15723. doi: 10.1073/pnas.0407076101
Baggio, L. L., Huang, Q., Brown, T. J., and Drucker, D. J. (2004). Oxyntomodulin and glucagon-like peptide-1 differentially regulate murine food intake and energy expenditure. Gastroenterology 127, 546–558. doi: 10.1053/j.gastro.2004.04.063
Balasubramaniam, A., Mullins, D. E., Lin, S., Zhai, W., Tao, Z., Dhawan, V. C., et al. (2006). Neuropeptide Y (NPY) Y4 receptor selective agonists based on NPY(32–36): development of an anorectic Y4 receptor selective agonist with picomolar affinity. J. Med. Chem. 49, 2661–2665. doi: 10.1021/jm050907d
Batterham, R. L., Cohen, M. A., Ellis, S. M., Le Roux, C. W., Withers, D. J., Frost, G. S., et al. (2003a). Inhibition of food intake in obese subjects by peptide YY3–36. New Engl. J. Med. 349, 941–948. doi: 10.1056/NEJMoa030204
Batterham, R. L., Cowley, M. A., Small, C. J., Herzog, H., Cohen, M. A., Dakin, C. L., et al. (2002). Gut hormone PYY3-36 physiologically inhibits food intake. Nature. 418, 650–654. doi: 10.1038/nature00887
Batterham, R. L., Heffron, H., Kapoor, S., Chivers, J. E., Chandarana, K., Herzog, H., et al. (2006). Critical role for peptide YY in protein-mediated satiation and body-weight regulation. Cell Metab. 4, 223–233. doi: 10.1016/j.cmet.2006.08.001
Batterham, R. L., Le Roux, C. W., Cohen, M. A., Park, A. J., Ellis, S. M., Patterson, M., et al. (2003b). Pancreatic polypeptide reduces appetite and food intake in humans. J. Clin. Endocrinol. Metab. 88, 3989–3992. doi: 10.1210/jc.2003-030630
Bauer, P. V., Hamr, S. C., and Duca, F. A. (2016). Regulation of energy balance by a gut–brain axis and involvement of the gut microbiota. Cell. Mol. Life Sci. 73, 737–755. doi: 10.1007/s00018-015-2083-z
Bedendi, I., Alloatti, G., Marcantoni, A., Malan, D., Catapano, F., Ghé, C., et al. (2003). Cardiac effects of ghrelin and its endogenous derivatives des-octanoyl ghrelin and des-Gln14-ghrelin. Eur. J. Pharmacol. 476, 87–95. doi: 10.1016/S0014-2999(03)02083-1
Bercik, P., Verdu, E. F., Foster, J. A., Macri, J., Potter, M., Huang, X., et al. (2010). Chronic gastrointestinal inflammation induces anxiety-like behavior and alters central nervous system biochemistry in mice. Gastroenterology 139, 2102–2112.e1. doi: 10.1053/j.gastro.2010.06.063
Berntson, G. G., Zipf, W. B., O'Dorisio, T. M., Hoffman, J. A., and Chance, R. E. (1993). Pancreatic polypeptide infusions reduce food intake in Prader-Willi syndrome. Peptides 14, 497–503. doi: 10.1016/0196-9781(93)90138-7
Berthoud, H.-R., Kressel, M., Raybould, H. E., and Neuhuber, W. L. (1995). Vagal sensors in the rat duodenal mucosa: distribution and structure as revealed by in vivo DiI-tracing. Anat. Embryol. 191, 203–212. doi: 10.1007/BF00187819
Bertrand, P. P., and Bertrand, R. L. (2010). Serotonin release and uptake in the gastrointestinal tract. Autonomic Neurosci. Basic Clin. 153, 47–57. doi: 10.1016/j.autneu.2009.08.002
Bertrand, R. L., Senadheera, S., Markus, I., Liu, L., Howitt, L., Chen, H., et al. (2011). A Western diet increases serotonin availability in rat small intestine. Endocrinology 152, 36–47. doi: 10.1210/en.2010-0377
Beydoun, M. A., Beydoun, H. A., and Wang, Y. (2008). Obesity and central obesity as risk factors for incident dementia and its subtypes: a systematic review and meta-analysis. Obesity Rev. 9, 204–218. doi: 10.1111/j.1467-789X.2008.00473.x
Bianchini, F., Kaaks, R., and Vainio, H. (2002). Overweight, obesity, and cancer risk. Lancet Oncol. 3, 565–574. doi: 10.1016/S1470-2045(02)00849-5
Billington, C. J., Briggs, J. E., Link, J. G., and Levine, A. S. (1991). Glucagon in physiological concentrations stimulates brown fat thermogenesis in vivo. Am. J. Physiol. Regul. Integr. Comparat. Physiol. 261, R501–R507. doi: 10.1152/ajpregu.1991.261.2.R501
Bindels, L. B., Porporato, P., Dewulf, E. M., Verrax, J., Neyrinck, A. M., Martin, J. C., et al. (2012). Gut microbiota-derived propionate reduces cancer cell proliferation in the liver. Br. J. Cancer. 107, 1337–1344. doi: 10.1038/bjc.2012.409
Blackburn, A. M., Bloom, S. R., Long, R. G., Fletcher, D. R., Christofides, N. D., Fitzpatrick, M. L., et al. (1980). Effect of neurotensin on gastric function in man. Lancet 315, 987–989. doi: 10.1016/S0140-6736(80)91434-8
Blouet, C., and Schwartz, G. J. (2012). Duodenal lipid sensing activates vagal afferents to regulate non-shivering brown fat thermogenesis in rats. PLoS ONE 7:e51898. doi: 10.1371/journal.pone.0051898
Bomhof, M. R., Saha, D. C., Reid, D. T., Paul, H. A., and Reimer, R. A. (2014). Combined effects of oligofructose and Bifidobacterium animalis on gut microbiota and glycemia in obese rats. Obesity 22, 763–771. doi: 10.1002/oby.20632
Boyer, J. L., and Bloomer, J. R. (1974). Canalicular bile secretion in man studies utilizing the biliary clearance of [(14)C]mannitol. J. Clin. Investig. 54, 773–781. doi: 10.1172/JCI107817
Bremer, A. A., Devaraj, S., Afify, A., and Jialal, I. (2011). Adipose tissue dysregulation in patients with metabolic syndrome. J. Clin. Endocrinol. Metab. 96, E1782–E1788. doi: 10.1210/jc.2011-1577
Broberger, C., Landry, M., Wong, H., Walsh, J. N., and Hökfelt, T. (1997). Subtypes Y1 and Y2 of the neuropeptide Y Receptor Are Respectively Expressed in Pro-opiomelanocortin- and neuropeptide-Y-containing neurons of the rat hypothalamic arcuate nucleus. Neuroendocrinology 66, 393–408. doi: 10.1159/000127265
Brown, L., Poudyal, H., and Panchal, S. K. (2015). Functional foods as potential therapeutic options for metabolic syndrome. Obesity Rev. 16, 914–941. doi: 10.1111/obr.12313
Buhmann, H., le Roux, C. W., and Bueter, M. (2014). The gut–brain axis in obesity. Best Pract. Res. Clin. Gastroenterol. 28, 559–571. doi: 10.1016/j.bpg.2014.07.003
Bump, R. C., Sugerman, H. J., Fantl, J. A., and McClish, D. K. (1992). Obesity and lower urinary tract function in women: effect of surgically induced weight loss. Am. J. Obstet. Gynecol. 167, 392–397; discussion 7–9. doi: 10.1016/S0002-9378(11)91418-5
Caesar, R., Tremaroli, V., Kovatcheva-Datchary, P., Cani Patrice, D., and Bäckhed, F. (2015). Crosstalk between gut microbiota and dietary lipids aggravates WAT inflammation through TLR signaling. Cell Metabolism 22, 658–668. doi: 10.1016/j.cmet.2015.07.026
Calingasan, N., Ritter, S., Ritter, R., and Brenner, L. (1992). Low-dose near-celiac arterial cholecystokinin suppresses food intake in rats. Am. J. Physiol. Regul. Integr. Comparat. Physiol. 263, R572–R5777. doi: 10.1152/ajpregu.1992.263.3.R572
Campos, C. A., Shiina, H., Silvas, M., Page, S., and Ritter, R. C. (2013). Vagal afferent NMDA receptors modulate CCK-induced reduction of food intake through synapsin I phosphorylation in adult male rats. Endocrinology 154, 2613–2625. doi: 10.1210/en.2013-1062
Cani, P. D., Amar, J., Iglesias, M. A., Poggi, M., Knauf, C., Bastelica, D., et al. (2007). Metabolic endotoxemia initiates obesity and insulin resistance. Diabetes 56, 1761–1772. doi: 10.2337/db06-1491
Cani, P. D., Neyrinck, A. M., Maton, N., and Delzenne, N. M. (2005). Oligofructose promotes satiety in rats fed a high-fat diet: involvement of glucagon-like peptide-1. Obes. Res. 13, 1000–1007. doi: 10.1038/oby.2005.117
Cani, P. D., Possemiers, S., Van de Wiele, T., Guiot, Y., Everard, A., Rottier, O., et al. (2009). Changes in gut microbiota control inflammation in obese mice through a mechanism involving GLP-2-driven improvement of gut permeability. Gut 58, 1091–1103. doi: 10.1136/gut.2008.165886
Casselbrant, A., Elias, E., Fändriks, L., and Wallenius, V. (2015). Expression of tight-junction proteins in human proximal small intestinal mucosa before and after Roux-en-Y gastric bypass surgery. Surg. Obes. Relat. Dis. 11, 45–53. doi: 10.1016/j.soard.2014.05.009
Castillo, E. J., Delgado-Aros, S., Camilleri, M., Burton, D., Stephens, D., O'Connor-Semmes, R., et al. (2004). Effect of oral CCK-1 agonist GI181771X on fasting and postprandial gastric functions in healthy volunteers. Am. J. Physiol. Gastrointest. Liver Physiol. 287, G363–G369. doi: 10.1152/ajpgi.00074.2004
Chen, S. M., Xiong, G. S., and Wu, S. M. (2012). Is obesity an indicator of complications and mortality in acute pancreatitis? An updated meta-analysis. J. Digest. Dis. 13, 244–251. doi: 10.1111/j.1751-2980.2012.00587.x
Cheng, C. Y., Chu, J. Y., and Chow, B. K. (2011). Central and peripheral administration of secretin inhibits food intake in mice through the activation of the melanocortin system. Neuropsychopharmacology 36, 459–471. doi: 10.1038/npp.2010.178
Cheung, G. W., Kokorovic, A., Lam, C. K., Chari, M., and Lam, T. K. (2009). Intestinal cholecystokinin controls glucose production through a neuronal network. Cell Metab. 10, 99–109. doi: 10.1016/j.cmet.2009.07.005
Cho, H.-J., Kosari, S., Hunne, B., Callaghan, B., Rivera, L. R., Bravo, D. M., et al. (2015). Differences in hormone localisation patterns of K and L type enteroendocrine cells in the mouse and pig small intestine and colon. Cell Tissue Res. 359, 693–698. doi: 10.1007/s00441-014-2033-3
Cho, I., Yamanishi, S., Cox, L., Methe, B. A., Zavadil, J., Li, K., et al. (2012). Antibiotics in early life alter the murine colonic microbiome and adiposity. Nature 488, 621–626. doi: 10.1038/nature11400
Christensen, R., Kristensen, P. K., Bartels, E. M., Bliddal, H., and Astrup, A. (2007). Efficacy and safety of the weight-loss drug rimonabant: a meta-analysis of randomised trials. Lancet 370, 1706–1713. doi: 10.1016/S0140-6736(07)61721-8
Chu, J. Y. S., Chung, S. C. K., Lam, A. K. M., Tam, S., Chung, S. K., and Chow, B. K. C. (2007). Phenotypes developed in secretin receptor-null mice indicated a role for secretin in regulating renal water reabsorption. Mol. Cell. Biol. 27, 2499–2511. doi: 10.1128/MCB.01088-06
Clark, J. T., Kalra, P. S., Crowley, W. R., and Kalra, S. P. (1984). Neuropeptide Y and human pancreatic polypeptide stimulate feeding behavior in rats. Endocrinology 115, 427–429. doi: 10.1210/endo-115-1-427
Cluny, N. L., Chambers, A. P., Vemuri, V. K., Wood, J. T., Eller, L. K., Freni, C., et al. (2011). The neutral cannabinoid CB1 receptor antagonist AM4113 regulates body weight through changes in energy intake in the rat. Pharmacol. Biochem. Behav. 97, 537–543. doi: 10.1016/j.pbb.2010.10.013
Cohen, M. A., Ellis, S. M., Le Roux, C. W., Batterham, R. L., Park, A., Patterson, M., et al. (2003). Oxyntomodulin suppresses appetite and reduces food intake in humans. J. Clin. Endocrinol. Metab. 88, 4696–4701. doi: 10.1210/jc.2003-030421
Cone, R., Cowley, M., Butler, A., Fan, W., Marks, D., and Low, M. (2001). The arcuate nucleus as a conduit for diverse signals relevant to energy homeostasis. Int. J. Obes. 25:S63. doi: 10.1038/sj.ijo.0801913
Cooke, A. R., and Clark, E. D. (1976). Effect of first part of duodenum on gastric emptying in dogs: response to acid, fat, glucose, and neural blockade. Gastroenterology 70, 550–555.
Costa, M., Brookes, S. J. H., and Hennig, G. W. (2000). Anatomy and physiology of the enteric nervous system. Gut 47(Suppl. 4):iv15–iv9. doi: 10.1136/gut.47.suppl_4.iv15
Cowley, M. A., Smart, J. L., Rubinstein, M., Cerdan, M. G., Diano, S., Horvath, T. L., et al. (2001). Leptin activates anorexigenic POMC neurons through a neural network in the arcuate nucleus. Nature 411, 480–484. doi: 10.1038/35078085
Cox, J. E., McCown, S. M., Bridges, J. M., and Tyler, W. J. (1996). Inhibition of sucrose intake by continuous celiac, superior mesenteric, and intravenous CCK-8 infusions. Am. J. Physiol. Regul. Integr. Compar. Physiol. 270, R319–R325. doi: 10.1152/ajpregu.1996.270.2.R319
Craig, A. D. (1996). Chapter 13 An ascending general homeostatic afferent pathway originating in lamina I. Prog. Brain Res. 107, 225–242. doi: 10.1016/S0079-6123(08)61867-1
Crane, J. D., Palanivel, R., Mottillo, E. P., Bujak, A. L., Wang, H., Ford, R. J., et al. (2015). Inhibiting peripheral serotonin synthesis reduces obesity and metabolic dysfunction by promoting brown adipose tissue thermogenesis. Nat. Med. 21, 166–172. doi: 10.1038/nm.3766
Dakin, C. L., Small, C. J., Batterham, R. L., Neary, N. M., Cohen, M. A., Patterson, M., et al. (2004). Peripheral oxyntomodulin reduces food intake and body weight gain in rats. Endocrinology 145, 2687–2695. doi: 10.1210/en.2003-1338
Dakin, C. L., Small, C. J., Park, A. J., Seth, A., Ghatei, M. A., and Bloom, S. R. (2002). Repeated ICV administration of oxyntomodulin causes a greater reduction in body weight gain than in pair-fed rats. Am. J. Physiol. Endocrinol. Metab. 283, E1173–E1177. doi: 10.1152/ajpendo.00233.2002
Daly, D. M., Park, S. J., Valinsky, W. C., and Beyak, M. J. (2011). Impaired intestinal afferent nerve satiety signalling and vagal afferent excitability in diet induced obesity in the mouse. J. Physiol. 589, 2857–2870. doi: 10.1113/jphysiol.2010.204594
Date, Y., Murakami, N., Toshinai, K., Matsukura, S., Niijima, A., Matsuo, H., et al. (2002). The role of the gastric afferent vagal nerve in ghrelin-induced feeding and growth hormone secretion in rats. Gastroenterology 123, 1120–1128. doi: 10.1053/gast.2002.35954
Davenport, R. J., and Wright, S. (2014). Treating obesity: is it all in the gut? Drug Discov. Today. 19, 845–858. doi: 10.1016/j.drudis.2013.10.025
David, L. A., Maurice, C. F., Carmody, R. N., Gootenberg, D. B., Button, J. E., Wolfe, B. E., et al. (2014). Diet rapidly and reproducibly alters the human gut microbiome. Nature 505, 559–563. doi: 10.1038/nature12820
Davis, J. D., and Smith, G. P. (1990). Learning to sham feed: behavioral adjustments to loss of physiological postingestional stimuli. Am. J. Physiol. Regul. Integr. Compar. Physiol. 259, R1228–R1235. doi: 10.1152/ajpregu.1990.259.6.R1228
De Filippo, C., Cavalieri, D., Di Paola, M., Ramazzotti, M., Poullet, J. B., Massart, S., et al. (2010). Impact of diet in shaping gut microbiota revealed by a comparative study in children from Europe and rural Africa. Proc. Natl. Acad. Sci. U.S.A. 107, 14691–14696. doi: 10.1073/pnas.1005963107
de La Serre, C. B., de Lartigue, G., and Raybould, H. E. (2015). Chronic exposure to low dose bacterial lipopolysaccharide inhibits leptin signaling in vagal afferent neurons. Physiol. Behav. 139, 188–194. doi: 10.1016/j.physbeh.2014.10.032
de La Serre, C. B., Ellis, C. L., Lee, J., Hartman, A. L., Rutledge, J. C., and Raybould, H. E. (2010). Propensity to high-fat diet-induced obesity in rats is associated with changes in the gut microbiota and gut inflammation. Am. J. Physiol. Gastrointestinal Liver Physiol. 299, G440–G448. doi: 10.1152/ajpgi.00098.2010
Deacon, C. F., Johnsen, A. H., and Holst, J. J. (1995). Degradation of glucagon-like peptide-1 by human plasma in vitro yields an N-terminally truncated peptide that is a major endogenous metabolite in vivo. J. Clin. Endocrinol. Metab. 80, 952–957.
Depoortere, I. (2014). Taste receptors of the gut: emerging roles in health and disease. Gut 63, 179–190. doi: 10.1136/gutjnl-2013-305112
Dewulf, E. M., Cani, P. D., Claus, S. P., Fuentes, S., Puylaert, P. G., Neyrinck, A. M., et al. (2012). Insight into the prebiotic concept: lessons from an exploratory, double blind intervention study with inulin-type fructans in obese women. Gut 62, 1112–1121. doi: 10.1136/gutjnl-2012-303304
Di Francesco, V., Zamboni, M., Dioli, A., Zoico, E., Mazzali, G., Omizzolo, F., et al. (2005). Delayed postprandial gastric emptying and impaired gallbladder contraction together with elevated cholecystokinin and peptide YY serum levels sustain satiety and inhibit hunger in healthy elderly persons. J. Gerontol. Series A. 60, 1581–1585. doi: 10.1093/gerona/60.12.1581
DiBaise, J. K., and Foxx-Orenstein, A. E. (2013). Role of the gastroenterologist in managing obesity. Expert Rev. Gastroenterol. Hepatol. 7, 439–451. doi: 10.1586/17474124.2013.811061
Dicker, A., Zhao, J., Cannon, B., and Nedergaard, J. (1998). Apparent thermogenic effect of injected glucagon is not due to a direct effect on brown fat cells. Am. J. Physiol. Regul. Integr. Comparat. Physiol. 275, R1674–R1682. doi: 10.1152/ajpregu.1998.275.5.R1674
Dimaline, R., and Varro, A. (2014). Novel roles of gastrin. J. Physiol. 592, 2951–2958. doi: 10.1113/jphysiol.2014.272435
Dimitriadis, G., Mitrou, P., Lambadiari, V., Maratou, E., and Raptis, S. A. (2011). Insulin effects in muscle and adipose tissue. Diabetes Res. Clin. Pract. 93, S52–S59. doi: 10.1016/S0168-8227(11)70014-6
Dirksen, C., Jørgensen, N. B., Bojsen-Møller, K. N., Kielgast, U., Jacobsen, S. H., Clausen, T. R., et al. (2013). Gut hormones, early dumping and resting energy expenditure in patients with good and poor weight loss response after Roux-en-Y gastric bypass. Int. J. Obes. 37, 1452–1459. doi: 10.1038/ijo.2013.15
Dixon, J. B., Lambert, E. A., and Lambert, G. W. (2015). Neuroendocrine adaptations to bariatric surgery. Mol. Cell. Endocrinol. 418, 143–152. doi: 10.1016/j.mce.2015.05.033
Dockray, G. J. (2013). Enteroendocrine cell signalling via the vagus nerve. Curr. Opin. Pharmacol. 13, 954–958. doi: 10.1016/j.coph.2013.09.007
Druce, M. R., Minnion, J. S., Field, B. C. T., Patel, S. R., Shillito, J. C., Tilby, M., et al. (2009). Investigation of structure-activity relationships of oxyntomodulin (Oxm) using oxm analogs. Endocrinology 150, 1712–1721. doi: 10.1210/en.2008-0828
Dryden, S., Pickavance, L., Frankish, H. M., and Williams, G. (1995). Increased neuropeptide Y secretion in the hypothalamic paraventricular nucleus of obese (fa/fa) Zucker rats. Brain Res. 690, 185–188. doi: 10.1016/0006-8993(95)00628-4
Duca, F. A., and Yue, J. T. Y. (2014). Fatty acid sensing in the gut and the hypothalamus: in vivo and in vitro perspectives. Mol. Cell. Endocrinol. 397, 23–33. doi: 10.1016/j.mce.2014.09.022
Duca, F. A., Sakar, Y., Lepage, P., Devime, F., Langelier, B., Doré, J., et al. (2014). Replication of obesity and associated signaling pathways through transfer of microbiota from obese prone rat. Diabetes 63, 1624–1636. doi: 10.2337/db13-1526
Duca, F. A., Swartz, T. D., Sakar, Y., and Covasa, M. (2012). Increased oral detection, but decreased intestinal signaling for fats in mice lacking gut microbiota. PLoS ONE 7:e39748. doi: 10.1371/journal.pone.0039748
Edwards, C. M., Todd, J. F., Mahmoudi, M., Wang, Z., Wang, R. M., Ghatei, M. A., et al. (1999). Glucagon-like peptide 1 has a physiological role in the control of postprandial glucose in humans: studies with the antagonist exendin 9-39. Diabetes 48, 86–93. doi: 10.2337/diabetes.48.1.86
Eisner, M. D., Blanc, P. D., Sidney, S., Yelin, E. H., Lathon, P. V., Katz, P. P., et al. (2007). Body composition and functional limitation in COPD. Respir. Res. 8:7. doi: 10.1186/1465-9921-8-7
Ejerblad, E., Fored, C. M., Lindblad, P., Fryzek, J., McLaughlin, J. K., and Nyrén, O. (2006). Obesity and risk for chronic renal failure. J. Am. Soc. Nephrol. 17, 1695–1702. doi: 10.1681/ASN.2005060638
Elias, C. F., Aschkenasi, C., Lee, C., Kelly, J., Ahima, R. S., Bjorbæk, C., et al. (1999). Leptin differentially regulates, NPYand POMC neurons projecting to the lateral hypothalamic area. Neuron 23, 775–786. doi: 10.1016/S0896-6273(01)80035-0
Elliott, R. M., Morgan, L. M., Tredger, J. A., Deacon, S., Wright, J., and Marks, V. (1993). Glucagon-like peptide-1(7–36)amide and glucose-dependent insulinotropic polypeptide secretion in response to nutrient ingestion in man: acute post-prandial and 24-h secretion patterns. J. Endocrinol. 138, 159–166. doi: 10.1677/joe.0.1380159
El-Salhy, M., Mazzawi, T., Gundersen, D., Hatlebakk, J. G., and Hausken, T. (2013). The role of peptide YY in gastrointestinal diseases and disorders (Review). Int. J. Mol. Med. 31, 275–282. doi: 10.3892/ijmm.2012.1222
Enriori, P. J., Evans, A. E., Sinnayah, P., Jobst, E. E., Tonelli-Lemos, L., Billes, S. K., et al. (2007). Diet-induced obesity causes severe but reversible leptin resistance in arcuate melanocortin neurons. Cell Metab. 5, 181–194. doi: 10.1016/j.cmet.2007.02.004
Eslick, G. D. (2012). Gastrointestinal symptoms and obesity: a meta-analysis. Obesity Rev. 13, 469–479. doi: 10.1111/j.1467-789X.2011.00969.x
Esposito, K., Giugliano, F., Di Palo, C., Giugliano, G., Marfella, R., D'Andrea, F., et al. (2004). Effect of lifestyle changes on erectile dysfunction in obese men: a randomized controlled trial. JAMA 291, 2978–2984. doi: 10.1001/jama.291.24.2978
Everard, A., Geurts, L., Caesar, R., Van Hul, M., Matamoros, S., Duparc, T., et al. (2014). Intestinal epithelial MyD88 is a sensor switching host metabolism towards obesity according to nutritional status. Nat. Commun. 5:5648. doi: 10.1038/ncomms6648
Farooqi, I. S., Keogh, J. M., Yeo, G. S. H., Lank, E. J., Cheetham, T., and O'Rahilly, S. (2003). Clinical spectrum of obesity and mutations in the melanocortin 4 receptor gene. New Engl. J. Med. 348, 1085–1095. doi: 10.1056/NEJMoa022050
Fawaz, C. S., Martel, P., Leo, D., and Trudeau, L.-E. (2009). Presynaptic action of neurotensin on dopamine release through inhibition of D2 receptor function. BMC Neurosci. 10:96. doi: 10.1186/1471-2202-10-96
Feifel, D., Goldenberg, J., Melendez, G., and Shilling, P. D. (2010). The acute and subchronic effects of a brain-penetrating, neurotensin-1 receptor agonist on feeding, body weight and temperature. Neuropharmacology 58, 195–198. doi: 10.1016/j.neuropharm.2009.07.001
Finucane, M. M., Sharpton, T. J., Laurent, T. J., and Pollard, K. S. (2014). A taxonomic signature of obesity in the microbiome? Getting to the guts of the matter. PLoS ONE 9:e84689. doi: 10.1371/journal.pone.0084689
Flegal, K. M., Graubard, B. I., Williamson, D. F., and Gail, M. H. (2007). Cause-specific excess deaths associated with underweight, overweight, and obesity. JAMA 298, 2028–2037. doi: 10.1001/jama.298.17.2028
Forsythe, P., and Kunze, W. A. (2013). Voices from within: gut microbes and the CNS. Cell. Mol. Life Sci. 70, 55–69. doi: 10.1007/s00018-012-1028-z
Fredborg, M., Theil, P. K., Jensen, B. B., and Purup, S. (2012). G protein-coupled receptor120 (GPR120) transcription in intestinal epithelial cells is significantly affected by bacteria belonging to the bacteroides, proteobacteria, and Firmicutes phyla1. J. Anim. Sci. 90(Suppl. 4), 10–12. doi: 10.2527/jas.53792
Fu-Cheng, X., Anini, Y., Chariot, J., Castex, N., Galmiche, J.-P., and Rozé, C. (1997). Mechanisms of peptide YY release induced by an intraduodenal meal in rats: neural regulation by proximal gut. Pflügers Archiv. 433, 571–579. doi: 10.1007/s004240050316
Furet, J.-P., Kong, L.-C., Tap, J., Poitou, C., Basdevant, A., Bouillot, J.-L., et al. (2010). Differential adaptation of human gut microbiota to bariatric surgery–induced weight loss. Links with metabolic and low-grade inflammation markers. Diabetes 59, 3049–3057. doi: 10.2337/db10-0253
Gao, Z., Yin, J., Zhang, J., Ward, R. E., Martin, R. J., Lefevre, M., et al. (2009). Butyrate improves insulin sensitivity and increases energy expenditure in mice. Diabetes 58, 1509–1517. doi: 10.2337/db08-1637
Ghanim, H., Aljada, A., Hofmeyer, D., Syed, T., Mohanty, P., and Dandona, P. (2004). Circulating mononuclear cells in the obese are in a proinflammatory state. Circulation 110, 1564–1571. doi: 10.1161/01.CIR.0000142055.53122.FA
Gibbs, J., Maddison, S., and Rolls, E. T. (1981). Satiety role of the small intestine examined in sham-feeding rhesus monkeys. J. Comp. Physiol. Psychol. 95, 1003–1015. doi: 10.1037/h0077845
Gibbs, J., Young, R. C., and Smith, G. P. (1973). Cholecystokinin elicits satiety in rats with open gastric fistulas. Nature. 245, 323–325. doi: 10.1038/245323a0
Lu, Y., Hajifathalian, K., Ezzati, M., Woodward, M., Rimm, E. B., et al. (2014). Metabolic mediators of the effects of body-mass index, overweight, and obesity on coronary heart disease and stroke: a pooled analysis of 97 prospective cohorts with 1·8 million participants. Lancet 383, 970–983. doi: 10.1016/S0140-6736(13)61836-X
Gómez, R., Navarro, M., Ferrer, B., Trigo, J. M., Bilbao, A., Del Arco, I., et al. (2002). A peripheral mechanism for CB1 cannabinoid receptor-dependent modulation of feeding. J. Neurosci. 22, 9612–9617. doi: 10.1523/JNEUROSCI.22-21-09612.2002
Graaf, C., Donnelly, D., Wootten, D., Lau, J., Sexton, P. M., Miller, L. J., et al. (2016). Glucagon-like peptide-1 and its class B G protein–coupled receptors: a long march to therapeutic successes. Pharmacol. Rev. 68, 954–1013. doi: 10.1124/pr.115.011395
Gray, M. A., Greenwell, J. R., and Argent, B. E. (1988). Secretin-regulated chloride channel on the apical plasma membrane of pancreatic duct cells. J. Membr. Biol. 105, 131–142. doi: 10.1007/BF02009166
Grenham, S., Clarke, G., Cryan, J., and Dinan, T. (2011). Brain–gut–microbe communication in health and disease. Front. Physiol. 2:94. doi: 10.3389/fphys.2011.00094
Gribble, F. M., and Reimann, F. (2016). Enteroendocrine cells: chemosensors in the intestinal epithelium. Annu. Rev. Physiol. 78, 277–299. doi: 10.1146/annurev-physiol-021115-105439
Grima, M., and Dixon, J. (2013). Obesity: recommendations for management in general practice and beyond. Aust. Fam. Physician 42, 532–541. Available online at: https://search.informit.com.au/documentSummary;dn=487012525790449;res=IELHEA
Grunddal, K. V., Ratner, C. F., Svendsen, B., Sommer, F., Engelstoft, M. S., Madsen, A. N., et al. (2016). Neurotensin is coexpressed, coreleased, and acts together with GLP-1 and PYY in enteroendocrine control of metabolism. Endocrinology 157, 176–194. doi: 10.1210/en.2015-1600
Guerra, S., Sherrill, D. L., Bobadilla, A., Martinez, F. D., and Barbee, R. A. (2002). The relation of body mass index to asthma, chronic bronchitis, and emphysema. Chest. 122:1256. doi: 10.1378/chest.122.4.1256
Guyenet, S. J., and Schwartz, M. W. (2012). Regulation of food intake, energy balance, and body fat mass: implications for the pathogenesis and treatment of obesity. J. Clin. Endocrinol. Metab. 97, 745–755. doi: 10.1210/jc.2011-2525
Habib, A. M., Richards, P., Cairns, L. S., Rogers, G. J., Bannon, C. A. M., Parker, H. E., et al. (2012). Overlap of endocrine hormone expression in the mouse intestine revealed by transcriptional profiling and flow cytometry. Endocrinology 153, 3054–3065. doi: 10.1210/en.2011-2170
Halford, J. C. G., and Harrold, J. A. (2012). “5-HT2C receptor agonists and the control of appetite,” in Appetite Control, ed H.-G. Joost (Berlin, Heidelberg: Springer Berlin), 349–356.
Halford, J. C. G., Lawton, C. L., and Blundell, J. E. (1997). The 5-HT2 receptor agonist MK-212 reduces food intake and increases resting but prevents the behavioural satiety sequence. Pharmacol. Biochem. Behav. 56, 41–46. doi: 10.1016/S0091-3057(96)00152-9
Hall, J. E. (2015). Guyton and Hall Textbook of Medical Physiology. Saint Louis, MO: Elsevier Health Sciences.
Hamr, S. C., Wang, B., Swartz, T. D., and Duca, F. A. (2015). Does nutrient sensing determine how we “see” food? Curr. Diab. Rep. 15:38. doi: 10.1007/s11892-015-0604-7
Hay, D. L., Chen, S., Lutz, T. A., Parkes, D. G., and Roth, J. D. (2015). Amylin: pharmacology, physiology, and clinical potential. Pharmacol. Rev. 67, 564–600. doi: 10.1124/pr.115.010629
Hayes, A. J., Lung, T. W. C., Bauman, A., and Howard, K. (2017). Modelling obesity trends in Australia: unravelling the past and predicting the future. Int. J. Obes. 41, 178–185. doi: 10.1038/ijo.2016.165
Heijtz, R. D., Wang, S., Anuar, F., Qian, Y., Björkholm, B., Samuelsson, A., et al. (2011). Normal gut microbiota modulates brain development and behavior. Proc. Natl. Acad. Sci. U.S.A. 108, 3047–3052. doi: 10.1073/pnas.1010529108
Higham, C. E., Hull, R. L., Lawrie, L., Shennan, K. I. J., Morris, J. F., Birch, N. P., et al. (2000). Processing of synthetic pro-islet amyloid polypeptide (proIAPP) ‘amylin’ by recombinant prohormone convertase enzymes, PC2 and PC3, in vitro. Eur. J. Biochem. 267, 4998–5004. doi: 10.1046/j.1432-1327.2000.01548.x
Hilbert, A., Braehler, E., Haeuser, W., and Zenger, M. (2014). Weight bias internalization, core self-evaluation, and health in overweight and obese persons. Obesity 22, 79–85. doi: 10.1002/oby.20561
Holst, J. J. (2007). The physiology of glucagon-like peptide 1. Physiol. Rev. 87, 1409–1439. doi: 10.1152/physrev.00034.2006
Ichimura, A., Hirasawa, A., Poulain-Godefroy, O., Bonnefond, A., Hara, T., Yengo, L., et al. (2012). Dysfunction of lipid sensor GPR120 leads to obesity in both mouse and human. Nature 483, 350–354. doi: 10.1038/nature10798
Irrazábal, T., Belcheva, A., Girardin Stephen, E., Martin, A., and Philpott Dana, J. (2014). The multifaceted role of the intestinal microbiota in colon cancer. Mol. Cell. 54, 309–320. doi: 10.1016/j.molcel.2014.03.039
Izzo, A. A., Piscitelli, F., Capasso, R., Aviello, G., Romano, B., Borrelli, F., et al. (2009). Peripheral endocannabinoid dysregulation in obesity: relation to intestinal motility and energy processing induced by food deprivation and re-feeding. Br. J. Pharmacol. 158, 451–461. doi: 10.1111/j.1476-5381.2009.00183.x
Janssens, P. L., Hursel, R., and Westerterp-Plantenga, M. S. (2014). Capsaicin increases sensation of fullness in energy balance, and decreases desire to eat after dinner in negative energy balance. Appetite 77, 46–51. doi: 10.1016/j.appet.2014.02.018
Jones, B. J., Tan, T., and Bloom, S. R. (2012). Minireview: glucagon in stress and energy homeostasis. Endocrinology 153, 1049–1054. doi: 10.1210/en.2011-1979
Jutel, M., Watanabe, T., Klunker, S., Akdis, M., Thomet, O. A. R., Malolepszy, J., et al. (2001). Histamine regulates T-cell and antibody responses by differential expression of H1 and H2 receptors. Nature 413, 420–425. doi: 10.1038/35096564
Kalafatakis, K., and Triantafyllou, K. (2011). Contribution of neurotensin in the immune and neuroendocrine modulation of normal and abnormal enteric function. Regul. Pept. 170, 7–17. doi: 10.1016/j.regpep.2011.04.005
Kanneganti, T.-D., and Dixit, V. D. (2012). Immunological complications of obesity. Nat. Immunol. 13, 707–712. doi: 10.1038/ni.2343
Kasubuchi, M., Hasegawa, S., Hiramatsu, T., Ichimura, A., and Kimura, I. (2015). Dietary gut microbial metabolites, short-chain fatty acids, and host metabolic regulation. Nutrients 7, 2839–2849. doi: 10.3390/nu7042839
Katz, L. M., Young, A., Frank, J. E., Wang, Y., and Park, K. (2004). Neurotensin-induced hypothermia improves neurologic outcome after hypoxic-ischemia*. Crit. Care Med. 32, 806–810. doi: 10.1097/01.CCM.0000114998.00860.FD
Kawabata, F., Inoue, N., Masamoto, Y., Matsumura, S., Kimura, W., Kadowaki, M., et al. (2009). Non-pungent capsaicin analogs (capsinoids) increase metabolic rate and enhance thermogenesis via gastrointestinal TRPV1 in mice. Biosci. Biotechnol. Biochem. 73, 2690–2697. doi: 10.1271/bbb.90555
Kazumori, H., Ishihara, S., Kawashima, K., Fukuda, R., Chiba, T., and Kinoshita, Y. (2001). Analysis of gastrin receptor gene expression in proliferating cells in the neck zone of gastric fundic glands using laser capture microdissection. FEBS Lett. 489, 208–214. doi: 10.1016/S0014-5793(01)02084-1
Kentish, S. J., and Page, A. J. (2015). The role of gastrointestinal vagal afferent fibres in obesity. J. Physiol. 593, 775–786. doi: 10.1113/jphysiol.2014.278226
Khandekar, N., Berning, B. A., Sainsbury, A., and Lin, S. (2015). The role of pancreatic polypeptide in the regulation of energy homeostasis. Mol. Cell. Endocrinol. 418, (Part 1), 33–41. doi: 10.1016/j.mce.2015.06.028
Kim, G. W., Lin, J. E., Valentino, M. A., Colon-Gonzalez, F., and Waldman, S. A. (2011). Regulation of appetite to treat obesity. Expert Rev. Clin. Pharmacol. 4, 243–259. doi: 10.1586/ecp.11.3
Kleinridders, A., Ferris, H. A., Cai, W., and Kahn, C. R. (2014). Insulin action in brain regulates systemic metabolism and brain function. Diabetes 63, 2232–2243. doi: 10.2337/db14-0568
Klovaite, J., Benn, M., and Nordestgaard, B. G. (2015). Obesity as a causal risk factor for deep venous thrombosis: a Mendelian randomization study. J. Intern. Med. 277, 573–584. doi: 10.1111/joim.12299
Koda, S., Date, Y., Murakami, N., Shimbara, T., Hanada, T., Toshinai, K., et al. (2005). The role of the vagal nerve in peripheral PYY3–36-induced feeding reduction in rats. Endocrinology 146, 2369–2375. doi: 10.1210/en.2004-1266
Kohno, D., and Yada, T. (2012). Arcuate NPY neurons sense and integrate peripheral metabolic signals to control feeding. Neuropeptides 46, 315–319. doi: 10.1016/j.npep.2012.09.004
Kojima, M., Hosoda, H., Date, Y., Nakazato, M., Matsuo, H., and Kangawa, K. (1999). Ghrelin is a growth-hormone-releasing acylated peptide from stomach. Nature 402, 656–660. doi: 10.1038/45230
Kopin, A. S., Mathes, W. F., McBride, E. W., Nguyen, M., Al-Haider, W., Schmitz, F., et al. (1999). The cholecystokinin-A receptor mediates inhibition of food intake yet is not essential for the maintenance of body weight. J. Clin. Invest. 103, 383–391. doi: 10.1172/JCI4901
Korner, J., Bessler, M., Cirilo, L. J., Conwell, I. M., Daud, A., Restuccia, N. L., et al. (2005). Effects of Roux-en-Y gastric bypass surgery on fasting and postprandial concentrations of plasma ghrelin, peptide, YY, and Insulin. J. Clin. Endocrinol. Metab. 90, 359–365. doi: 10.1210/jc.2004-1076
Korner, J., Inabnet, W., Conwell, I. M., Taveras, C., Daud, A., Olivero-Rivera, L., et al. (2006). Differential effects of gastric bypass and banding on circulating gut hormone and leptin levels. Obesity 14, 1553–1561. doi: 10.1038/oby.2006.179
Kuhre, R. E., Wewer Albrechtsen, N. J., Hartmann, B., Deacon, C. F., and Holst, J. J. (2015). Measurement of the incretin hormones: glucagon-like peptide-1 and glucose-dependent insulinotropic peptide. J. Diabetes Complicat. 29, 445–450. doi: 10.1016/j.jdiacomp.2014.12.006
Laferrère, B., Teixeira, J., McGinty, J., Tran, H., Egger, J. R., Colarusso, A., et al. (2008). Effect of weight loss by gastric bypass surgery versus hypocaloric diet on glucose and incretin levels in patients with type 2 diabetes. J. Clin. Endocrinol. Metab. 93, 2479–2485. doi: 10.1210/jc.2007-2851
Lam, D. D., Przydzial, M. J., Ridley, S. H., Yeo, G. S. H., Rochford, J. J., O'Rahilly, S., et al. (2008). Serotonin 5-HT2C receptor agonist promotes hypophagia via downstream activation of melanocortin 4 receptors. Endocrinology 149, 1323–1328. doi: 10.1210/en.2007-1321
Larsen, P. J., Tang-Christensen, M., and Jessop, D. S. (1997). Central administration of glucagon-like peptide-1 activates hypothalamic neuroendocrine neurons in the rat*. Endocrinology 138, 4445–4455. doi: 10.1210/endo.138.10.5270
Lassmann, V., Vague, P., Vialettes, B., and Simon, M.-C. (1980). Low plasma levels of pancreatic polypeptide in obesity. Diabetes 29, 428–430. doi: 10.2337/diab.29.6.428
le Roux, C. W., Batterham, R. L., Aylwin, S. J. B., Patterson, M., Borg, C. M., Wynne, K. J., et al. (2006). Attenuated peptide YY release in obese subjects is associated with reduced satiety. Endocrinology 147, 3–8. doi: 10.1210/en.2005-0972
Ley, R. E., Turnbaugh, P. J., Klein, S., and Gordon, J. I. (2006). Microbial ecology: human gut microbes associated with obesity. Nature 444:1022. doi: 10.1038/4441022a
Li, B., Shao, D., Luo, Y., Wang, P., Liu, C., Zhang, X., et al. (2015). Role of 5-HT3 receptor on food intake in fed and fasted mice. PLoS ONE 10:e0121473. doi: 10.1371/journal.pone.0121473
Li, J., Song, J., Zaytseva, Y. Y., Liu, Y., Rychahou, P., Jiang, K., et al. (2016). An obligatory role for neurotensin in high-fat-diet-induced obesity. Nature 533, 411–415. doi: 10.1038/nature17662
Li, M., Luo, X., Chen, L., Zhang, J., Hu, J., and Lu, B. (2003). Co-localization of histamine and dopamine-β-hydroxylase in sympathetic ganglion and release of histamine from cardiac sympathetic terminals of guinea-pig. Autonomic Autacoid Pharmacol. 23, 327–333. doi: 10.1111/j.1474-8673.2004.00305.x
Li, Y., Perry, T., Kindy, M. S., Harvey, B. K., Tweedie, D., Holloway, H. W., et al. (2009). GLP-1 receptor stimulation preserves primary cortical and dopaminergic neurons in cellular and rodent models of stroke and Parkinsonism. Proc. Natl. Acad. Sci. U.S.A. 106, 1285–1290. doi: 10.1073/pnas.0806720106
Lieverse, R. J., Jansen, J. B., Masclee, A. A., and Lamers, C. B. (1995). Satiety effects of a physiological dose of cholecystokinin in humans. Gut 36, 176–179. doi: 10.1136/gut.36.2.176
Lin, H. V., Frassetto, A., Kowalik, E. J. Jr, Nawrocki, A. R., Lu, M. M., Kosinski, J. R., et al. (2012). Butyrate and propionate protect against diet-induced obesity and regulate gut hormones via free fatty acid receptor 3-independent mechanisms. PLoS ONE 7:e35240. doi: 10.1371/journal.pone.0035240
Lin, S., Shi, Y.-C., Yulyaningsih, E., Aljanova, A., Zhang, L., Macia, L., et al. (2009). Critical role of arcuate Y4 receptors and the melanocortin system in pancreatic polypeptide-induced reduction in food intake in mice. PLoS ONE 4:e8488. doi: 10.1371/journal.pone.0008488
Liou, A. P., Paziuk, M., Luevano, J.-M., Machineni, S., Turnbaugh, P. J., and Kaplan, L. M. (2013). Conserved shifts in the gut microbiota due to gastric bypass reduce host weight and adiposity. Sci. Transl. Med. 5:178ra41. doi: 10.1126/scitranslmed.3005687
Liu, Q., Zhang, J., Zerbinatti, C., Zhan, Y., Kolber, B. J., Herz, J., et al. (2011). Lipoprotein receptor LRP1 regulates leptin signaling and energy homeostasis in the adult central nervous system. PLoS Biol. 9:e1000575. doi: 10.1371/journal.pbio.1000575
Lo, C. C., Sean Davidson, W., Hibbard, S. K., Georgievsky, M., Lee, A., Tso, P., et al. (2014). Intraperitoneal CCK, and fourth-intraventricular Apo AIV require both peripheral and NTS CCK1R to reduce food intake in male rats. Endocrinology 155, 1700–1707. doi: 10.1210/en.2013-1846
Löffler, H., Aramaki, J. U. N., and Effendy, I. (2002). The influence of body mass index on skin susceptibility to sodium lauryl sulphate. Skin Res. Technol. 8, 19–22. doi: 10.1046/j.0909-752x
Ludy, M.-J., Moore, G. E., and Mattes, R. D. (2011). The effects of capsaicin and capsiate on energy balance: critical review and meta-analyses of studies in humans. Chemical Senses. 152, 103–121. doi: 10.1093/chemse/bjr100
Mackie, K. (2008). Cannabinoid receptors: where they are and what they do. J. Neuroendocrinol. 20, 10–14. doi: 10.1111/j.1365-2826.2008.01671.x
Maida, A., Lovshin, J. A., Baggio, L. L., and Drucker, D. J. (2008). The glucagon-like peptide-1 receptor agonist oxyntomodulin enhances β-cell function but does not inhibit gastric emptying in mice. Endocrinology 149, 5670–5678. doi: 10.1210/en.2008-0336
Mayer, E. A., Tillisch, K., and Gupta, A. (2015). Gut/brain axis and the microbiota. J. Clin. Invest. 125, 926–938. doi: 10.1172/JCI76304
McAdams DeMarco, M. A., Maynard, J. W., Huizinga, M. M., Baer, A. N., Köttgen, A., Gelber, A. C., et al. (2011). Obesity and younger age at gout onset in a community-based cohort. Arthritis Care Res. 63, 1108–1114. doi: 10.1002/acr.20479
Meek, C. L., Lewis, H. B., Reimann, F., Gribble, F. M., and Park, A. J. (2016). The effect of bariatric surgery on gastrointestinal and pancreatic peptide hormones. Peptides 77, 28–37. doi: 10.1016/j.peptides.2015.08.013
Meier, J. J., Hücking, K., Holst, J. J., Deacon, C. F., Schmiegel, W. H., and Nauck, M. A. (2001). Reduced insulinotropic effect of gastric inhibitory polypeptide in first-degree relatives of patients with type 2 diabetes. Diabetes 50, 2497–2504. doi: 10.2337/diabetes.50.11.2497
Melanson, E. L. (2017). The effect of exercise on non-exercise physical activity and sedentary behavior in adults. Obesity Rev. 18, 40–49. doi: 10.1111/obr.12507
Mentlein, R. (2009). Mechanisms underlying the rapid degradation and elimination of the incretin hormones GLP-1 and GIP. Best Pract. Res. Clin. Endocrinol. Metab. 23, 443–452. doi: 10.1016/j.beem.2009.03.005
Mieczkowska, A., Irwin, N., Flatt, P. R., Chappard, D., and Mabilleau, G. (2013). Glucose-dependent insulinotropic polypeptide (GIP) receptor deletion leads to reduced bone strength and quality. Bone 56, 337–342. doi: 10.1016/j.bone.2013.07.003
Molenaar, E. A., Numans, M. E., van Ameijden, E. J., and Grobbee, D. E. (2008). Considerable comorbidity in overweight adults: results from the Utrecht Health Project. Netherlands J. Med. 152, 2457–2463.
Monje, M. (2017). Settling a nervous stomach: the neural regulation of enteric cancer. Cancer Cell 31, 1–2. doi: 10.1016/j.ccell.2016.12.008
Mönnikes, H., Lauer, G., Bauer, C., Tebbe, J., Zittel, T. T., and Arnold, R. (1997). Pathways of Fos expression in locus ceruleus, dorsal vagal complex, and PVN in response to intestinal lipid. Am. J. Physiol. Regul. Integr. Compar. Physiol. 273, R2059–R2071. doi: 10.1152/ajpregu.1997.273.6.R2059
Moran, C. P., and Shanahan, F. (2014). Gut microbiota and obesity: role in aetiology and potential therapeutic target. Best Pract. Res. Clin. Gastroenterol. 28, 585–597. doi: 10.1016/j.bpg.2014.07.005
Muccioli, G. G., Naslain, D., Bäckhed, F., Reigstad, C. S., Lambert, D. M., Delzenne, N. M., et al. (2010). The endocannabinoid system links gut microbiota to adipogenesis. Mol. Syst. Biol. 6:392. doi: 10.1038/msb.2010.46
Mumphrey, M. B., Patterson, L. M., Zheng, H., and Berthoud, H. R. (2013). Roux-en-Y gastric bypass surgery increases number but not density of CCK-, GLP-1-, 5-HT-, and neurotensin-expressing enteroendocrine cells in rats. Neurogastroenterol. Motility 25, e70–e79. doi: 10.1111/nmo.12034
Munkhaugen, J., Lydersen, S., Widerøe, T.-E., and Hallan, S. (2009). Prehypertension, obesity, and risk of kidney disease: 20-year follow-up of the HUNT I study in Norway. Am. J. Kidney Dis. 54, 638–646. doi: 10.1053/j.ajkd.2009.03.023
Münzberg, H., Laque, A., Yu, S., Rezai-Zadeh, K., and Berthoud, H. R. (2015). Appetite and body weight regulation after bariatric surgery. Obesity Rev. 16, 77–90. doi: 10.1111/obr.12258
Nakhate, K. T., Kokare, D. M., Singru, P. S., and Subhedar, N. K. (2011). Central regulation of feeding behavior during social isolation of rat: evidence for the role of endogenous CART system. Int. J. Obes. 35, 773–784. doi: 10.1038/ijo.2010.231
Neufeld, K. M., Kang, N., Bienenstock, J., and Foster, J. A. (2011). Reduced anxiety-like behavior and central neurochemical change in germ-free mice. Neurogastroenterol. Motility 23, 255–264. doi: 10.1111/j.1365-2982.2010.01620.x
Neyrinck, A. M., Van Hee, V. F., Piront, N., De Backer, F., Toussaint, O., Cani, P. D., et al. (2012). Wheat-derived arabinoxylan oligosaccharides with prebiotic effect increase satietogenic gut peptides and reduce metabolic endotoxemia in diet-induced obese mice. Nutr. Diabetes 2:e28. doi: 10.1038/nutd.2011.24
Nøhr, M. K., Egerod, K. L., Christiansen, S. H., Gille, A., Offermanns, S., Schwartz, T. W., et al. (2015). Expression of the short chain fatty acid receptor GPR41/FFAR3 in autonomic and somatic sensory ganglia. Neuroscience 290, 126–137. doi: 10.1016/j.neuroscience.2015.01.040
Nørsett, K. G., Lægreid, A., Kusnierczyk, W., Langaas, M., Ylving, S., Fossmark, R., et al. (2008). Changes in gene expression of gastric mucosa during therapeutic acid inhibition. Eur. J. Gastroenterol. Hepatol. 20, 613–623. doi: 10.1097/MEG.0b013e3282f5dc19
Nørsett, K. G., Steele, I., Duval, C., Sammut, S. J., Murugesan, S. V. M., Kenny, S., et al. (2011). Gastrin stimulates expression of plasminogen activator inhibitor-1 in gastric epithelial cells. Am. J. Physiol. 301, G446–G4453. doi: 10.1152/ajpgi.00527.2010
O'Donnell, D. E., Ciavaglia, C. E., and Neder, J. A. (2014). When obesity and chronic obstructive pulmonary disease collide. physiological and clinical consequences. Ann. Am. Thorac. Soc. 11, 635–644. doi: 10.1513/AnnalsATS.201312-438FR
Oesch, S., Rüegg, C., Fischer, B., Degen, L., and Beglinger, C. (2006). Effect of gastric distension prior to eating on food intake and feelings of satiety in humans. Physiol. Behav. 87, 903–910. doi: 10.1016/j.physbeh.2006.02.003
Oh, D. Y., Talukdar, S., Bae, E. J., Imamura, T., Morinaga, H., Fan, W., et al. (2010). GPR120 is an omega-3 fatty acid receptor mediating potent anti-inflammatory and insulin-sensitizing effects. Cell 142, 687–698. doi: 10.1016/j.cell.2010.07.041
Ohlsson, L., Kohan, A. B., Tso, P., and Ahrén, B. (2014). GLP-1 released to the mesenteric lymph duct in mice: effects of glucose and fat. Regul. Pept. 189, 40–45. doi: 10.1016/j.regpep.2014.02.001
Ollmann, M. M., Wilson, B. D., Yang, Y.-K., Kerns, J. A., Chen, Y., Gantz, I., et al. (1997). Antagonism of central melanocortin receptors in vitro and in vivo by Agouti-related protein. Science 278, 135–138. doi: 10.1126/science.278.5335.135
Olshansky, S. J., Passaro, D. J., Hershow, R. C., Layden, J., Carnes, B. A., Brody, J., et al. (2005). A potential decline in life expectancy in the United States in the 21st Century. N. Engl. J. Med. 352, 1138–1145. doi: 10.1056/NEJMsr043743
Onakpoya, I. J., Heneghan, C. J., and Aronson, J. K. (2016). Post-marketing withdrawal of anti-obesity medicinal products because of adverse drug reactions: a systematic review. BMC Med. 14:191. doi: 10.1186/s12916-016-0735-y
Ono, K., Tsukamoto-Yasui, M., Hara-Kimura, Y., Inoue, N., Nogusa, Y., Okabe, Y., et al. (2011). Intragastric administration of capsiate, a transient receptor potential channel agonist, triggers thermogenic sympathetic responses. J. Appl. Physiol. 110, 789–798. doi: 10.1152/japplphysiol.00128.2010
Ortega, F. J., Moreno-Navarrete, J. M., Sabater, M., Ricart, W., Frühbeck, G., and Fernández-Real, J. M. (2011). Circulating glucagon is associated with inflammatory mediators in metabolically compromised subjects. Eur. J. Endocrinol. 165, 639–645. doi: 10.1530/EJE-11-0384
Osto, M., Abegg, K., Bueter, M., le Roux, C. W., Cani, P. D., and Lutz, T. A. (2013). Roux-en-Y gastric bypass surgery in rats alters gut microbiota profile along the intestine. Physiol. Behav. 119, 92–96. doi: 10.1016/j.physbeh.2013.06.008
Owen Bryn, M., Ding, X., Morgan Donald, A., Coate Katie, C., Bookout Angie, L., Rahmouni, K., et al. (2014). FGF21 acts centrally to induce sympathetic nerve activity, energy expenditure, and weight loss. Cell Metab. 20, 670–677. doi: 10.1016/j.cmet.2014.07.012
Palamiuc, L., Noble, T., Witham, E., Ratanpal, H., Vaughan, M., and Srinivasan, S. (2017). A tachykinin-like neuroendocrine signalling axis couples central serotonin action and nutrient sensing with peripheral lipid metabolism. Nat. Commun. 8:14237. doi: 10.1038/ncomms14237
Pan, H., Guo, J., and Su, Z. (2014). Advances in understanding the interrelations between leptin resistance and obesity. Physiol. Behav. 130, 157–169. doi: 10.1016/j.physbeh.2014.04.003
Pan, X.-D., and Chen, F.-Q, Wu, T.-X, Tang, H.-G, Zhao, Z.-Y. (2009). Prebiotic oligosaccharides change the concentrations of short-chain fatty acids and the microbial population of mouse bowel. J. Zhejiang Univ. Sci. B. 10, 258–263. doi: 10.1631/jzus.B0820261
Panchal, S. K., Poudyal, H., Waanders, J., and Brown, L. (2012). Coffee extract attenuates changes in cardiovascular and hepatic structure and function without decreasing obesity in high-carbohydrate, high-fat diet-fed male rats. J. Nutr. 142, 690–697. doi: 10.3945/jn.111.153577
Panchal, S. K., Ward, L., and Brown, L. (2013). Ellagic acid attenuates high-carbohydrate, high-fat diet-induced metabolic syndrome in rats. Eur. J. Nutr. 52, 559–568. doi: 10.1007/s00394-012-0358-9
Pankov, Y. A. (2016). Adipogenic function and other biologic effects of insulin. Biomed. Khim. 62, 5–13. doi: 10.18097/pbmc20166201005
Panula, P., Chazot, P. L., Cowart, M., Gutzmer, R., Leurs, R., Liu, W. L. S., et al. (2015). International union of basic and clinical pharmacology. XCVIII. Histamine receptors. Pharmacol. Rev. 67, 601–655. doi: 10.1124/pr.114.010249
Parker, R. M., and Herzog, H. (1999). Regional distribution of Y-receptor subtype mRNAs in rat brain. Eur. J. Neurosci. 11, 1431–1448. doi: 10.1046/j.1460-9568.1999.00553.x
Parmentier, R., Ohtsu, H., Djebbara-Hannas, Z., Valatx, J.-L., Watanabe, T., and Lin, J.-S. (2002). Anatomical, physiological, and pharmacological characteristics of histidine decarboxylase knock-out mice: evidence for the role of brain histamine in behavioral and sleep–wake control. J. Neurosci. 22, 7695–7711. doi: 10.1523/JNEUROSCI.22-17-07695.2002
Pestana, I. A., Greenfield, J. M., Walsh, M., Donatucci, C. F., and Erdmann, D. (2009). Management of “buried” penis in adulthood: an overview. Plast. Reconstr. Surg. 124, 1186–1195. doi: 10.1097/PRS.0b013e3181b5a37f
Phillips, R. J., and Powley, T. L. (1996). Gastric volume rather than nutrient content inhibits food intake. Am. J. Physiol. Regul. Integr. Compar. Physiol. 271, R766–R769. doi: 10.1152/ajpregu.1996.271.3.R766
Pinto-Sanchez, M. I., Hall, G. B., Ghajar, K., Nardelli, A., Bolino, C., Lau, J. T., et al. (2017). Probiotic bifidobacterium longum NCC3001 reduces depression scores and alters brain activity: a pilot study in patients with irritable bowel syndrome. Gastroenterology 153, 448–459.e8. doi: 10.1053/j.gastro.2017.05.003
Pocai, A. (2014). Action and therapeutic potential of oxyntomodulin. Mol. Metab. 3, 241–251. doi: 10.1016/j.molmet.2013.12.001
Pocai, A., Carrington, P. E., Adams, J. R., Wright, M., Eiermann, G., Zhu, L., et al. (2009). Glucagon-like peptide 1/glucagon receptor dual agonism reverses obesity in mice. Diabetes 58, 2258–2266. doi: 10.2337/db09-0278
Polednak, A. P. (2008). Estimating the number of U.S. incident cancers attributable to obesity and the impact on temporal trends in incidence rates for obesity-related cancers. Cancer Detect. Prev. 32, 190–199. doi: 10.1016/j.cdp.2008.08.004
Powell, B. M. (1976). Participation of H1 and H2 histamine receptors in physiological vasodilator responses. Am. J. Physiol. 231, 1002–1009. doi: 10.1152/ajplegacy.1976.231.4.1002
Prechtl, J. C., and Powley, T. L. (1990). The fiber composition of the abdominal vagus of the rat. Anat. Embryol. 181, 101–115. doi: 10.1007/BF00198950
Psichas, A., Reimann, F., and Gribble, F. M. (2015). Gut chemosensing mechanisms. J. Clin. Invest. 125, 908–917. doi: 10.1172/JCI76309
Puente, N., Cui, Y., Lassalle, O., Lafourcade, M., Georges, F., Venance, L., et al. (2011). Polymodal activation of the endocannabinoid system in the extended amygdala. Nat. Neurosci. 14, 1542–1547. doi: 10.1038/nn.2974
Pyarokhil, A. H., Ishihara, M., Sasaki, M., and Kitamura, N. (2012). Immunohistochemical study on the ontogenetic development of the regional distribution of peptide YY, pancreatic polypeptide, and glucagon-like peptide 1 endocrine cells in bovine gastrointestinal tract. Regul. Peptides 175, 15–20. doi: 10.1016/j.regpep.2011.12.004
Qin, J., Li, R., Raes, J., Arumugam, M., Burgdorf, K. S., Manichanh, C., et al. (2010). A human gut microbial gene catalogue established by metagenomic sequencing. Nature 464, 59–65. doi: 10.1038/nature08821
Rahat-Rozenbloom, S., Fernandes, J., Gloor, G. B., and Wolever, T. M. S. (2014). Evidence for greater production of colonic short-chain fatty acids in overweight than lean humans. Int. J. Obes. 38, 1525–1531. doi: 10.1038/ijo.2014.46
Rasmussen, B. A., Breen, D. M., Luo, P., Cheung, G. W. C., Yang, C. S., Sun, B., et al. (2012). Duodenal activation of cAMP-dependent protein kinase induces vagal afferent firing and lowers glucose production in rats. Gastroenterology 142, 834.e3–843.e3. doi: 10.1053/j.gastro.2011.12.053
Ratner, R. E., Dickey, R., Fineman, M., Maggs, D. G., Shen, L., Strobel, S. A., et al. (2004). Amylin replacement with pramlintide as an adjunct to insulin therapy improves long-term glycaemic and weight control in Type 1 diabetes mellitus: a 1-year, randomized controlled trial. Diabetic Medicine 21, 1204–1212. doi: 10.1111/j.1464-5491.2004.01319.x
Ravinet Trillou, C., Delgorge, C., Menet, C., Arnone, M., and Soubrie, P. (2004). CB1 cannabinoid receptor knockout in mice leads to leanness, resistance to diet-induced obesity and enhanced leptin sensitivity. Int. J. Obes. Relat. Metab. Disord. 28, 640–648. doi: 10.1038/sj.ijo.0802583
Reidelberger, R. D., Kalogeris, T. J., Leung, P. M., and Mendel, V. E. (1983). Postgastric satiety in the sham-feeding rat. Am. J. Physiol. Regul. Integr. Comparat. Physiol. 244, R872–R881. doi: 10.1152/ajpregu.1983.244.6.R872
Richards, P., Parker, H. E., Adriaenssens, A. E., Hodgson, J. M., Cork, S. C., Trapp, S., et al. (2014). Identification and characterization of GLP-1 receptor–expressing cells using a new transgenic mouse model. Diabetes 63, 1224–1233. doi: 10.2337/db13-1440
Ritter, R. C. (2011). A tale of two endings: modulation of satiation by NMDA receptors on or near central and peripheral vagal afferent terminals. Physiol. Behav. 105, 94–99. doi: 10.1016/j.physbeh.2011.02.042
Rowland, K. J., Trivedi, S., Lee, D., Wan, K., Kulkarni, R. N., Holzenberger, M., et al. (2011). Loss of glucagon-like peptide-2–induced proliferation following intestinal epithelial insulin-like growth factor-1–receptor deletion. Gastroenterology 141, 2166–2175.e7. doi: 10.1053/j.gastro.2011.09.014
Rüttimann, E. B., Arnold, M., Hillebrand, J. J., Geary, N., and Langhans, W. (2009). Intrameal hepatic portal and intraperitoneal infusions of glucagon-like peptide-1 reduce spontaneous meal size in the rat via different mechanisms. Endocrinology 150, 1174–1181. doi: 10.1210/en.2008-1221
Sakata, I., and Sakai, T. (2010). Ghrelin cells in the gastrointestinal tract. Int. J. Pept. 2010:945056. doi: 10.1155/2010/945056
Sanchez, M., Darimont, C., Drapeau, V., Emady-Azar, S., Lepage, M., Rezzonico, E., et al. (2014). Effect of Lactobacillus rhamnosus CGMCC1.3724 supplementation on weight loss and maintenance in obese men and women. Br. J. Nutr. 111, 1507–1519. doi: 10.1017/S0007114513003875
Sanchez, M., Darimont, C., Panahi, S., Drapeau, V., Marette, A., Taylor, V., et al. (2017). Effects of a diet-based weight-reducing program with probiotic supplementation on satiety efficiency, eating behaviour traits, and psychosocial behaviours in obese individuals. Nutrients 9:284. doi: 10.3390/nu9030284
Sánchez-Villegas, A., Ruíz-Canela, M., Gea, A., Lahortiga, F., and Martínez-González, M. A. (2016). The association between the mediterranean lifestyle and depression. Clin. Psychol. Sci. 4, 1085–1093. doi: 10.1177/2167702616638651
Santo, M. A., Riccioppo, D., Pajecki, D., Kawamoto, F., de Cleva, R., Antonangelo, L., et al. (2016). Weight regain after gastric bypass: influence of gut hormones. Obes. Surg. 26, 919–925. doi: 10.1007/s11695-015-1908-z
Savastano, D. M., Hayes, M. R., and Covasa, M. (2007). Serotonin-type 3 receptors mediate intestinal lipid-induced satiation and Fos-like immunoreactivity in the dorsal hindbrain. Am. J. Physiol. Regul. Integr. Compar. Physiol. 292, R1063–R1070. doi: 10.1152/ajpregu.00699.2006
Sayegh, A. I., Covasa, M., and Ritter, R. C. (2004). Intestinal infusions of oleate and glucose activate distinct enteric neurons in the rat. Autonomic Neurosci. 115, 54–63. doi: 10.1016/j.autneu.2004.08.006
Schéle, E., Grahnemo, L., Anesten, F., Hallén, A., Bäckhed, F., and Jansson, J.-O. (2013). The gut microbiota reduces leptin sensitivity and the expression of the obesity-suppressing neuropeptides proglucagon (Gcg) and brain-derived neurotrophic factor (Bdnf) in the central nervous system. Endocrinology 154, 3643–3651. doi: 10.1210/en.2012-2151
Schéle, E., Grahnemo, L., Anesten, F., Hallén, A., Bäckhed, F., and Jansson, J.-O. (2016). Regulation of body fat mass by the gut microbiota: possible mediation by the brain. Peptides 77, 54–59. doi: 10.1016/j.peptides.2015.03.027
Schwartz, M. W., Woods, S. C., Porte, D., Seeley, R. J., and Baskin, D. G. (2000). Central nervous system control of food intake. Nature 404, 661–671. doi: 10.1038/35007534
Schwartz, T. W., Holst, J. J., Fahrenkrug, J., Jensen, S. L., Nielsen, O. V., Rehfeld, J. F., et al. (1978). Vagal, cholinergic regulation of pancreatic polypeptide secretion. J. Clin. Invest. 61, 781–789. doi: 10.1172/JCI108992
Schwiertz, A., Taras, D., Schäfer, K., Beijer, S., Bos, N. A., Donus, C., et al. (2010). Microbiota and SCFA in lean and overweight healthy subjects. Obesity 18, 190–195. doi: 10.1038/oby.2009.167
Seeley, R. J., Grill, H. J., and Kaplan, J. M. (1994). Neurological dissociation of gastrointestinal and metabolic contributions to meal size control. Behav. Neurosci. 108, 347–352. doi: 10.1037/0735-7044.108.2.347
Sender, R., Fuchs, S., and Milo, R. (2016a). Are we really vastly outnumbered? Revisiting the ratio of bacterial to host cells in humans. Cell 164, 337–340. doi: 10.1016/j.cell.2016.01.013
Sender, R., Fuchs, S., and Milo, R. (2016b). Revised estimates for the number of human and bacteria cells in the body. PLoS Biol. 14:e1002533. doi: 10.1371/journal.pbio.1002533
Shak, J. R., Roper, J., Perez-Perez, G. I., Tseng, C.H., Francois, F., Gamagaris, Z., et al. (2008). The effect of laparoscopic gastric banding surgery on plasma levels of appetite-control, insulinotropic, and digestive hormones. Obes. Surg. 18, 1089–1096. doi: 10.1007/s11695-008-9454-6
Sheridan, P. A., Paich, H. A., Handy, J., Karlsson, E. A., Hudgens, M. G., Sammon, A. B., et al. (2012). Obesity is associated with impaired immune response to influenza vaccination in humans. Int. J. Obes. 36, 1072–1077. doi: 10.1038/ijo.2011.208
Smith, S. R., Weissman, N. J., Anderson, C. M., Sanchez, M., Chuang, E., Stubbe, S., et al. (2010). Multicenter, placebo-controlled trial of lorcaserin for weight management. New Engl. J. Med. 363, 245–256. doi: 10.1056/NEJMoa0909809
Smits, L. P., Bouter, K. E. C., de Vos, W. M., Borody, T. J., and Nieuwdorp, M. (2013). Therapeutic potential of fecal microbiota transplantation. Gastroenterology 145, 946–953. doi: 10.1053/j.gastro.2013.08.058
Steenbergen, L., Sellaro, R., van Hemert, S., Bosch, J. A., and Colzato, L. S. (2015). A randomized controlled trial to test the effect of multispecies probiotics on cognitive reactivity to sad mood. Brain Behav. Immun. 48, 258–264. doi: 10.1016/j.bbi.2015.04.003
Stein, P. D., Matta, F., and Goldman, J. (2011). Obesity and pulmonary embolism: the mounting evidence of risk and the mortality paradox. Thromb. Res. 128, 518–523. doi: 10.1016/j.thromres.2011.10.019
Steuten, L. M., Creutzberg, E. C., Vrijhoef, H. J., and Wouters, E. F. (2006). COPD as a multicomponent disease: inventory of dyspnoea, underweight, obesity and fat free mass depletion in primary care. Primary Care Respir. J. 15, 84–91. doi: 10.1016/j.pcrj.2005.09.001
Stiedl, O., Pappa, E., Konradsson-Geuken, Å., and Ögren, S. O. (2015). The role of the serotonin receptor subtypes 5-HT(1A) and 5-HT(7) and its interaction in emotional learning and memory. Front. Pharmacol. 6:162. doi: 10.3389/fphar.2015.00162
Stinton, L. M., and Shaffer, E. A. (2012). Epidemiology of gallbladder disease: cholelithiasis and cancer. Gut Liver 6, 172–187. doi: 10.5009/gnl.2012.6.2.172
Stock, S., Leichner, P., Wong, A. C. K., Ghatei, M. A., Kieffer, T. J., Bloom, S. R., et al. (2005). Ghrelin, Peptide, YY glucose-dependent insulinotropic polypeptide, and hunger responses to a mixed meal in anorexic, obese, and control female adolescents. J. Clin. Endocrinol. Metab. 90, 2161–2168. doi: 10.1210/jc.2004-1251
Suzuki, K., Jayasena, C. N., and Bloom, S. R. (2012). Obesity and appetite control. Exp. Diabetes Res. 2012:824305. doi: 10.1155/2012/824305
Svendsen, B., Pedersen, J., Albrechtsen, N. J. W., Hartmann, B., Toräng, S., Rehfeld, J. F., et al. (2015). An analysis of cosecretion and coexpression of gut hormones from male rat proximal and distal small intestine. Endocrinology 156, 847–857. doi: 10.1210/en.2014-1710
Swarbrick, M. M., Stanhope, K. L., Austrheim-Smith, I. T., Van Loan, M. D., Ali, M. R., Wolfe, B. M., et al. (2008). Longitudinal changes in pancreatic and adipocyte hormones following Roux-en-Y gastric bypass surgery. Diabetologia 51, 1901–1911. doi: 10.1007/s00125-008-1118-5
Swartz, T. D., Duca, F., De Wouters, T., Sakar, Y., and Covasa, M. (2012). Up-regulation of intestinal type 1 taste receptor 3 and sodium glucose luminal transporter-1 expression and increased sucrose intake in mice lacking gut microbiota. Br. J. Nutr. 107, 621–630. doi: 10.1017/S0007114511003412
Talsania, T., Anini, Y., Siu, S., Drucker, D. J., and Brubaker, P. L. (2005). Peripheral exendin-4 and peptide YY3–36 synergistically reduce food intake through different mechanisms in mice. Endocrinology 146, 3748–3756. doi: 10.1210/en.2005-0473
Tanida, M., Yamano, T., Maeda, K., Okumura, N., Fukushima, Y., and Nagai, K. (2005). Effects of intraduodenal injection of Lactobacillus johnsonii La1 on renal sympathetic nerve activity and blood pressure in urethane-anesthetized rats. Neurosci. Lett. 389, 109–114. doi: 10.1016/j.neulet.2005.07.036
Tims, S., Derom, C., Jonkers, D. M., Vlietinck, R., Saris, W. H., Kleerebezem, M., et al. (2013). Microbiota conservation and BMI signatures in adult monozygotic twins. ISME J. 7, 707–717. doi: 10.1038/ismej.2012.146
Tolhurst, G., Heffron, H., Lam, Y. S., Parker, H. E., Habib, A. M., Diakogiannaki, E., et al. (2012). Short-chain fatty acids stimulate glucagon-like peptide-1 secretion via the G-Protein–coupled receptor FFAR2. Diabetes 61, 364–371. doi: 10.2337/db11-1019
Tolhurst, P., Lindberg, R., Calder, R., and de Courten, M. (2016). Australia's Health Tracker 2016. Melbourne: The Australian Health Policy.
Tukker, A., Visscher, T. L. S., and Picavet, H. S. J. (2009). Overweight and health problems of the lower extremities: osteoarthritis, pain and disability. Public Health Nutr. 12, 359–368. doi: 10.1017/S1368980008002103
Turnbaugh, P. J., Hamady, M., Yatsunenko, T., Cantarel, B. L., Duncan, A., Ley, R. E., et al. (2009). A core gut microbiome in obese and lean twins. Nature 457, 480–484. doi: 10.1038/nature07540
Valenzuela, J. E., Walsh, J. H., and Isenberg, J. I. (1976). Effect of gastrin on pancreatic enzyme secretion and gallbladder emptying in man. Gastroenterology 71, 409–411.
van der Kooy, D. (1984). Area postrema: site where cholecystokinin acts to decrease food intake. Brain Res. 295, 345–347. doi: 10.1016/0006-8993(84)90982-X
Vaure, C., and Liu, Y. (2014). A comparative review of toll-like receptor 4 expression and functionality in different animal species. Front. Immunol. 5:316. doi: 10.3389/fimmu.2014.00316
Villanueva-Peñacarrillo, M. L., Márquez, L., González, N., Díaz-Miguel, M., and Valverde, I. (2001). Effect of GLP-1 on lipid metabolism in human adipocytes. Horm. Metab. Res. 33, 73–77. doi: 10.1055/s-2001-12428
Vilsbøll, T., Agersø, H., Krarup, T., and Holst, J. J. (2003). Similar elimination rates of glucagon-like peptide-1 in obese type 2 diabetic patients and healthy subjects. J. Clin. Endocrinol. Metab. 88, 220–224. doi: 10.1210/jc.2002-021053
Wang, H.-X., and Wang, Y.-P. (2016). Gut microbiota-brain axis. Chin. Med. J. 129, 2373–2380. doi: 10.4103/0366-6999.190667
Wang, J., Barbuskaite, D., Tozzi, M., Giannuzzo, A., Sørensen, C. E., and Novak, I. (2015). Proton pump inhibitors inhibit pancreatic secretion: role of gastric and non-gastric H+/K+-ATPases. PLoS ONE 10:e0126432. doi: 10.1371/journal.pone.0126432
Wang, P., Yan, Z., Zhong, J., Chen, J., Ni, Y., Li, L., et al. (2012). Transient receptor potential vanilloid 1 activation enhances gut glucagon-like peptide-1 secretion and improves glucose homeostasis. Diabetes 61, 2155–2165. doi: 10.2337/db11-1503
Wilson, P. F., D'Agostino, R. B., Sullivan, L., Parise, H., and Kannel, W. B. (2002). Overweight and obesity as determinants of cardiovascular risk: the framingham experience. Arch. Intern. Med. 162, 1867–1872. doi: 10.1001/archinte.162.16.1867
Wostmann, B. S., Larkin, C., Moriarty, A., and Bruckner-Kardoss, E. (1983). Dietary intake, energy metabolism, and excretory losses of adult male germfree Wistar rats. Lab. Anim. Sci. 33, 46–50.
Writing Group, M., Go, A. S., Mozaffarian, D., Roger, V. L., Benjamin, E. J., Berry, J. D., et al. (2014). Heart disease and stroke statistics-−2014 update: a report from the American Heart Association. Circulation 129, e28–e292. doi: 10.1161/01.cir.0000441139.02102.80
Wynne, K., Park, A. J., Small, C. J., Meeran, K., Ghatei, M. A., Frost, G. S., et al. (2006). Oxyntomodulin increases energy expenditure in addition to decreasing energy intake in overweight and obese humans: a randomised controlled trial. Int. J. Obes. 30, 1729–1736. doi: 10.1038/sj.ijo.0803344
Wynne, K., Park, A. J., Small, C. J., Patterson, M., Ellis, S. M., Murphy, K. G., et al. (2005). Subcutaneous oxyntomodulin reduces body weight in overweight and obese subjects. A double-blind, randomized, controlled trial. Diabetes 54, 2390–2395. doi: 10.2337/diabetes.54.8.2390
Yamato, E., Ikegami, H., Takekawa, K., Fujisawa, T., Nakagawa, Y., Hamada, Y., et al. (1997). Tissue-specific and glucose-dependent expression of receptor genes for glucagon and glucagon-like peptide-1 (GLP-1). Horm. Metab. Res. 29, 56–59. doi: 10.1055/s-2007-978985
Yang, Y.-K., Chen, M., Clements, R. H., Abrams, G. A., Aprahamian, C. J., and Harmon, C. M. (2008). Human mesenteric adipose tissue plays unique role versus subcutaneous and omental fat in obesity related diabetes. Cell. Physiol. Biochem. 22, 531–538. doi: 10.1159/000185527
Yavuz, Y., Kumral, Z. N. Ö., Memi, G., Çevik, Ö. D., Yegen, C., and Yegen, B. Ç. (2017). Serum leptin, obestatin, and ghrelin levels and gastric emptying rates of liquid and solid meals in non-obese rats with roux-en-Y bypass surgery or prosthesis placement: implications for the role of vagal afferents. Obes. Surg. 27, 1037–1046. doi: 10.1007/s11695-016-2420-9
Yosipovitch, G., DeVore, A., and Dawn, A. (2007). Obesity and the skin: skin physiology and skin manifestations of obesity. J. Am. Acad. Dermatol. 56, 901–916. doi: 10.1016/j.jaad.2006.12.004
Yosipovitch, G., Sackett-Lundeen, L., Goon, A., Yiong Huak, C., Leok Goh, C., and Haus, E. (2004). Circadian and ultradian (12 h) variations of skin blood flow and barrier function in non-irritated and irritated skin—effect of topical corticosteroids. J. Investig. Dermatol. 122, 824–829. doi: 10.1111/j.0022-202X.2004.22313.x
Young, R. L., Sutherland, K., Pezos, N., Brierley, S. M., Horowitz, M., Rayner, C. K., et al. (2009). Expression of taste molecules in the upper gastrointestinal tract in humans with and without type 2 diabetes. Gut 58, 337–346. doi: 10.1136/gut.2008.148932
Yuan, X., Yao, P. Y., Jiang, J., Zhang, Y., Su, Z., Yao, W., et al. (2017). MST4 phosphorylates ACAP4 to orchestrate apical membrane remodeling during gastric acid secretion. J. Biol. Chem. 292, 16174–16187. doi: 10.1074/jbc.M117.808212
Zhang, H., DiBaise, J. K., Zuccolo, A., Kudrna, D., Braidotti, M., Yu, Y., et al. (2009). Human gut microbiota in obesity and after gastric bypass. Proc. Natl. Acad. Sci. U.S.A. 106, 2365–2370. doi: 10.1073/pnas.0812600106
Zhang, J., and Ritter, R. C. (2012). Circulating GLP-1 and CCK-8 reduce food intake by capsaicin-insensitive, nonvagal mechanisms. Am. J. Physiol. Regul. Integr. Comparat. Physiol. 302, R264–R273. doi: 10.1152/ajpregu.00114.2011
Zittel, T. T., De Giorgio, R., Sternini, C., and Raybould, H. E. (1994). Fos protein expression in the nucleus of the solitary tract in response to intestinal nutrients in awake rats. Brain Res. 663, 266–270. doi: 10.1016/0006-8993(94)91272-6
Keywords: gut-brain axis, microbiota, cholecystokinin (CCK), glucagon-like peptide 1 (GLP1), peptide YY3−36 (PYY), lipopolysaccharide (LPS), obesity, short-chain fatty-acids (SCFA)
Citation: Bliss ES and Whiteside E (2018) The Gut-Brain Axis, the Human Gut Microbiota and Their Integration in the Development of Obesity. Front. Physiol. 9:900. doi: 10.3389/fphys.2018.00900
Received: 27 February 2018; Accepted: 21 June 2018;
Published: 12 July 2018.
Edited by:
Stephen J. Pandol, Cedars-Sinai Medical Center, United StatesReviewed by:
Georg Singer, Medizinische Universität Graz, AustriaAngelo Tremblay, Laval University, Canada
Copyright © 2018 Bliss and Whiteside. This is an open-access article distributed under the terms of the Creative Commons Attribution License (CC BY). The use, distribution or reproduction in other forums is permitted, provided the original author(s) and the copyright owner(s) are credited and that the original publication in this journal is cited, in accordance with accepted academic practice. No use, distribution or reproduction is permitted which does not comply with these terms.
*Correspondence: Edward S. Bliss, edward.bliss@usq.edu.au