- Marine Bio-Products Research Laboratory, Department of Plant, Food and Environmental Sciences, Faculty of Agriculture, Dalhousie University, Truro, NS, Canada
Phosphorous is one of the major limiting factors determining plant growth. Current agricultural practices mainly rely on the use of chemical fertilizers posing threat to the ecosystem. In this study, the application of an Ascophyllum nodosum extract (ANE) in phosphorous (P)-limited conditions improved the fresh and dry weight of shoots and roots of Zea mays. ANE-treated Z. mays grown under P-limited conditions showed a higher P content than the control. ANE activated simultaneous responses, at multiple levels, in Z. mays grown under P-limited conditions as seen from the regulation of gene expression at the whole-plant level to specific biochemical responses on a subcellular level. ANE-supplemented Z. mays grown under P-limited conditions also showed reduced electrolyte leakage and lipid peroxidation by an improved membrane stability. ANE treatment reduced P-limitation-induced oxidative damage in Z. mays by reducing H2O2 and accumulation. Furthermore, ANE also induced the accumulation of the total contents of soluble sugars, amino acids, phenolics, and flavonoids. Gene expression analysis suggested that ANE differentially modulated the expression of P-starvation responsive genes involved in metabolic, signal transduction, and developmental pathways in Z. mays. ANE also modulated the expression of genes involved in sugar, lipid, and secondary metabolism. Thus, this study illustrated the role of ANE in improving the productivity of Z. mays, an important crop, in P-limited conditions. Furthermore, it sets the framework to increase agricultural productivity in nutrient deficient soils using a sustainable, eco-friendly strategy.
Introduction
Phosphorous (P) is an important macronutrient for plants and constitutes about 0.2% of dry weight (DW) (Schachtman et al., 2002). Phosphorous is a key constituent of cellular biomolecules, such as ATP, NADP, nucleic acids, phospholipids, sugar phosphates, and enzymes (Hernández and Munné-Bosch, 2015; Achary et al., 2017). It plays an important role in nucleic acid and protein synthesis, membrane integrity, photosynthesis, respiration, energy metabolism, hormone regulation, stress tolerance, and disease resistance (Sun et al., 2018). Adequate P availability is necessary for normal plant growth (Vance et al., 2003). Globally, 5.7 billion ha of all the available agricultural land is defined as being deficient in phosphorous due to soil degradation and is a major limiting factor of agricultural productivity (Roch et al., 2019).
Chemical fertilizers are used to enhance the availability of P to plants from the soil (Veneklaas et al., 2012; Heuer et al., 2017). However, the excessive use of chemical fertilizers poses risks to the environment. In addition, the raw material (i.e., rock phosphate) used to manufacture P fertilizer is a finite, natural source, and thus the costs of P fertilizers can only increase (Vance et al., 2003). Attempts have been made to genetically engineer selected plants with a higher P-use efficiency through transformation of genes involved in P acquisition, transportation, and signal transduction (Hammond, 2003; Chiou, 2005). However, P uptake has been found to be a multi-genic trait; limited success had been achieved in generating P-efficient crops (Shenoy and Kalagudi, 2005; George and Richardson, 2008). In the current scenario, there is a need to develop a more sustainable strategy to enhance P-use efficiency in agricultural systems.
Seaweed-derived biostimulants are new class of agricultural input that are being widely researched for the improvement of various nutrient-use efficiencies in plants (Khan et al., 2009; Jannin et al., 2013). Ascophyllum nodosum is a brown, intertidal seaweed, common to the Northern Hemisphere and has been extensively investigated as a source of various commercial biostimulants with the specific aims of improving plant growth and productivity by increasing nutrient availability and uptake (Jannin et al., 2013; Shukla et al., 2019; Pereira et al., 2020). AZAL5 is a commercial extract of A. nodosum and plays a vital role in nitrogen (N), carbon (C), and sulfur (S) metabolism in Brassica napus (Jannin et al., 2013). In the same study, micro-array analysis of B. napus treated with AZAL5 showed differential regulation of genes involved in nitrate assimilation and amino acid metabolism. Another commercial extract of A. nodosum extract (ANE) improved salinity tolerance in Arabidopsis by improving P uptake in salt-stressed plants by regulating the expression of miRNA involved in P homeostasis (Shukla et al., 2018). Applications of ANE also enhanced the macro- and micro-nutrient contents of tomato fruits grown under salinized conditions (Di Stasio et al., 2018). The aim of this study was to elucidate the mechanisms of action of ANE in improving the growth of Zea mays grown under phosphorous (P)-limited conditions.
Materials and Methods
Treatment of Z. mays With Experimentally Induced P-Limiting Conditions
Z. mays seeds (cv. Bilicious) were purchased from Halifax Seed (Halifax, NS, Canada). ANE, commercially available as Acadian Marine Plant Extract (Acadian Seaplants Ltd., Dartmouth, NS, Canada), was used as the seaweed biostimulant. The chemical composition of the extract was described by Rayirath et al. (2009). The P content in the 0.01% of ANE was measured using inductively coupled plasma optical emission spectrometer (ICP-OES) at the Analytical and Dairy Lab, Harlow Institute, Nova Scotia, Canada.
Seeds were sterilized using 2.5% Clorox® and were kept on filter paper moistened with water in a petri dish for germination in the dark. Seven-day-old, uniformly germinated, seedlings were used for further experiments. The experiment was designed to assess the effects of 0.01% ANE on the growth of 7-day-old Z. mays seedlings grown under P-limited conditions for 14 days. This concentration of ANE was selected based on the results reported in previous studies (Shukla et al., 2018; Bajpai et al., 2019; Jithesh et al., 2019). In addition to the control (1/2 MS (Murashige and Skoog media), C), the other three treatments were 1/2 MS + 0.01% ANE (T1), 1/2 MS-P (T2), and 1/2 MS-P + 0.01% ANE (T3). The MS medium without P (MSP19) was procured from Caisson Labs, USA. It was estimated that 0.01% ANE contained 5.0 μM P, which was added to T2 in the form of H3PO4 (Rickard, 2000). The plants treated with different treatments were grown for 14 days in the growth chamber maintained at 24°C with a photoperiod (100 μmol photons/m2/s) of 16/8 h (day/night). Increase in total root length and leaf area of plants was recorded at 14 days after the imposition of P-limited conditions by using ImageJ software. After 14 days under different conditions, fresh weights of shoots and roots were recorded. DW was recorded after drying in an oven at 70°C for 72 h. The data for the morphological parameters were generated using six plants for each experimental condition, and the experiment was triplicated.
Mineral Element Analysis
The nitrogen content and mineral element analysis were done at the Analytical and Dairy Lab, Harlow Institute, Nova Scotia, Canada. The plants grown under different treatments for 14 days were dried at 72°C for 72 h. The nitrogen content in the plant tissue was determined by combustion analysis method using Leco CN828 Carbon Nitrogen Determinator. The mineral elements were measured from the ash dried plant samples with an ICP-OES.
Determination of Pigments
The effect of ANE on chlorophyll a and b and the carotenoid content of Z. mays grown under different treatments for 14 days were determined according to the protocol described by Lichtenthaler (1987). Briefly, 1 g of six individual plant samples was instantaneously ground using a mortar and pestle in 5 ml of cold methanol. Following extraction, the ground mixture was centrifuged at 10,000 rpm at 4°C for 10 min, and the pellet was re-extracted with 10 ml of cold methanol until all color was removed. Two extracts were combined, and total volume was made up to 15 ml. Absorbance was measured at 470, 652.4, and 665.2 nm using a UV–VIS spectrophotometer (Biotek, USA). The chlorophyll contents were calculated according to Lichtenthaler (1987).
Chla = 16.72 A665.2 – 9.16 A652.4
Chlb = 34.09 A652.4 – 15.28 A665.2
Carotenoids = (1,000 A470 – 1.63 Chla – 104.96 Chlb) / 221
Determination of Anthocyanin Content
The effect of ANE on the anthocyanin content of Z. mays grown under different treatments for 14 days was evaluated using the protocol published by Burgos et al. (2013). One gram of plant sample was extracted with 10 ml of methanol and acidified with 1% HCl. The mixture was centrifuged at 5,000 rpm for 10 min at 4°C, and the pellet was re-extracted. Different fractions were combined, and the final extract volume was made up to 25 ml. Absorbance at 545 nm was recorded using a UV–VIS spectrophotometer. The anthocyanin content was calculated using the molar extinction coefficient and molecular weight of malvidin-3-p-coumaroyl-glucoside (i.e., 545 nm, 3.02 × 104 L/mol/cm, 718.5 g/mol).
Determination of Electrolyte Leakage and Membrane Stability Index
Electrolyte leakage was determined as described by Shukla et al. (2012). The youngest, fully expanded leaves were collected from plants grown under different treatments for 14 days. The leaves were thoroughly cleaned with de-ionized water to remove surface-adhered electrolyte. The samples were placed in a closed, glass tube containing 10 ml of de-ionized water. After a 24-h incubation at 25°C on a rotary shaker, electrical conductivity (Lt) was measured (Hanna Instruments, Canada). The samples were autoclaved at 120°C for 20 min and cooled to room temperature to determine the final electrical conductivity (L0). Electrolyte leakage was calculated according to Lutts et al. (1996).
A membrane stability index (MSI) was determined according to the protocol published by Yadav et al. (2012). In this method the youngest, fully expanded leaves were collected from the plants grown under different treatments for 14 days and placed into 10 ml of de-ionized water in two sets, as triplicates. The electrical conductivity (C1) of one set of samples was measured after heating at 40°C for 30 min in a water bath. The second set was boiled at 100°C for 10 min; electrical conductivity (C2) was measured after cooling to room temperature. MSI was calculated using the following formula:
Determination of Lipid Peroxidation
Lipid peroxidation was estimated by determining the concentration of malondialdehyde (MDA) in the leaves of the plants grown under different treatments for 14 days, after Hodges et al. (1999). Leaf material (0.5 g) was homogenized in 15 ml of 80% ethanol (EtOH), followed by centrifugation at 5,000 rpm at 4°C for 10 min. The supernatant (100 μl) and distilled water (900 μl) were mixed in a test tube with 1 ml of either (i) –TBA (thiobarbituric acid) solution including 20% (w/v) trichloroacetic acid (TCA) and 0.01% (w/v) butylated hydroxytoluene (BHT) or (ii) +TBA solution containing 0.65% (w/v) TBA with 20% (w/v) TCA and 0.01% (w/v) BHT. The mixture was vortexed, heated at 95°C in a dry bath for 25 min, cooled, and centrifuged at 5,000 rpm for 10 min. Absorbance was measured at 440, 532, and 600 nm. MDA equivalents were calculated using the following formula:
(1) [(Abs532+TBA) – (Abs600+TBA) – (Abs532−TBA – Abs600−TBA)] = A,
(2) [(Abs440+TBA – Abs600+TBA) 0.0571] = B,
(3) MDA equivalents (nmol/ml) = 106 [(A – B) / 157,000].
In vivo Localization and Quantification of and H2O2
In vivo localization and quantification of and H2O2 were performed by histochemical staining with nitro-blue tetrazolium (NBT) and 3,3′-diaminobenzidine (DAB), as described by Yadav et al. (2012). To stain in the leaves of the Z. mays grown under treatments C, T1, T2, and T3, leaf samples were immersed in 1 mg ml−1 of NBT in 10 mM phosphate buffer. After a 12-h incubation at room temperature, blue colored spots were observed, indicative of formation. Similarly, H2O2 was stained by incubating leaf samples in 1 mg ml−1 of DAB for 24 h. After incubation, brown colored spots were formed as a result of the reaction between DAB and H2O2.
content was estimated in leaves of Z. mays, as described by Liu and Pang (2010). Leaf tissue was homogenized in 10 ml of 65 mM potassium phosphate buffer (pH 7.8) and centrifuged at 5,000 rpm for 10 min. The reaction mixture consisted of 0.9 ml of 65 mM phosphate buffer (pH 7.8) and 0.1 ml of 10 mM hydroxylamine hydrochloride, and 1 ml of the extract was incubated at 25°C for 20 min. Then, 17 mM sulfanilamide and 7 mM α-naphthylamine were added to the extract, the mixture was further incubated at 25°C for 20 min, and absorbance was read at 530 nm using a UV–VIS spectrophotometer. A standard curve (10–200 nmol) was prepared with NaNO2 to calculate the production rate of . The H2O2 content in leaf samples was measured as described by Yadav et al. (2012). Leaf tissue was extracted with cold acetone. Two milliliters of the extract was mixed with 0.5 ml of 0.1% titanium dioxide in 20% (v/v) H2SO4, and the mixture was centrifuged at 6,000 rpm for 15 min. The intensity of yellow color of the supernatant was measured at 415 nm using a UV–VIS spectrophotometer.
Determination of Total Soluble Sugars and Total Amino Acids
The effect of ANE treatments on the total soluble sugars and amino acids of Z. mays was determined according to the protocol described by Irigoyen et al. (1992) and Shukla et al. (2012), respectively. Leaf samples (100 mg) from all the treatments were frozen in liquid nitrogen and homogenized with mortar and pestle. The fine frozen powder was immediately suspended in 5 ml of 80% EtOH. The mixture was centrifuged at 5,000 rpm for 10 min at 4°C, and the pellet was re-extracted. Fractions were combined, and the final volume was made up to 15 ml. The total soluble sugars were analyzed by reacting 100 μl of alcoholic extract with 3 ml of freshly prepared anthrone reagent (150 mg anthrone in 100 ml of 72% (v/v) H2SO4). The mixture was placed in a boiling water bath for 10 min. After the mixture was cooled to room temperature, absorbance at 620 nm was measured. The content of total amino acids was determined by treating 1 ml of alcoholic extract with 1 ml of 0.2 M citrate buffer (pH 5.0), 1 ml of 80% EtOH, and 1 ml of ninhydrin (1%), followed by incubation at 95°C for 15 min. The samples were cooled, and the absorbance at 570 nm was measured.
Determination of Total Phenolics and Flavonoids Contents
For the quantification of secondary metabolites, Z. mays leaves were harvested from different treatments after 14 days. The samples were frozen in liquid nitrogen and homogenized with mortar and pestle. The fine frozen powder was immediately suspended in 70% methanol and centrifuged at 10,000 rpm for 10 min at 4°C. The total phenolics content was determined using Folin-Ciocalteu assay as described by Hodges and Lester (2006). One milliliter of extract was treated with 0.2 N Folin-Ciocalteu phenol reagent and vortexed. After incubation of the mixture for 5 min at room temperature, 1 ml of 7% Na2CO3 solution was added to the mixture. The mixture was incubated for 90 min at room temperature. After incubation, the absorbance against the reagent blank was determined at 550 nm. Total phenolics content was expressed as gallic acid equivalents (mg/g FW).
Total flavonoids content was determined according to Hichem et al. (2009). Briefly, 1 ml of plant extract was added to 4 ml of distilled water. The diluted samples were treated with 0.3 ml of 5% sodium nitrite (NaNO2). After 5 min of incubation at room temperature, 0.3 ml of 10% aluminum chloride was added. Then, after 5 more minutes, 2 ml of 1 M sodium hydroxide (NaOH) was added to the mixture, and the final volume was made up to 10 ml. The solution was vortexed, and absorbance was measured against the reagent blank at 510 nm. The total flavonoids content was expressed as mg quercetin equivalents (QE).
Gene Expression Analysis
To elucidate the role of ANE treatments in improving plant growth under P-limited conditions, real-time expression of genes involved in P homeostasis, carbohydrate metabolism, lipid metabolism, and secondary metabolism were performed. For gene expression analysis, experiments were conducted in a hydroponic system. Seven-day-old seedlings were inserted into a polystyrene disc floated on 1/2 MS (C), 1/2 MS + 0.01% ANE (T1), 1/2 MS-P + 5 μM H3PO4 (T2), and 1/2 MS-P + 0.01% ANE (T3) in 300 ml Phyta jars. Leaf and root samples were harvested after 2 and 7 days of treatment. Total RNA was extracted using the RNAeasy kit (Qiagen, Germany) following the manufacturer's protocol. The quantity and purity of the total RNA were analyzed using a NanoDrop 2000 spectrophotometer (Thermo Scientific, USA). An amount of 2.5 μg RNA from each sample was treated with DNase I (Promega, USA), and then the synthesis of cDNA was performed using the Revert Aid cDNA reverse transcription kit (Thermo Scientific, USA). Real-time quantitative PCR (qPCR) was performed using the StepOnePlus Real-Time PCR system (Applied Biosystems). Actin and β-tubulin were used as reference genes. A list of the different primers used in this study is presented in Supplementary Table 1. The specificity of PCR amplification was checked at the end of the PCR cycles by melt-curve analysis. Each biological sample had three technical replicates, and the relative-fold expression was determined using the Livak (2−ΔΔCt) method (Livak and Schmittgen, 2001).
Statistical Analysis
Each experiment was carried out in triplicate, each experimental unit had six plants, and for each response variable, the average of the 18 values was used for statistical analysis. The results were presented as mean ± standard error. Data were analyzed using ANOVA, with a p ≤ 0.01 using the “Proc. mixed procedure,” of the SAS Institute, Inc. software version 9.3 (SAS Institute, Inc., Cary, NC, USA). When significant effects of treatments were found, multiple means comparison was carried out using Tukey's analysis with a 95% confidence interval. The significantly different mean values were represented by different alphabets.
Results
ANE Improved Growth of Z. mays Grown Under P-Limited Conditions by Regulating Nutrient Homeostasis
Under low P conditions, the shoot and root growth of the Z. mays were significantly reduced, as compared with the control (Figure 1). The addition of ANE in the growth media improved the growth of the plant under P-limited conditions (Figure 1). Leaf area and total root length of the ANE-supplemented plants were significantly higher (p ≤ 0.01) in P-limited conditions than those of the control (Figures 1C,D). ANE-supplemented Z. mays plants grown under P-limited conditions showed higher fresh and DWs of shoots and roots, but not their percentage water content (PWC), than the control (Figure 2). The dry biomass of the shoot and root of ANE-supplemented Z. mays grown under P-limited conditions was found to be 4.33 and 1.29 times, respectively, higher than the plants grown in P-limited conditions alone (Figures 2B,E).
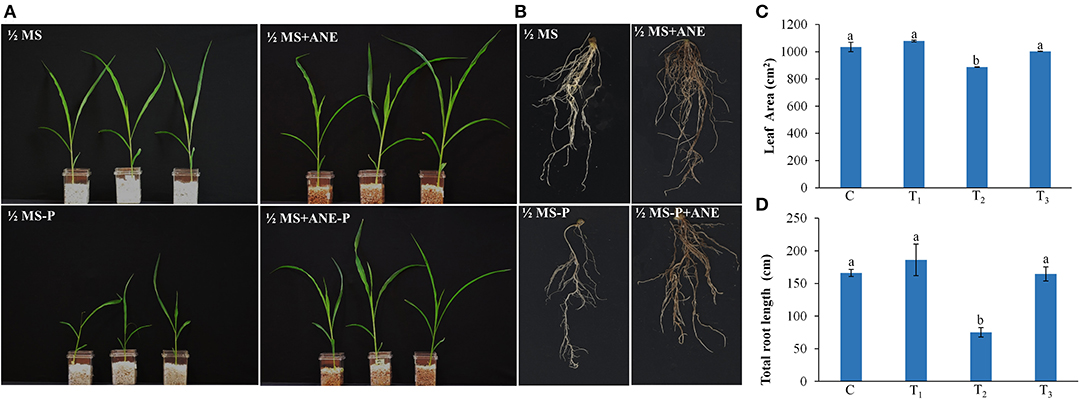
Figure 1. Ascophyllum nodosum extract (ANE) improves the growth of Z. mays grown under P-limiting conditions. Effect of ANE on (A) shoot, (B) root growth, (C) leaf area, and (D) total root length of Z. mays under the P-limiting conditions. Seven-day-old Z. mays seedlings were grown in perlite supplemented with 1/2 MS and 1/2 MS-P for 14 days. C (control): plant grown in perlite supplemented with 1/2 MS, T1: plant grown in perlite supplemented with 1/2 MS + ANE, T2: plant grown in perlite supplemented with 1/2 MS-P, T3: plant grown in perlite supplemented with 1/2 MS-P + ANE. The values were presented as mean ± SE, and means represented by the same letters are not significantly different at p ≤ 0.01. Each experiment was carried out in triplicate, and each experimental unit had six plants (n = 18).
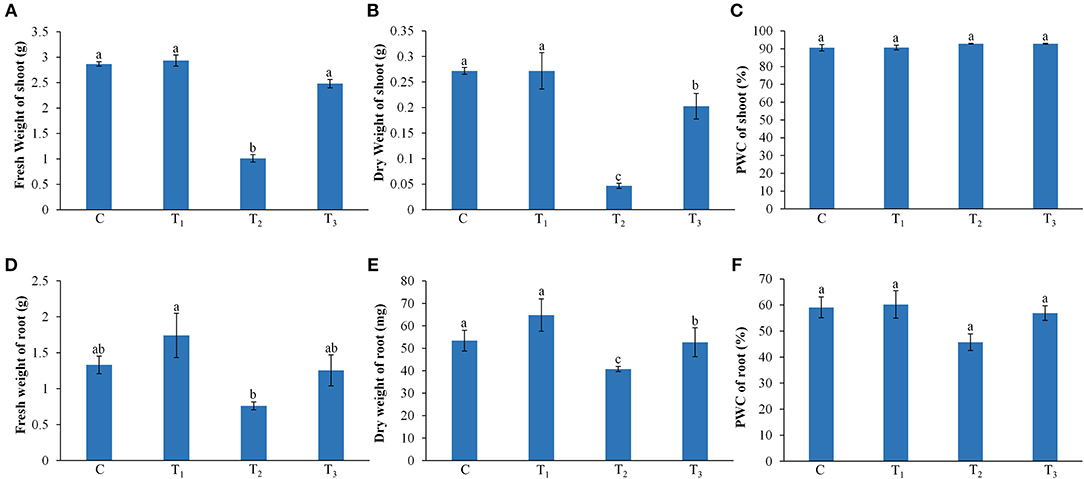
Figure 2. Effect of ANE on fresh weight, dry weight, and percentage water content (PWC) of shoot (A–C) and root (D–F), respectively, of Z. mays grown under P-limiting conditions. C (control): plant grown in perlite supplemented with 1/2 MS, T1: plant grown in perlite supplemented with 1/2 MS + ANE, T2: plant grown in perlite supplemented with 1/2 MS-P, T3: plant grown in perlite supplemented with 1/2 MS-P + ANE. The values were presented as mean ± SE, and means represented by the same letters are not significantly different at p ≤ 0.01. Each experiment was carried out in triplicate, and each experimental unit had six plants (n = 18).
Mineral analysis was carried out on the leaves of the Z. mays plants grown under different treatments [1/2 MS (control, C), 1/2 MS + 0.01% ANE (T1), 1/2 MS-P (T2), and 1/2 MS-P + 0.01% ANE (T3)] (Table 1). No significant differences were observed in the nitrogen (N), phosphorus (P), potassium (K), calcium (Ca), magnesium (Mg), sodium (Na), zinc (Zn), and copper (Cu) contents of those plants grown in 1/2 MS and 1/2 MS + 0.01% ANE. P-limited conditions did not appear to have any influence on the K, Ca, and Na contents of the Z. mays. However, P-limited conditions significantly (p ≤ 0.01) reduced the N content in the plants, whereas ANE supplementation in the P-limited media increased N content by 18.3% in those plants. The application of ANE in the P-limited media significantly increased the P content, suggesting that ANE restored growth of those plants, in P-limited conditions, by regulating P homeostasis. Those plants grown in the presence of 1/2 MS-P had a reduction in Mg content of 30.2% and Zn by 26.25%, as compared with the control, whereas ANE treatments showed significantly less reduction in Mg content by 11.9% and Zn content by 15.3% under P-limited conditions, as compared with the control (Table 1).

Table 1. Mineral content in the leaves of Z. mays grown in the perlite supplemented with 1/2 MS (C), 1/2 MS + ANE (T1), 1/2 MS-P (T2), and 1/2 MS-P + ANE (T3).
P-limited conditions increased the Fe content by 101.6%, as compared with the control, but the increment of Fe content was less (i.e., 74.71%, as compared with the control) in those plants grown in the ANE-supplemented P-limited media (Table 1). The application of ANE significantly increased the uptake of B by 40% in those plants grown under normal conditions. P-limited conditions increased the uptake of B in plants grown in 1/2 MS-P (T2) and 1/2 MS-P + 0.01% ANE (T3), but the addition of ANE in P-limited media significantly increased the B content by 8.0%, as compared with the plants grown in P-limited conditions. ANE treatment increased the uptake of Mn by 7.6 and 11.5% in plants grown under the normal and P-limited conditions, respectively (Table 1).
Effects of ANE Treatment on the Pigment Content of Z. mays Grown Under P-Limited Conditions
ANE treatment showed no significant (p ≤ 0.01) effects on the chlorophyll a and b, carotenoid, and anthocyanin contents of leaves of Z. mays grown under normal conditions, as compared with the control (Figures 3A,B). The P-limited condition reduced the leaf content of chlorophyll a and b, whereas supplementation with ANE significantly increased the content of chlorophyll a and b in P-limited conditions. Carotenoid was also found to be higher in the ANE-treated plants grown in the P-limited media than in the plants grown in P-limited media alone (Figure 3C). The anthocyanin content was found to be increased by 173.1% as a result of the imposed P-limited conditions, whereas ANE-treated plants showed significantly less (98.3%) anthocyanin accumulation in plants grown under P-limited conditions (Figure 3D).
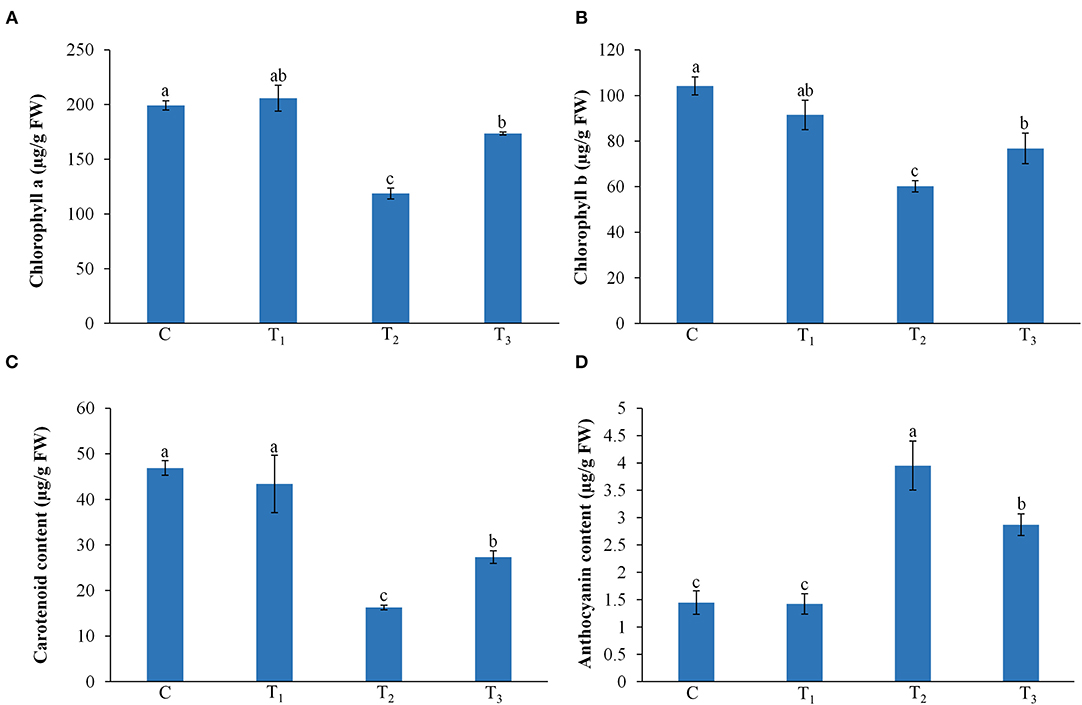
Figure 3. Effect of ANE on (A) chlorophyll a, (B) chlorophyll b, (C) carotenoid, and (D) anthocyanin contents of Z. mays grown under the P-limiting conditions. C (control): plant grown in perlite supplemented with 1/2 MS, T1: plant grown in perlite supplemented with 1/2 MS + ANE, T2: plant grown in perlite supplemented with 1/2 MS-P, T3: plant grown in perlite supplemented with 1/2 MS-P + ANE. The values were presented as mean ± SE, and means represented by the same letters are not significantly different at p ≤ 0.01. Each experiment was carried out in triplicate, and each experimental unit had six plants (n = 18).
Effect of ANE Treatment on Electrolyte Leakage, Membrane Stability, and MDA Contents of Z. mays Grown Under P-Limited Conditions
Z. mays plants treated with ANE showed a significant reduction of electrolyte leakage of leaves of those plants grown under P-limitation (Figure 4A). ANE reduced the electrolyte leakage by 35.3% in the leaves of Z. mays grown under P-limited conditions, as compared with the plants grown under P-limited conditions alone. MSI analysis revealed that the cell membranes of plants grown under P-limited conditions were more stable in those plants supplemented with ANE (Figure 4B). Membrane stability of the plants grown in 1/2 MS-P + 0.01% ANE was 68.2% higher than that of the plants grown in 1/2 MS-P (Figure 4B). To determine the impact of ANE treatment on the membrane lipids during P-limited conditions, the MDA content was measured in leaves of Z. mays grown under different treatments. No significant differences were observed in the MDA content of plants grown in 1/2 MS and 1/2 MS + 0.01% ANE. Compared with those plants grown in 1/2 MS-P, the MDA content was significantly reduced by 57.6% in ANE-supplemented plants, suggesting that lipid peroxidation of membrane lipids was significantly less than those grown in P-limited conditions (Figure 4C).
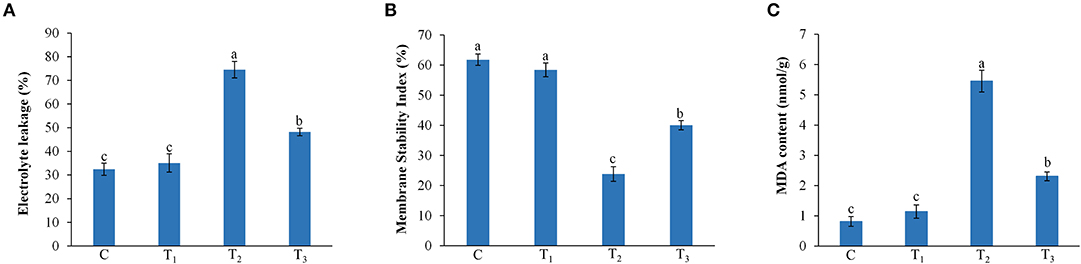
Figure 4. Effect of ANE on (A) electrolyte leakage, (B) membrane stability index, and (C) MDA contents of Z. mays grown under the P-limiting conditions. C (control): plant grown in perlite supplemented with 1/2 MS, T1: plant grown in perlite supplemented with 1/2 MS + ANE, T2: plant grown in perlite supplemented with 1/2 MS-P, T3: plant grown in perlite supplemented with 1/2 MS-P + ANE. The values were presented as mean ± SE, and means represented by the same letters are not significantly different at p ≤ 0.01. Each experiment was carried out in triplicate, and each experimental unit had six plants (n = 18).
ANE Treatment Reduced Oxidative Damage Induced by P-Limited Conditions
The impact of ANE treatments on oxidative damage induced by P-limited conditions was assessed by staining and H2O2 in the tissues of leaves in treated Z. mays plants (Figure 5). Control and ANE-treatments showed similar patterns of staining, whereas P-limited conditions showed more staining of and H2O2 than the ANE-treated plants (Figures 5A,B). These results suggested that ANE treatments helped plants to reduce the P-limited-induced accumulation of and H2O2 in situ. These results were further confirmed by quantification of the tissue and H2O2 contents (Figures 5C,D) that were significantly reduced in those ANE-treated plants grown under P-limited conditions, as compared with the control (Figures 5C,D).
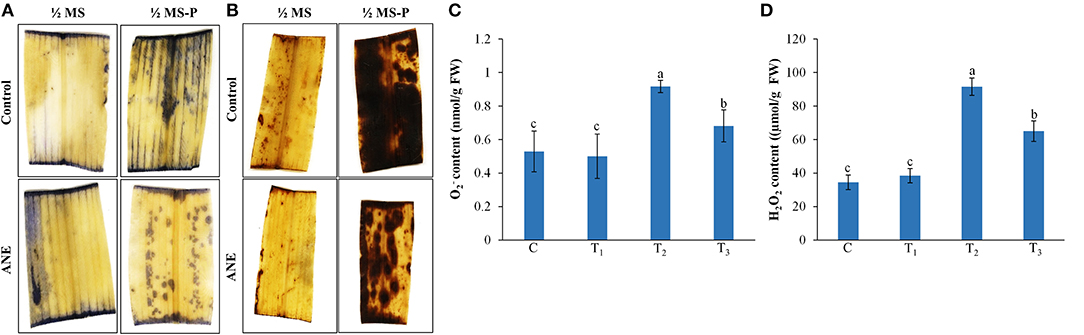
Figure 5. In vivo localization and quantification of (A,C) and (B,D) H2O2 in leaves of Z. mays grown for 21 days in perlite supplemented with 1/2 MS (C, control), 1/2 MS + ANE (T1), 1/2 MS-P (T2), 1/2 MS-P + ANE (T3). (A) Localization of by NBT staining, (B) localization of H2O2 by DAB staining, (C) quantification of content, and (D) quantification of H2O2 content. The values were presented as mean ± SE, and means represented by the same letters are not significantly different at p ≤ 0.01. Each experiment was carried out in triplicate, and each experimental unit had six plants (n = 18).
ANE Treatment Improved Total Content of Soluble Sugars, Amino Acids, Phenolics, and Flavonoids in the Z. mays Grown Under P-Limited Conditions
P-starvation is known to influence the carbohydrate metabolism in plants (Karthikeyan et al., 2007). In maize plants grown on P-sufficient media, the level of total soluble sugars remained almost the same in both the control and ANE-supplemented plants. Plants grown on a P-limited medium showed a significant reduction in total soluble sugars content (Figure 6A). The supplementation of media with 0.01% ANE in P-limited media significantly increased the total soluble sugars content by 74%, as compared with the plants grown in P-starved medium (Figure 6A). The increased levels of total amino acids content were observed in those plants grown in 1/2 MS + 0.01% ANE, as compared with the control (Figure 6B). P-limitation showed a significant (p ≤ 0.01) reduction in amino acid metabolites. ANE application improved total amino acids content in those plants grown in P-limited media (Figure 6B). Imposition of a P-limited condition induced the total phenolics content, whereas the addition of ANE in the P-limited media reduced the total phenolics content in the plant (Figure 6C), suggesting that ANE treatment relieved plants from the deleterious effects of P-limitation. Similarly, the total flavonoids content was also significantly reduced in plants grown in ANE-supplemented P-limited media (Figure 6D). These results suggest that ANE supplementation in P-starved media improved plant growth by modulating the biochemical status of the plants.
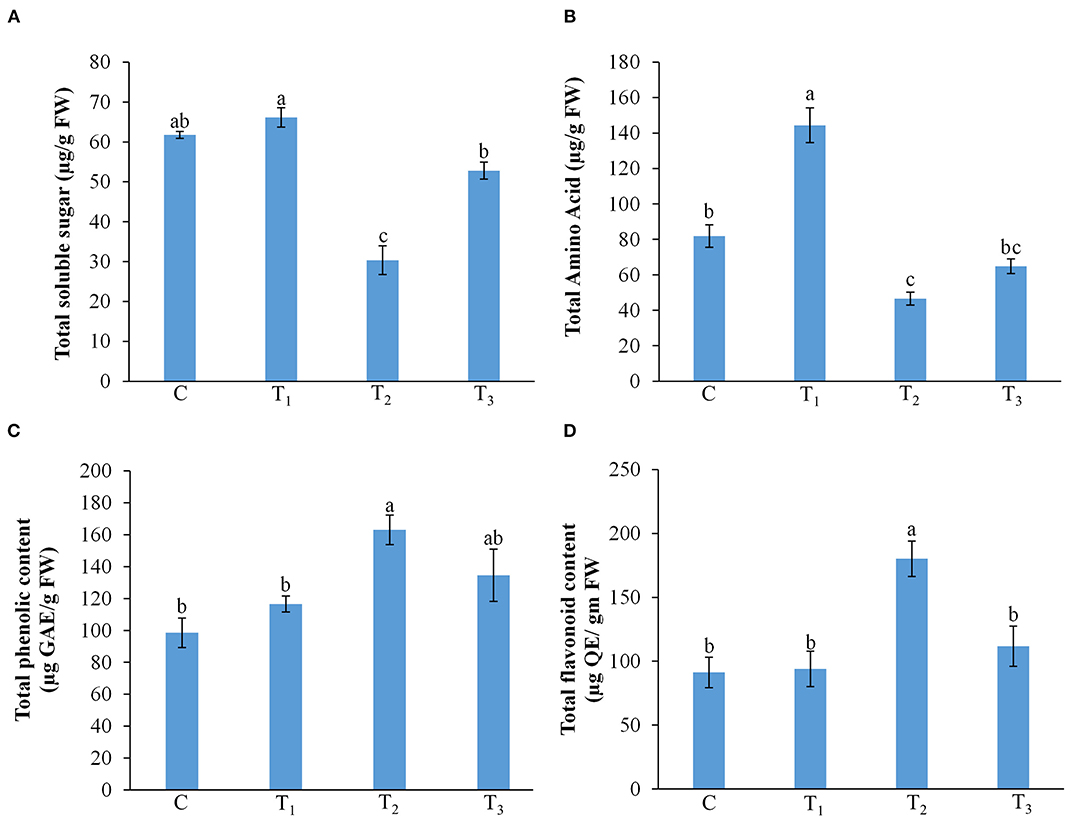
Figure 6. Effect of ANE on (A) total soluble sugar, (B) total amino acid, (C) total phenolic, and (D) total flavonoid content of Z. mays under the P-limiting conditions. C (control): plants grown in perlite supplemented with 1/2 MS, T1: plants grown in perlite supplemented with 1/2 MS + ANE, T2: plants grown in perlite supplemented with 1/2 MS-P, T3: plants grown in perlite supplemented with 1/2 MS-P + ANE. The values were presented as mean ± SE, and means represented by the same letters are not significantly different at p ≤ 0.01. Each experiment was carried out in triplicate, and each experimental unit had six plants (n = 18).
Treatments With ANE Regulated the Expression of Genes Involved in P Homeostasis in Z. mays Grown Under P-Limited Conditions
To further elucidate the role of ANE treatments in ameliorating plant growth in P-impoverished conditions, the expression of genes involved in P homeostasis was evaluated in both the shoots and roots of Z. mays plants after 2 and 8 days of P-limited conditions. PHR1, a GARP-type MYB transcription factor, was significantly induced in the shoots and roots of the control, as well as ANE-supplemented plants grown under P-limited conditions, at both time-points investigated (Figure 7A). The induction of PHR1 in the roots and shoots of ANE-supplemented plants was significantly less (p ≤ 0.01) than that of the control. No significant change in the expression of PHT1 was observed in the shoots of Z. mays grown in the different treatments (Figure 7B). After 2 and 8 days, PHT1 was significantly induced in the roots of plants grown in 1/2 MS-P and 1/2 MS-P + ANE. The addition of ANE in P-limited media limited the transient up-regulation of PHT1, as observed in those plants grown under P-limitation alone (Figure 7B). PTF1, a P-starvation-induced, basic helix–loop–helix (bHLH) transcription factor, was induced in shoots after 2 days of imposition of P-limited conditions in treatments supplemented with ANE vs. control (Figure 7C). After 8 days, ANE treatments showed a reduction in the expression of PTF1 (Figure 7C). In roots, P-limited conditions induced the expression of PTF1 at both time-points, whereas ANE supplementation in P-starved media showed reductions in the expression of PTF1 (Figure 7C). These results suggested that experimental supplementation with ANE reduced the physiological severity of P-limitation.
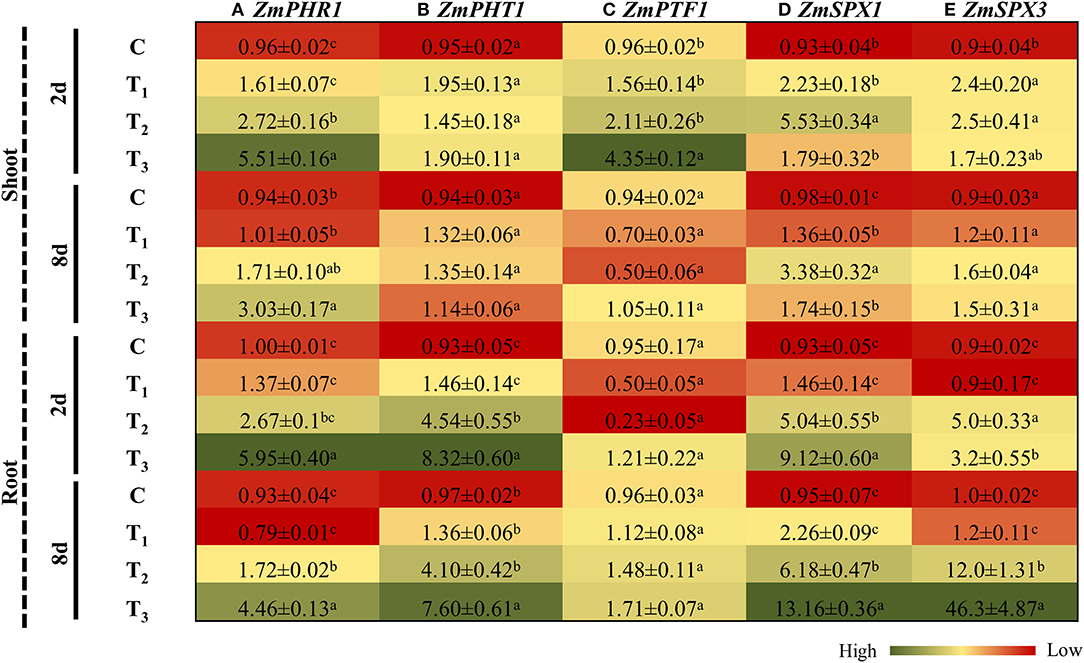
Figure 7. ANE altered the expression of (A) ZmPHR1, (B) ZmPHT1, (C) ZmPTF1, (D) ZmSPX1, and (E) ZmSPX3 involved in phosphate homeostasis in Z. mays grown under P-limiting conditions. The colors ranging from red to green represent the average values of fold change (red, low expression and green, high expression). The values were presented as mean ± SE of three independent replicates, and significantly different mean values were represented by different alphabets.
SPX (SYG1/Pho81/XPR1) genes are known to play a key role in maintaining P homeostasis in plants (Secco et al., 2012). The expression of SPX1 was significantly induced in ANE-supplemented plants, as compared with the controls. From day 2 to day 8 of P-limitation, the expression of SPX1 was reduced from 5.94 times to 3.44 times in the shoots of Z. mays (Figure 7D). However, no change was observed in the expression of SPX1 in ANE-supplemented plants across the time-points. After 2 and 8 days of application of ANE, the expression SPX1 was increased by 1.46 and 2.26 times in the roots (Figure 7D). Under P-limited conditions, the SPX1 transcript was strongly expressed (5.04 times) at day 2, which was further increased to 6.18 times after 8 days of treatment. However, the addition of ANE in the 1/2 MS-P highly induced the expression of SPX1 in the roots at both time-points (Figure 7D). The expression of SPX3 remained unaltered in the shoots of plants grown in different treatments at both time-points. Similarly, plants grown in 1/2 MS (C) and 1/2 MS + ANE (T1) did not show any change in the expression of SPX3 in their roots, across different time-points. P-limitation consistently increased the expression of SPX3 in roots, at both time-points. Overall, under P-limitation, the application of ANE resulted in strong induction (14.5 times) across the time-points, whereas P-limited conditions alone showed only 2.4 times induction from days 2 to 8 after treatment (Figure 7E).
ANE Treatment Regulated the Expression of Genes Involved in Carbohydrate Metabolism in the Z. mays Grown Under P-Limited Conditions
P-starvation had a direct impact on photosynthesis and consequently reduced the carbon assimilation in those treatments. To cope with this situation, plants maneuver the expression of different genes involved in carbohydrate metabolism (Hermans et al., 2006). In shoots, under control conditions, ANE applications resulted in a significant induction (11.5 times) in the expression of SUC2, a gene involved in organ-specific sucrose transportation. After 2 days of imposition of P-limited conditions, the expression of SUC2 induced the expression by 8.5 times in the shoots, as compared with the control, whereas supplementation of ANE in the P-limited media further induced the expression by 17.2 times. However, after 8 days, the expression pattern of SUC2 was found to be 3.18 times higher in the shoots of ANE-supplemented plants grown in P-limited media than in those plants grown in P-limited media alone (Figure 8A). In the roots, initially, after 2 days, SUC2 was down-regulated in all treatments, as compared with the control, whereas at the later stage, all plants showed induction of SUC2 in all treatments, as compared with the control (Figure 8A). The Glucose 6P/P translocator (G6Ptr) involved in the conversion of Glucose 6P to starch was differentially regulated in the shoots and roots of Z. mays grown under P-limited conditions (Figure 8B). After 2 days of P-limitation, ANE supplementation induced the expression of G6Ptr in the shoots (Figure 8B), whereas after 8 days, no differences in expression patterns were observed in any of the treatments. In roots, the transcript accumulation of G6Ptr was found to be higher at both time-points in those Z. mays plants that were treated with ANE and grown under P-limited conditions than the control (Figure 8B). Initially, no changes in the expression patterns of sucrose phosphate synthase (SPS) were observed in the shoots and roots of ANE-treated, P-limited plants. However, after 8 days of P-limitation, ANE-supplemented plants showed a significant induction in the expression of SPS, as compared with the control (Figure 8C). ANE applications significantly stimulated the expression of pyruvate kinase 1 (PK1) in the roots and shoots of plants grown under P-limited conditions, at both time-points, as compared with the plants grown in P-limited media alone (Figure 8D). ADP-glucose pyrophosphorylase (AGPase) was significantly induced in the shoots of ANE-treated, P-limited plants at both time-points. In the roots, after 2 days of P-limitation, the expression of AGPase was 2.6 times higher in the ANE treatments, which declined to 1.33 times after 8 days, than in the control (Figure 8E). Likewise, phosphoenolpyruvate carboxylase (PEPCase), an important gene involved in carbon assimilation, was found to be significantly up-regulated in the shoots and roots of ANE-supplemented, P-limited plants, at both time-points (Figure 8F). These results suggest that the application of ANE regulated the carbon assimilation and better plant growth resulted under P-limited conditions.
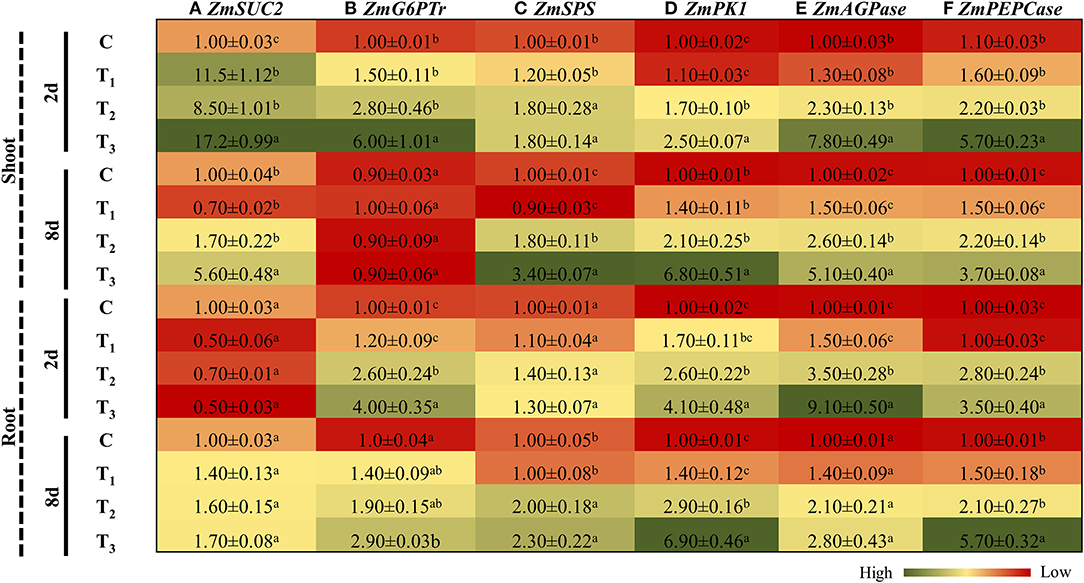
Figure 8. ANE regulated the expression of (A) ZmSUC2, (B) ZmG6PTr, (C) ZmSPS, (D) ZmPK1, (E) ZmAGPase, and (F) ZmPEPCase involved in carbohydrate metabolism in Z. mays grown under P-limiting conditions. The colors ranging from red to green represent the average values of fold change (red, low expression and green, high expression). The values were presented as mean ± SE of three independent replicates, and significantly different mean values were represented by different alphabets.
ANE Treatment Regulated the Expression of Genes Involved in Lipid Metabolism in Z. mays Grown Under P-Limited Conditions
To survive in P limiting conditions, plants go through significant alterations in their membrane lipid compositions by regulating the expression of different genes involved in lipid metabolism. Monogalactosyldiacylglycerol (MGDG) and digalactosyldiacylglycerol (DGDG) are the main components of glycolipids of the thylakoid membrane. MGDG synthase (MGDGS) and DGDG synthase (DGDGS) catalyze the formation of MGDG and DGDG. P-limited conditions induced the expression of MGDGS and DGDGS in the shoots and roots of Z. mays at both time-points (Figures 9A,B). ANE supplementation increased the expression of MGDGS in the shoots of Z. mays grown under P-limited conditions, by 2.6 and 1.58 times at 2 and 8 days, respectively. Similarly, in the roots, the expression of MGDGS was significantly induced at both time-points in ANE-treated, P-limited plants (Figure 9A). After 2 days of P-limited conditions, no changes in the expression pattern of DGDGS were observed in either the shoots or roots of the treatments (Figure 9B). Prolonged exposure of P-limited conditions in the presence of ANE increased the transcript accumulation of DGDGS in shoots, whereas no substantial differences were observed in the roots, as compared with the control (Figure 9B). Phosphatidylinositol (PIS) is a key enzyme involved in phospholipid pathways. After 2 days, the expression of PIS was significantly reduced in plants grown in 1/2 MS-P (T2) and 1/2 MS-P + ANE (T3), as compared with those plants grown in 1/2 MS (C) and 1/2 MS + ANE (T1) (Figure 9C). ANE significantly induced the expression of PIS after 8 days, as compared with the control plants (Figure 9C). P-limited conditions, however, increased the expression of PIS from 0.7 to 2.5 times from day 2 to 8, whereas the treatment of ANE application in P-starved media increased expression from 0.6 to 4.2 times from day 2 to 8 (Figure 9C). Notably, in the roots, no difference was observed in the expression of PIS among all the treatments at both time-points (Figure 9C). In the shoots of those plants grown under P-limited conditions, the expression of fatty acid desaturase 7 (FAD7) increased from 2.2 to 5.1 times between time-points. This trend was not observed in plants grown under P-limited conditions with ANE treatment (Figure 9D). In roots, FAD7 was also induced at both the time-points by P-limited conditions. By contrast, the expression was reduced from 6 to 2.6 times from day 2 to 8 (Figure 9D). In the roots of ANE-treated plants, under P-limited condition, FAD7 was also induced at both time-points, but to a lesser degree than those plants grown in P-limited conditions (Figure 9D). The expression of diacylglycerol kinase 1 (DGK1) was significantly induced (2.2 times) in the shoots of plants treated with ANE, as compared with the control conditions (Figure 9E). P-limited conditions increased the expression of DGK1 in the shoots of plants grown with ANE, as well as without ANE, but the expression was 2.81 times higher in ANE-supplemented plants grown under P-limited conditions than in the plants grown under P-limited conditions alone (Figure 9E). In roots, DGK1 was significantly induced at both time-points by P-limited conditions; however, ANE supplementation in P-starved media induced DGK1 by 2.25 times and 1.4 times at 2 and 8 days, respectively (Figure 9E). These results suggest that ANE supplementation regulated the expression of genes involved in lipid metabolism that helped treated plants to grow better under P-limited conditions.
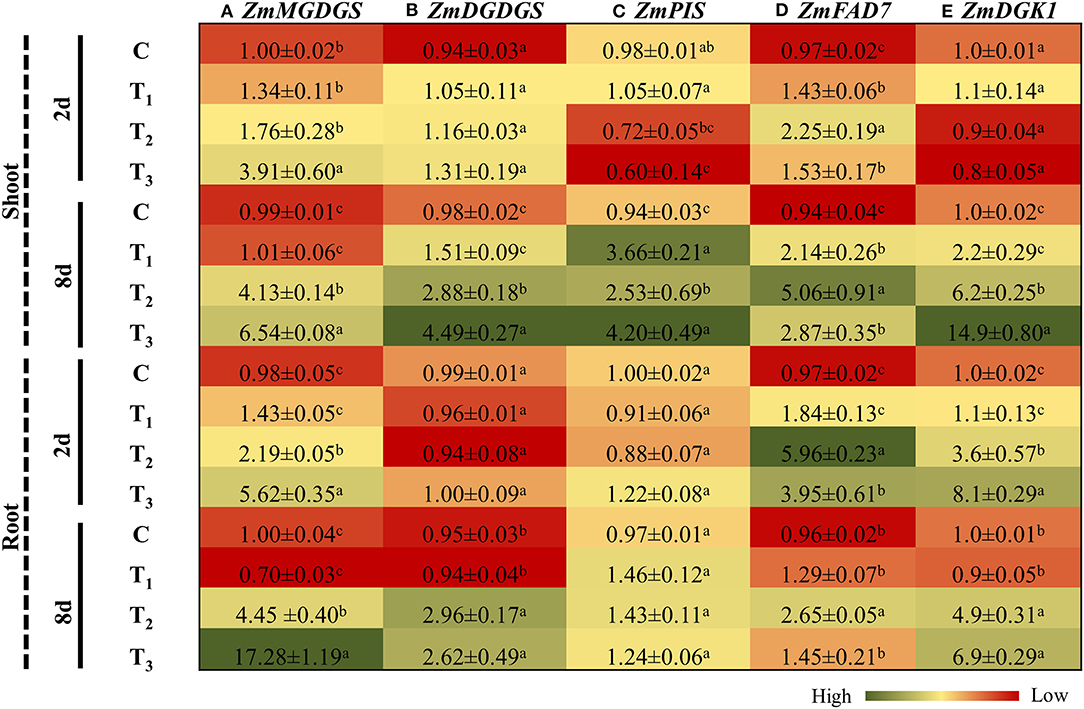
Figure 9. ANE altered the expression of (A) ZmMGDGS, (B) ZmDGDGS, (C) ZmPIS, (D) ZmFAD7, and (E) ZmDGK1 involved in lipid metabolism in Z. mays grown under P-limiting conditions. The colors ranging from red to green represent the average values of fold change (red, low expression and green, high expression). The values were presented as mean ± SE of three independent replicates, and significantly different mean values were represented by different alphabets.
ANE Treatment Regulated the Expression of Genes Involved in Secondary Metabolism in Z. mays Under P-Limited Conditions
After 2 days of treatment, Bronze 2 (Bz2), an anthocyanin biosynthetic gene in maize, was significantly down-regulated (2 times) in the shoots of plants grown in the 1/2 MS media supplemented with ANE, as compared with the control plants (Figure 10A). Bz2 was induced by 1.86 times in plants grown for 2 days in 1/2 MS-P (T2), as compared with the plants grown in 1/2 MS-P + ANE (T3) (Figure 10A). The expression of Bz2 was reduced in the shoots after 8 days under P-limited conditions. However, no changes were observed in shoots of the ANE-treated plants under P-limited conditions (Figure 10A). In roots, after 2 days of treatment, Bz2 was down-regulated in all treatments, as compared with the control, whereas maximum reduction in expression was observed in those plants grown in 1/2 MS + ANE (T1) (Figure 10A). Prolonged exposure of P-limited conditions increased the expression of Bz2 in those plant roots. Addition of ANE in P-limited media significantly increased the expression of Bz2 in the roots by 2.7 times, as compared with the plants grown under P-limited conditions alone (Figure 10A). Flavonol synthase 1 (FLS1), an important gene involved in flavonol biosynthesis, was differentially regulated in the shoots and roots of Z. mays in all treatments (Figure 10B). Initially, no change in the expression of FLS1 was observed (Figure 10B). However, after 8 days under P-limited conditions, ANE-supplemented plants showed 1.8 times increased expression of FLS1, as compared with the P-limited plants alone (Figure 10B). No significant changes were observed in the expression of FLS1 in the roots of plants grown under different treatments, at both time-points (Figure 10B). The expression of cinnamyl alcohol dehydrogenase (ZmCAD) remained unchanged in the shoots and roots of Z. mays in all treatments after 2 days (Figure 10C). Expression analysis revealed that the transcript accumulation of CAD increased in the shoots of ANE-supplemented plants grown for 8 days under P-limited conditions. In roots at 8 days after treatment, expression was significantly reduced in the ANE-treated plants grown under P-limited conditions (Figure 10C). Under P-limited conditions, ANE supplementation significantly increased the expression of dihydroflavonol-4-reductase (ZmDHFR) and chalcone synthase (ZmCHS) in the shoots of Z. mays at both time-points (Figures 10D,E). These results allow us to draw a conclusion that ANE regulates secondary metabolism of plants grown under P-limited conditions and help the plant to reduce the deleterious effect of P-limitation.
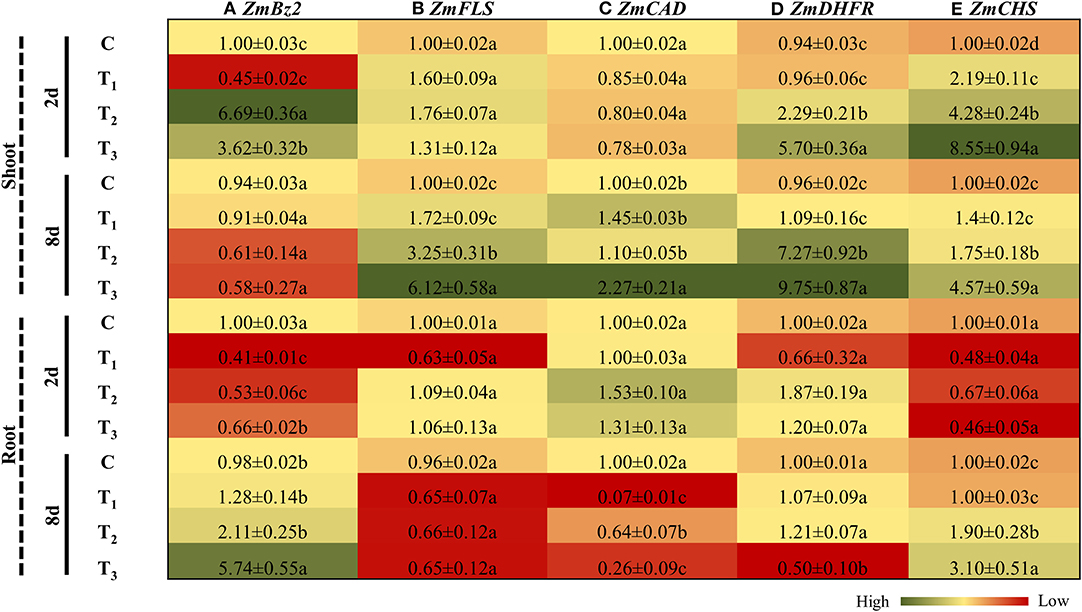
Figure 10. ANE altered the expression of (A) ZmBz2, (B) ZmFLS, (C) ZmCAD, (D) ZmDHFR, and (E) ZmCHS involved in secondary metabolism in Z. mays grown under P-limiting conditions. The colors ranging from red to green represent the average values of fold change (red, low expression and green, high expression). The values were presented as mean ± SE of three independent replicates, and significantly different mean values were represented by different alphabets.
Discussion
In order to provide basic food security for an ever-increasing global population, chemical fertilizers have been used excessively to increase agricultural productivity (Savci, 2012a). Excessive use of chemical fertilizers acts as an agricultural pollutant (Savci, 2012a,b) and is a significant anthropogenic threat to the world's terrestrial and aquatic ecosystems. Nevertheless, P is an important soil nutrient required for the maintenance of plant growth and productivity and is generally supplied in the form of chemical fertilizer (Kim et al., 2018). To address this challenge, there is a compelling need to develop sustainable strategies that enhance the agricultural output, while minimizing the chemical input.
In this study, we report that judicious applications of an alkaline extract of ANE are sustainable strategies to improve the growth of Z. mays grown under P-impoverished conditions. The ICP-OES analysis reveals the presence of 5.0 μM of P in 0.01% ANE (Supplementary Table 2), and the same amount of P was added to 1/2 MS-P to construct a positive control that would define the role of ANE in ameliorating the growth of plant in P-limited conditions. ANE improved leaf area, root length, and fresh and DW of shoots and roots of corn grown under P-limited conditions. The application of ANE showed increased uptake of Mg, B, Mn, Zn, and Cu in those shoots grown under P-limited conditions. Similarly, Di Stasio et al. (2018) also reported that two commercial extracts, Rygex® and Super Fifty®, also prepared from A. nodosum, enhanced the nutrient contents of tomato fruits. Additionally, the supplementation of ANE in P-limited media reduces Fe content in plants, as compared with the plants grown in P-limited media alone. Briat et al. (2015) explained the physiological connections between reductions in Fe content in plants grown under P-limitation. ANE-supplemented plants grown under P-limited conditions showed higher P content than plants grown in P-limited media alone, which demonstrated the “biostimulant boost” of ANE, as opposed to it acting as a micro- or macronutrient fertilizer alone. To further explain this behavior, we conducted expression analysis of genes involved in P homeostasis. ANE differentially up-regulated the expression of ZmPHR1 in those shoots and roots of Z. mays grown under P-limited conditions. In the present study, ANE up-regulated the expression of PHR1 by down-regulating the expression of SPX1 in the shoots grown under P-limited conditions (Figure 11). PHR1, a MYB transcription factor, is known to control a large part of P-starvation responsive transcriptome (Nilsson et al., 2007; Pant et al., 2015), and its expression is negatively regulated by SPX1 and SPX3 (Puga et al., 2017). In addition to its role in P homeostasis, PHR1 also controls the integration of P and Fe homeostasis (Briat et al., 2015). High P availability down-regulates the expression of PHO2 which in turn induces the expression of PHT1, phosphate transporter genes expressed in the roots of plant (Doerner, 2008). ANE supplementation also induced the expression of PHT1 in the roots of plants grown under P-limitation, whereas its expression remained unchanged in the shoots. ANE treatment also modulated the expression of PTF1, a bHLH transcription factor involved in tolerance to P-limited conditions (Li et al., 2011). Similarly, Shukla et al. (2018) showed that the application of ANE in P-starved media improves the growth of Arabidopsis. ANE is also reported to increase P uptake in Arabidopsis grown under saline conditions by regulating the expression of regulatory RNAs and genes involved in the efficient relocation of P resources (Shukla et al., 2018, 2019). Taken together, these results suggest that applications of ANE improved the growth of Z. mays by regulating the expression of genes involved in P homeostasis (Figure 11).
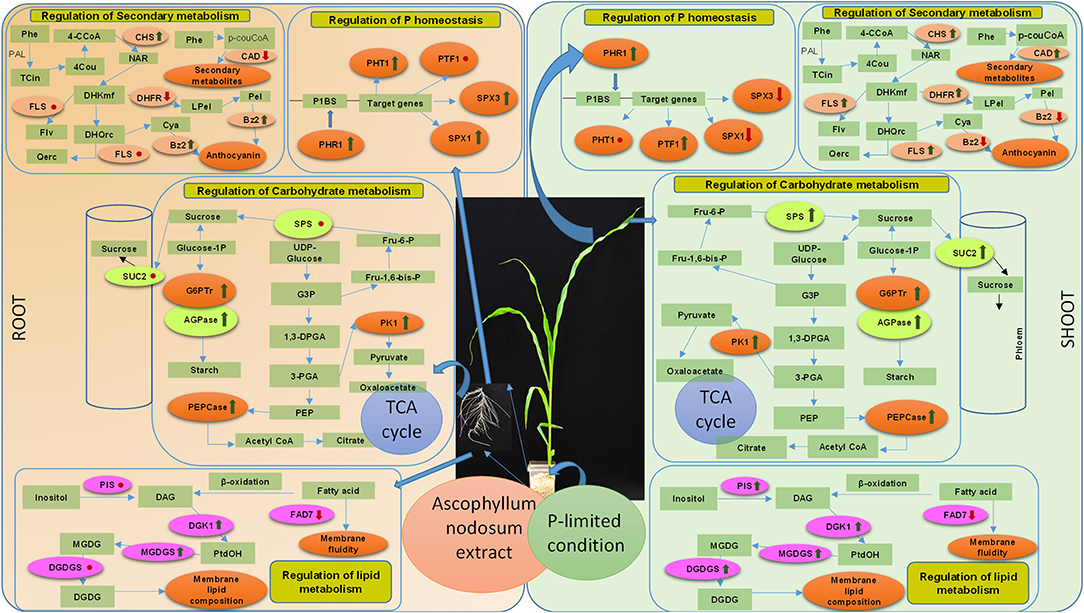
Figure 11. A schematic presentation of regulation of the genes involved in P homeostasis, lipid, carbohydrate, and secondary metabolism by the A. nodosum extract in the shoots and roots of the Z. mays grown under P-impoverished conditions. The green arrow shows the up-regulation of the gene expression, whereas the red arrow shows the down-regulation. The red dot represents no significant change in the expression. Abbreviations listed in the diagram are as follows: P homeostasis (PHR1, Phosphate Starvation Response 1; PTF1, basic helix–loop–helix domain containing transcription factor; SPX1 and SPX3, SYG1/Pho81/XPR1 domain containing protein; PHT1, Pi transporters), carbohydrate metabolism (SUC2, sucrose transporter; SPS, sucrose phosphate synthase; PEP, phosphoenolpyruvate; AGPase, ADP-glucose pyrophosphorylase; G6Ptr, Glucose 6P/P translocator; PK, pyruvate kinase 1; PEPCase, phosphoenolpyruvate carboxylase; G3P, Glyceraldehyde-3-phosphate; 1,3-DPGA, 1,3 di-phosphoglyceraldehyde; 3-PGA, 3-phosphoglyceraldehyde; Fru 6-P, fructose-6-phosphate; Fru-1,6-bis-P, fructose 1,6-bis-phosphate), secondary metabolism (Phe, phenylalanine; TCin, transcinnamate; 4Cou, 4-coumarate; 4-CCoA, 4-coumaroyl CoA; Nar, naringenin; DHKmf, dihydrokaempferol; Flv, flavonoids. DHQrc, dihydroquercetin; Qerc, quercetin. Lpel, leucopelargonidin. Cya, cyanidin; Pel, pelargonidin; PAL, phenylalanine ammonia lyase; CHS, chalcone synthase; FLS, flavonol synthase; BZ2, glutathione-S-transferase encoded by Bronze 2; DFR, dihydroflavonol-4-reductase; pcouCoA, p-coumaroyl-CoA; CAD, cinnamyl alcohol dehydrogenase).
To sustain growth in P-limited conditions, plants have developed a number of developmental and metabolic responses to adapt to both the internal P status in plants and the external soil P availability (Jiang et al., 2007; Pant et al., 2014). P-limited conditions reduce the chlorophyll content in the plants (Manna et al., 2015). The supplementation of ANE in P-starved media increased chlorophyll a and b and carotenoid contents of Z. mays. Contrary to the chlorophyll content, P-limited conditions contributed to anthocyanin accumulation (Jiang et al., 2007), whereas the accumulation was less in ANE-supplemented plants grown under P-limited conditions. Phosphorous limitation has a direct impact on photosynthesis and leads to reduced carbon assimilation (Rosa et al., 2009; Lemoine et al., 2013). It is well-known that P deficiency reduces the sugar and starch accumulation in the leaves of plants by regulating the expression of those genes involved in photosynthesis and sucrose biosynthesis (Cakmak et al., 1994; Hermans et al., 2006). In the present study, the application of ANE in P-limited media increased the total soluble sugars by regulating the expression of genes involved in carbohydrate metabolism (Figure 11). Under P-limited conditions, plants reallocate their carbon sources to their roots by increasing the loading of sucrose to the phloem through regulating the expression of SUC2, a sucrose transporter involved in phloem loading (Lloyd and Zakhleniuk, 2004; Hammond and White, 2008). ANE supplementation increased sucrose translocation to the roots by increasing the expression of SUC2 in the shoots of plants grown under P-limited conditions. However, expression was unaltered in the roots. In addition, the expression of SPS was also found to be highest in ANE-supplemented plants, grown under P-limitation. Low P activated the expression of SPS (Morcuende et al., 2007; Rosa et al., 2009), which was further enhanced by the addition of ANE in the P-limited media. ANE supplementation modulated the expression of G6PTr in the shoots, in response to P-limitation. G6PTr and AGPase are involved in the conversion of glucose-1P to starch and are reported to be differentially regulated in response to P-limited conditions (Hermans et al., 2006; Calderon-Vazquez et al., 2008). P-limited conditions regulate the allosteric regulation of AGPase in starch synthesis (Hermans et al., 2006). ANE increased the expression of AGPase in both the shoots and roots, in response to P-limitation, at both time-points. Metabolites involved in carbon assimilation were also reduced in P-starved plants (Morcuende et al., 2007). The expression of pyruvate carboxylase (PEPCase) and PK1 was induced by ANE in response to P-limited conditions. Thus, ANE supplementation showed an increase in the glycolytic intermediates in those plants grown under P-limitation which, in turn, increased CO2 assimilation. These results provide clear evidence that ANE treatment not only helped to relocate carbohydrate resources within plants but also has the potential to regulate sugar signaling cascades that assist treated plants to improve growth under P-impoverished conditions.
Low phosphorous availability induces photo-oxidative damage in plants (Hernández and Munné-Bosch, 2015). P-limited conditions in plants lead to alterations of the photosynthetic apparatus, including reduction in carbon fixation, leading to the generation of reactive oxygen species (Hernández and Munné-Bosch, 2015). In this series of experiments, those plants treated with ANE reduced the accumulation of P-limited-induced reactive oxygen species. In addition to this, ANE-supplemented plants also showed the reduction in P-limited-induced electrolyte leakage by improving membrane stability. Several reports showed that low P availability increased the MDA content, a biomarker of lipid peroxidation in plants grown under P-limited conditions (Zhang et al., 2014). ANE supplementation reduced the accumulation of MDA content in plants grown under P-limited conditions. Membrane lipid remodeling is one of the most important metabolic adaptations in response to P-starvation (Nakamura et al., 2009; Pant et al., 2015). In an adaptive response to P-starvation, plants promote the re-mobilization of internal P by replacing the composition of membrane lipids rich in phospholipids with non-phosphorous glycerolipids, such as MGDG and DGDG (Nakamura et al., 2009; Nakamura, 2013). ANE supplementation triggered the lipid remodeling response during P-limitation by inducing the expression of MGDGS and DGDGS, key genes involved in their synthesis from diacylglycerol (DAG). Similarly, Calderon-Vazquez et al. (2008) also demonstrated that P-starvation differentially modulated the expression of the genes involved in lipid metabolism. Phosphatidylinositol synthase (PIS) and DGK1, important genes involved in phospholipid metabolism who act as phospholipid signaling molecules (Liu et al., 2013), were also differentially regulated by ANE in those plants grown under P-starvation. Plant fatty acid desaturases catalyze the desaturation of fatty acids and play an important role in regulating fatty acid composition that maintains membrane fluidity (Zhao et al., 2019). ANE altered the saturation pattern of membrane lipids by regulating the expression of FAD7. Furthermore, ANE treatments reduced the expression of FAD7 in plants grown under P-limited conditions. These results suggest that to conserve phosphorus in situations of P-limitation, ANE remodeled membrane phospholipids and replaced them by increased biosynthesis of galactolipids (Ticconi and Abel, 2004).
Accumulation of secondary metabolites are typical P-starvation responses that recycle significant amounts of P from phosphorylated precursors (Ticconi and Abel, 2004; Malusà et al., 2006). ANE supplementation increased the total amino acid content in the leaves of those corn plants grown under P-limited conditions. Low P availability is reported to differentially reduce the amino acid content and increase flavonoid content in Camellia sinensis. P-starvation induces the accumulation of flavonoids and phenolics in plants (Stewart et al., 2001; Trejo-Téllez et al., 2019). ANE treatment showed a reduction in the P-limitation-induced accumulation of anthocyanin, flavonoids, and phenolics contents in the corn. Bronze-2 (Bz2) plays an important role in the anthocyanin biosynthetic pathway and is significantly induced under P-limited conditions in the roots of maize (Nash and Walbot, 1992; Calderon-Vazquez et al., 2008; Calderón-Vázquez et al., 2011). The expression of Bz2 was reduced at the early time-point in the shoot of ANE-treated Z. mays grown under P-limited conditions, whereas its expression was induced by ANE in the roots after the prolonged exposure of P-limitation. Similarly, ANE differentially regulated the expression of flavonol synthase (FLS), cinnamyl alcohol dehydrogenase (CAD), dihydroflavonol-4-reductase (DHFR), and chalcone synthase (CHS) in the shoots and roots of Z. mays grown under P-limited treatments (Figure 11).
Conclusion
The largely undetermined bioactive components present in the chemically complex, crude commercial extract of ANE improved the growth of Z. mays plants grown under P-limited conditions by efficiently relocating the internal P reserves. This was achieved by regulating the physiological and biochemical processes in response to P-limited conditions. In conclusion, this study provided a holistic understanding regarding the mode of action of ANE in improving plant growth under P-limited conditions. This study provides the basis for the applications of ANE as a component of an important sustainable strategy to reduce the input of chemical fertilizers, especially those impoverished in P to agricultural farmlands. The results documented here are encouraging but to realize the full potential of ANE in nutrient management programs to reduce dependency on chemical fertilizers, more comprehensive field trials under natural conditions are required.
Data Availability Statement
The raw data supporting the conclusions of this article will be made available by the authors, without undue reservation.
Author Contributions
PS and BP conceived the idea, design of the experiments, drafted all versions of the manuscript, and contributed to the final versions of the manuscript. PS performed the experiments, performed data processing, analysis, and interpretation.
Funding
This project was supported by the Accelerate Cluster grant (IT08347) from Mitacs (Canada), awarded to BP. The funder provided support in the form of salary for PS.
Conflict of Interest
The authors declare that the research was conducted in the absence of any commercial or financial relationships that could be construed as a potential conflict of interest.
Acknowledgments
The authors are grateful to Dr. Alan T. Critchley (Acadian Seaplants Ltd., Canada) and Emily Peters (Saint Mary's University, Department of Biology, Canada) for their valuable suggestions in preparing the manuscript. Acadian Seaplants Ltd. is thanked for providing the Ascophyllum nodosum extract.
Supplementary Material
The Supplementary Material for this article can be found online at: https://www.frontiersin.org/articles/10.3389/fpls.2020.601843/full#supplementary-material
References
Achary, V. M. M., Ram, B., Manna, M., Datta, D., Bhatt, A., Reddy, M. K., et al. (2017). Phosphite: a novel P fertilizer for weed management and pathogen control. Plant Biotechnol. J. 15, 1493–1508 doi: 10.1111/pbi.12803
Bajpai, S., Shukla, P. S., Asiedu, S., Pruski, K., and Prithiviraj, B. (2019). A biostimulant preparation of brown seaweed Ascophyllum nodosum suppresses powdery mildew of strawberry. Plant Pathol. J. 35, 406–416. doi: 10.5423/PPJ.OA.03.2019.0066
Briat, J. F., Rouached, H., Tissot, N., Gaymard, F., and Dubos, C. (2015). Integration of P, S, Fe, and Zn nutrition signals in Arabidopsis thaliana: potential involvement of phosphate starvation response 1 (PHR1). Front. Plant Sci. 6:290. doi: 10.3389/fpls.2015.00290
Burgos, G., Amoros, W., Muñoa, L., Sosa, P., Cayhualla, E., Sanchez, C., et al. (2013). Total phenolic, total anthocyanin and phenolic acid concentrations and antioxidant activity of purple-fleshed potatoes as affected by boiling. J. Food. Compost. Anal. 30, 6–12. doi: 10.1016/j.jfca.2012.12.001
Cakmak, I., Hengeler, C., and Marschner, H. (1994). Changes in phloem export of sucrose in leaves in response to phosphorus, potassium and magnesium deficiency in bean plants. J. Exp. Bot. 45, 1251–1257. doi: 10.1093/jxb/45.9.1251
Calderon-Vazquez, C., Ibarra-Laclette, E., Caballero-Perez, J., and Herrera-Estrella, L. (2008). Transcript profiling of Zea mays roots reveals gene responses to phosphate deficiency at the plant- and species-specific levels. J. Exp. Bot. 59, 2479–2497. doi: 10.1093/jxb/ern115
Calderón-Vázquez, C., Sawers, R. J. H., and Herrera-Estrella, L. (2011). Phosphate deprivation in maize: genetics and genomics. Plant Physiol. 156, 1067–1077. doi: 10.1104/pp.111.174987
Chiou, T.-J. (2005). Regulation of phosphate homeostasis by microRNA in Arabidopsis. Plant Cell. 18, 412–421. doi: 10.1105/tpc.105.038943
Di Stasio, E., Van Oosten, M. J., Silletti, S., Raimondi, G., dell'Aversana, E., Carillo, P., et al. (2018). Ascophyllum nodosum-based algal extracts act as enhancers of growth, fruit quality, and adaptation to stress in salinized tomato plants. J. Appl. Phycol. 30, 2675–2686. doi: 10.1007/s10811-018-1439-9
Doerner, P. (2008). Phosphate starvation signaling: a threesome controls systemic Pi homeostasis. Curr. Opin. Plant Biol. 11, 536–540. doi: 10.1016/j.pbi.2008.05.006
George, T. S., and Richardson, A. E. (2008). “Potential and limitations to improving crops for enhanced phosphorus utilization,” in The Ecophysiology of Plant-Phosphorus Interactions, eds P. J. White and J. P. Hammond (Dordrecht: Springer), 247–270. doi: 10.1007/978-1-4020-8435-5_11
Hammond, J. P. (2003). Changes in gene expression in Arabidopsis shoots during phosphate starvation and the potential for developing smart plants. Plant Physiol. 132, 578–596 doi: 10.1104/pp.103.020941
Hammond, J. P., and White, P. J. (2008). Sucrose transport in the phloem: integrating root responses to phosphorus starvation. J. Exp. Bot. 59, 93–109. doi: 10.1093/jxb/erm221
Hermans, C., Hammond, J. P., White, P. J., and Verbruggen, N. (2006). How do plants respond to nutrient shortage by biomass allocation? Trends Plant Sci. 11, 610–617. doi: 10.1016/j.tplants.2006.10.007
Hernández, I., and Munné-Bosch, S. (2015). Linking phosphorus availability with photo-oxidative stress in plants. J. Exp. Bot. 66, 2889–2900. doi: 10.1093/jxb/erv056
Heuer, S., Gaxiola, R., Schilling, R., Herrera-Estrella, L., López-Arredondo, D., Wissuwa, M., et al. (2017). Improving phosphorus use efficiency: a complex trait with emerging opportunities. Plant J. 90, 868–885. doi: 10.1111/tpj.13423
Hichem, H., Mounir, D., and Naceur, E. A. (2009). Differential responses of two maize (Zea mays L.) varieties to salt stress: changes on polyphenols composition of foliage and oxidative damages. Ind. Crops Prod. 30, 144–151. doi: 10.1016/j.indcrop.2009.03.003
Hodges, D. M., DeLong, J. M., Forney, C. F., and Prange, R. K. (1999). Improving the thiobarbituric acid-reactive-substances assay for estimating lipid peroxidation in plant tissues containing anthocyanin and other interfering compounds. Planta 207, 604–611.
Hodges, D. M., and Lester, G. E. (2006). Comparisons between orange- and green-fleshed non-netted and orange-fleshed netted muskmelons: antioxidant changes following different harvest and storage periods. J. Am. Soc. Hortic. Sci. 131, 110–117. doi: 10.21273/JASHS.131.1.110
Irigoyen, J. J., Emerich, D. W., and Sánchez-Díaz, M. (1992). Water stress induced changes in concentrations of proline and total soluble sugars in nodulated alfalfa (Medicago sativa) plants. Physiol. Plant 84, 67–72. doi: 10.1111/j.1399-3054.1992.tb08766.x
Jannin, L., Arkoun, M., Etienne, P., Laîné, P., Goux, D., Garnica, M., et al. (2013). Brassica napus growth is promoted by Ascophyllum nodosum (L.) Le Jol. Seaweed extract: microarray analysis and physiological characterization of N, C, and S metabolisms. J. Plant Growth Regul. 32, 31–52. doi: 10.1007/s00344-012-9273-9
Jiang, C., Gao, X., Liao, L., Harberd, N. P., and Fu, X. (2007). Phosphate starvation root architecture and anthocyanin accumulation responses are modulated by the gibberellin-DELLA signaling pathway in Arabidopsis. Plant Physiol. 145, 1460–1470. doi: 10.1104/pp.107.103788
Jithesh, M. N., Shukla, P. S., Kant, P., Joshi, J., Critchley, A. T., and Prithiviraj, B. (2019). Physiological and transcriptomics analyses reveal that Ascophyllum nodosum extracts induce salinity tolerance in Arabidopsis by regulating the expression of stress responsive genes. J. Plant Growth Regul. 38, 463–478. doi: 10.1007/s00344-018-9861-4
Karthikeyan, A. S., Varadarajan, D. K., Jain, A., Held, M. A., Carpita, N. C., and Raghothama, K. G. (2007). Phosphate starvation responses are mediated by sugar signaling in Arabidopsis. Planta 225, 907–918. doi: 10.1007/s00425-006-0408-8
Khan, W., Rayirath, U. P., Subramanian, S., Jithesh, M. N., Rayorath, P., Hodges, D. M., et al. (2009). Seaweed extracts as biostimulants of plant growth and development. J. Plant Growth Regul. 28, 386–399. doi: 10.1007/s00344-009-9103-x
Kim, J. A., Vijayaraghavan, K., Reddy, D. H. K., and Yun, Y. S. (2018). A phosphorus-enriched biochar fertilizer from bio-fermentation waste: a potential alternative source for phosphorus fertilizers. J. Clean. Prod. 196, 163–171. doi: 10.1016/j.jclepro.2018.06.004
Lemoine, R., La Camera, S., Atanassova, R., Dédaldéchamp, F., Allario, T., Pourtau, N., et al. (2013). Source-to-sink transport of sugar and regulation by environmental factors. Front. Plant Sci. 4:272. doi: 10.3389/fpls.2013.00272
Li, Z., Gao, Q., Liu, Y., He, C., Zhang, X., and Zhang, J. (2011). Overexpression of transcription factor ZmPTF1 improves low phosphate tolerance of maize by regulating carbon metabolism and root growth. Planta. 233, 1129–1143. doi: 10.1007/s00425-011-1368-1
Lichtenthaler, H. K. (1987). Chlorophylls and carotenoids: pigments of photosynthetic biomembranes. Methods Enzymol. 148, 350–382. doi: 10.1016/0076-6879(87)48036-1
Liu, F., and Pang, S. J. (2010). Stress tolerance and antioxidant enzymatic activities in the metabolism of the reactive oxygen species in two intertidal red algae Grateloupia turuturu and Palmaria palmate. J. Exp. Mar. Bio. Eco. 328, 82–87. doi: 10.1016/j.jembe.2009.11.005
Liu, X., Zhai, S., Zhao, Y., Sun, B., Liu, C., Yang, A., et al. (2013). Overexpression of the phosphatidylinositol synthase gene (ZmPIS) conferring drought stress tolerance by altering membrane lipid composition and increasing ABA synthesis in maize. Plant Cell Environ. 36, 1037–1055. doi: 10.1111/pce.12040
Livak, K. J., and Schmittgen, T. D. (2001). Analysis of relative gene expression data using real-time quantitative PCR and the 2−ΔΔCT Method. Methods 25, 402–408. doi: 10.1006/meth.2001.1262
Lloyd, J. C., and Zakhleniuk, O. V. (2004). Responses of primary and secondary metabolism to sugar accumulation revealed by microarray expression analysis of the Arabidopsis mutant, pho3. J. Exp. Bot. 55, 1221–1230. doi: 10.1093/jxb/erh143
Lutts, S., Kinet, J. M., and Bouharmont, J. (1996). NaCl-induced senescence in leaves of rice (Oryza sativa L.) cultivars differing in salinity resistance. Ann. Bot. 78, 389–398. doi: 10.1006/anbo.1996.0134
Malusà, E., Russo, M. A., Mozzetti, C., and Belligno, A. (2006). Modification of secondary metabolism and flavonoid biosynthesis under phosphate deficiency in bean roots. J. Plant Nutr. 29, 245–258. doi: 10.1080/01904160500474090
Manna, M., Islam, T., Kaul, T., Reddy, C. S., Fartyal, D., James, D., et al. (2015). A comparative study of effects of increasing concentrations of phosphate and phosphite on rice seedlings. Acta Physiol. 37:258. doi: 10.1007/s11738-015-2016-3
Morcuende, R., Bari, R., Gibon, Y., Zheng, W., Pant, B. D., Bläsing, O., et al. (2007). Genome-wide reprogramming of metabolism and regulatory networks of Arabidopsis in response to phosphorus. Plant Cell Environ. 30, 85–112. doi: 10.1111/j.1365-3040.2006.01608.x
Nakamura, Y. (2013). Phosphate starvation and membrane lipid remodeling in seed plants. Prog. Lipid Res. 52, 43–50. doi: 10.1016/j.plipres.2012.07.002
Nakamura, Y., Koizumi, R., Shui, G., Shimojima, M., Wenk, M. R., Ito, T., et al. (2009). Arabidopsis lipins mediate eukaryotic pathway of lipid metabolism and cope critically with phosphate starvation. Proc. Natl. Acad. Sci. U.S.A. 106, 20978–20983. doi: 10.1073/pnas.0907173106
Nash, J., and Walbot, V. (1992). Bronze-2 gene expression and intron splicing patterns in cells and tissues of Zea mays L. Plant Physiol. 100, 464–471. doi: 10.1104/pp.100.1.464
Nilsson, L., MÜller, R., and Nielsen, T. H. (2007). Increased expression of the MYB-related transcription factor, PHR1, leads to enhanced phosphate uptake in Arabidopsis thaliana. Plant Cell Environ. 30, 1499–1512. doi: 10.1111/j.1365-3040.2007.01734.x
Pant, B.-D., Pant, P., Erban, A., Huhman, D., Kopka, J., and Scheible, W.-R. (2014). Identification of primary and secondary metabolites with phosphorus status-dependent abundance in Arabidopsis, and of the transcription factor PHR1 as a major regulator of metabolic changes during phosphorus-limitation. Plant. Cell Environ. 38, 172–187. doi: 10.1111/pce.12378
Pant, B. D., Burgos, A., Pant, P., Cuadros-Inostroza, A., Willmitzer, L., and Scheible, W. R. (2015). The transcription factor PHR1 regulates lipid remodeling and triacylglycerol accumulation in Arabidopsis thaliana during phosphorus starvation. J. Exp. Bot. 66, 1907–1918 doi: 10.1093/jxb/eru535
Pereira, L., Morrison, L., Shukla, P. S., and Critchley, A. T. (2020). A concise review of the brown macroalga Ascophyllum nodosum (Linnaeus) Le Jolis. J. Appl. Phycol. 32, 3561–3584. doi: 10.1007/s10811-020-02246-6
Puga, M. I., Rojas-Triana, M., de Lorenzo, L., Leyva, A., Rubio, V., and Paz-Ares, J. (2017). Novel signals in the regulation of Pi starvation responses in plants: facts and promises. Curr. Opin. 39, 40–49. doi: 10.1016/j.pbi.2017.05.007
Rayirath, P., Benkel, B., Mark Hodges, D., Allan-Wojtas, P., MacKinnon, S., Critchley, A. T., et al. (2009). Lipophilic components of the brown seaweed, Ascophyllum nodosum, enhance freezing tolerance in Arabidopsis thaliana. Planta 230, 135–147. doi: 10.1007/s00425-009-0920-8
Rickard, D. A. (2000). Review of phosphorus acid and its salts as fertilizer materials. J. Plant Nutr. 23, 161–180. doi: 10.1080/01904160009382006
Roch, G. V., Maharajan, T., Ceasar, S. A., and Ignacimuthu, S. (2019). The role of PHT1 family transporters in the acquisition and redistribution of phosphorus in plants. Crit. Rev. Plant Sci. 38, 171–198. doi: 10.1080/07352689.2019.1645402
Rosa, M., Prado, C., Podazza, G., Interdonato, R., González, J. A., Hilal, M., et al. (2009). Soluble sugars-metabolism, sensing and abiotic stress a complex network in the life of plants. Plant Signal. Behav. 4, 388–393. doi: 10.4161/psb.4.5.8294
Savci, S. (2012a). An agricultural pollutant: chemical fertilizer. Int. J. Environ. Sci. Dev. 3:73. doi: 10.7763/IJESD.2012.V3.191
Savci, S. (2012b). Investigation of effect of chemical fertilizers on environment. APCBEE Proc. 1, 287–292. doi: 10.1016/j.apcbee.2012.03.047
Schachtman, D. P., Reid, R. J., and Ayling, S. M. (2002). Phosphorus uptake by plants: from soil to cell. Plant Physiol. 116, 447–453. doi: 10.1104/pp.116.2.447
Secco, D., Wang, C., Arpat, B. A., Wang, Z., Poirier, Y., Tyerman, S. D., et al. (2012). The emerging importance of the SPX domain-containing proteins in phosphate homeostasis. N. Phytol. 193, 842–851. doi: 10.1111/j.1469-8137.2011.04002.x
Shenoy, V. V., and Kalagudi, G. M. (2005). Enhancing plant phosphorus use efficiency for sustainable cropping. Biotechnol. Adv. 23, 501–513. doi: 10.1016/j.biotechadv.2005.01.004
Shukla, P. S., Agarwal, P. K., and Jha, B. (2012). Improved salinity tolerance of Arachis hypogaea (L.) by the interaction of halotolerant plant-growth-promoting rhizobacteria. J. Plant Growth Regul. 31, 195–206. doi: 10.1007/s00344-011-9231-y
Shukla, P. S., Borza, T., Critchley, A. T., Hiltz, D., Norrie, J., and Prithiviraj, B. (2018). Ascophyllum nodosum extract mitigates salinity stress in Arabidopsis thaliana by modulating the expression of miRNA involved in stress tolerance and nutrient acquisition. PLoS ONE 13:e0206221. doi: 10.1371/journal.pone.0206221
Shukla, P. S., Mantin, E. G., Adil, M., Bajpai, S., Critchley, A. T., and Prithiviraj, B. (2019). Ascophyllum nodosum-based biostimulants: sustainable applications in agriculture for the stimulation of plant growth, stress tolerance, and disease management. Front. Plant Sci. 10:655. doi: 10.3389/fpls.2019.00655
Stewart, A. J., Chapman, W., Jenkins, G. I., Graham, I., Martin, T., and Crozier, A. (2001). The effect of nitrogen and phosphorus deficiency on flavonol accumulation in plant tissues. Plant Cell Environ. 24, 1189–1197. doi: 10.1046/j.1365-3040.2001.00768.x
Sun, Y., Mu, C., and Liu, X. (2018). Key factors identified by proteomic analysis in maize (Zea mays L.) seedlings' response to long-term exposure to different phosphate levels. Proteome Sci. 16:19. doi: 10.1186/s12953-018-0147-3
Ticconi, C., and Abel, S. (2004). Short on phosphate: plant surveillance and countermeasures. Trends Plant Sci. 9, 548–555. doi: 10.1016/j.tplants.2004.09.003
Trejo-Téllez, L. I., Estrada-Ortiz, E., Gómez-Merino, F. C., Becker, C., Krumbein, A., and Schwarz, D. (2019). Flavonoid, nitrate and glucosinolate concentrations in Brassica species are differentially affected by photosynthetically active radiation, phosphate and phosphite. Front. Plant Sci. 10:371. doi: 10.3389/fpls.2019.00371
Vance, C. P., Uhde-Stone, C., and Allan, D. L. (2003). Phosphorus acquisition and use: critical adaptations by plants for securing a non-renewable resource. N. Phytol. 157, 423–447. doi: 10.1046/j.1469-8137.2003.00695.x
Veneklaas, E. J., Lambers, H., Bragg, J., Finnegan, P. M., Lovelock, C. E., Plaxton, W. C., et al. (2012). Opportunities for improving phosphorus-use efficiency in crop plants. N. Phytol. 195, 306–320. doi: 10.1111/j.1469-8137.2012.04190.x
Yadav, N. S., Shukla, P. S., Jha, A., Agarwal, P. K., and Jha, B. (2012). The SbSOS1 gene from the extreme halophyte Salicornia brachiata enhances Na+ loading in xylem and confers salt tolerance in transgenic tobacco. BMC Plant Biol. 12:188. doi: 10.1186/1471-2229-12-188
Zhang, K., Liu, H., Tao, P., and Chen, H. (2014). Comparative proteomic analyses provide new insights into low phosphorus stress responses in maize leaves. PLoS ONE 9:e98215. doi: 10.1371/journal.pone.0098215
Keywords: Ascophyllum nodosum extract, P-limited condition, chemical fertilizer, gene expression, Zea mays
Citation: Shukla PS and Prithiviraj B (2021) Ascophyllum nodosum Biostimulant Improves the Growth of Zea mays Grown Under Phosphorus Impoverished Conditions. Front. Plant Sci. 11:601843. doi: 10.3389/fpls.2020.601843
Received: 12 October 2020; Accepted: 23 November 2020;
Published: 08 January 2021.
Edited by:
Youssef Rouphael, University of Naples Federico II, ItalyReviewed by:
Izabela Michalak, Wrocław University of Science and Technology, PolandShane O. Connell, Institute of Technology, Tralee, Ireland
Copyright © 2021 Shukla and Prithiviraj. This is an open-access article distributed under the terms of the Creative Commons Attribution License (CC BY). The use, distribution or reproduction in other forums is permitted, provided the original author(s) and the copyright owner(s) are credited and that the original publication in this journal is cited, in accordance with accepted academic practice. No use, distribution or reproduction is permitted which does not comply with these terms.
*Correspondence: Balakrishnan Prithiviraj, bprithiviraj@dal.ca