- 1College of Land Resources and Environment, Jiangxi Agricultural University, Nanchang, China
- 2Key Innovation Center for the Integration of Industry and Education on Comprehensive Utilization of Agricultural Wastes, Prevention and Control of Agricultural Non-point Pollution of Jiangxi Province, Nanchang, China
- 3Department of Agronomy, Abdul Wali Khan University, Mardan, Pakistan
Whether combining rice-straw biochar (RSB) with leguminous cover crop (LCC) has synergistic effects in the rice production system or not, is still unknown. Two pot experiments were conducted to systematically explore the impacts of RSB on mass decomposition and nitrogen (N) release from LCC residues after incorporation into acidic paddy soil. Similarly, the effect of combining these two factors on soil nutrient status and microbial biomasses in the rice production system was also examined. Five treatments, namely, no N fertilizer (CK), 100% N fertilizer (150 kg N ha–1 as N100), 80% N fertilizer plus RSB (N80B), LCC (N80M), and a combination of RSB with LCC (N80BM), were included. The results indicated that biomass decomposition and N release pattern followed a double exponential decay model such that the addition of RSB slightly stimulated the rates of both mass decomposition and N release during the initial rapid phase of decomposition. Thereafter, it notably slowed down the rates of both these parameters during the relatively slower stage of incorporating LCC residues to paddy soil during early rice season. Compared to 100% N, applying 80% N in conjunction with RSB and/or LCC residue increased grain yield and its components (i.e., effective panicles, 1,000-grain weight, and fully filled grains) that subsequently increased N accumulation and its physiological use efficiency (PUEN) of rice shoot. Moreover, under 20% N, applying RSB and/or LCC residue remarkably increased the soil organic matter and total N, and soil microbial populations and biomasses, while the contents of NH4+ and NO3– were decreased in RSB-amended paddy soil (N80B and N80BM), in comparison with N100. Thus, combining RSB with LCC residue is a novel and promising management intervention for reducing mineral fertilizer use, improving soil fertility and rice production, and consequently minimizing the overall production cost in south China.
Introduction
The existing pressure on agriculture to meet the global food demand is expected to further intensify as the galloping world population may roughly cross 9 billion in 2045. Rice being a staple food plays a pivotal role as it is consumed by more than half of the world’s population and about 65% of people in China (Tang and Cheng, 2019). For a hugely populated country like China, that resides more than 1.4 billion people (19% of the world’s population), another 26.9% more rice grain should be produced by 2030 in comparison with 2000 to meet its domestic need and ensure food security, if rice consumption per capita stays at the current level (Cheng et al., 2007).
The unsustainable and intensive agricultural developing pattern has generated serious soil degradation and productivity deterioration-related concerns. Excessive application of nitrogen (N) fertilizer to reddish paddy soil has further exacerbated the menace by causing considerable reactive N losses which in turn result in severe environmental problems and human health issues in China. The solution is to raise awareness of farmers on managing mineral N fertilization strategy and increasing the use of organic fertilizer, to promote sustainable development of agriculture and environment (Jiang et al., 2018). Maintaining or even improving the sustainable fertility and productivity of limited arable reddish soil is highly essential for future rice production to overcome the challenge of producing more rice grain yields with lower environmental costs.
Leguminous cover crop (LCC) that biologically fixes N from the inexhaustible pool of atmospheric N with its symbiotic rhizobia system on roots, can be grown on paddy fields which may otherwise usually be fallow after the rice harvest. LCC is regarded as an important source that adds to soil fertility by mainly providing N. It generally has considerable amounts of aboveground and underground biomass (Poeplau et al., 2015). Thus, it subsequently increases C and N fluxes into the soil and provides energy for soil organisms during rotation, thereby contributing to improve organic matter, nutrient content and bacterial community in the soil (He et al., 2020). Incorporating LCC residue into paddy soil is an appropriate alternative fertilization option, which not only produces more rice yields without increasing chemical fertilizers, but also consistently increases N use efficiency (Xie et al., 2016; Yang L. et al., 2019). Similarly, LCC reduces N pollution while maintaining net economic benefits for the overall ecosystem. Thus, partially substituting the conventional mineral N fertilizer with other alternatives will have less environmental impact without negatively affecting rice productivity (Cai et al., 2018; Ding et al., 2018; Yang L. et al., 2019). After incorporating the LCC residue into the soil, the peak decomposition and N mineralization of the LCC residue occur about 30 days after incorporation (Zhou et al., 2019). This time frame unfortunately under most conditions may not synchronize nutrients release with rice crop demand, forcing farmers to incorporate LCC residue about 15 days prior to transplanting rice seedlings. Therefore, it is necessary to synchronize biomass decay and nutrient release from LCC residue with crop nutrient demand for improving potential benefits of LCC to sustainable agriculture.
Rice straw, a representative agricultural residue that accounts for almost 29% of total crop straw and contains millions of tons of nutrients every year in China, is generally treated improperly. It is either combusted or littered directly, thereby leading to energy and nutrients waste as well as extensive N loss and critical environmental pollutions. Moreover, on-site incorporating rice straw directly prior to planting rice seedlings would cause adverse effects, such as competing N against crop plants, increasing greenhouse gas emission (e.g., CH4 and N2O), plant diseases, and insect pests (Jiang et al., 2019; Liu et al., 2021), thereby resulting in rice grain yields reduction. Hence, issues about effectively using rice straw are becoming more and more important as the concerns on food security and environmental protection increase in China. Biochar, a carbon-rich porous solid, is produced from various agricultural and forest wastes via oxygen-limited pyrolysis at a high temperature up to 700°C (Lehmann and Joseph, 2009). Recently, biochar has significantly received interests in increasing soil inorganic nutrients, reducing mean cumulative leaching of NO3– due to the capability of retaining both anionic and cationic solutes, including NO3– and NH4+, and improving plant growth and N use efficiency in soil-plant systems (Mandal et al., 2019). Therefore, generating biochar from rice straw after crop harvesting is considered a practical promising management strategy of crop residue wastes to avoid the on-site burning of crop residues which is one of the key issues related to air pollution and the reduction of soil microbial community and biomass. Agegnehua et al. (2017) showed that applying biochar benefits both acidic and neutral agriculture soils.
Red soil, the predominant type of soil in south China, is used for rice production. This type of soil is typically characterized by a low productivity mainly due to poor soil properties (e.g., low pH, lack of organic C, and nutrients). Consequently, it results in increasing reliance on chemical fertilizers (especially N fertilizer) to meet the growing demand for grain production (Bouwman et al., 2013). Thus, coping with the massive rice straw and the winter fallow paddy fields along with the excessive use of N fertilizer (leads to severe N losses) are some of the key obstacles of rice-based cropping systems for farmers in south China are confronted with. Combining rice-straw biochar (RSB) with LCC residue in a rice-based cropping system can be a novel and viable management intervention that can effectively allow recycling organic materials and tackle the abovementioned problems. As a result, it may help in improving N utilization efficiency and increasing rice yield. A previous study conducted by Kimani et al. (2021) has suggested that poultry litter biochar application in combination with chemical fertilizer and/or Azolla as green manure is a promising approach to improve rice productivity, reduce N use efficiency, and minimize application of chemical fertilizer, ultimately leading to a reduced agricultural pollution and production cost. However, the effects of RSB on biomass decomposition and N release from incorporated LCC residues as well as their co-incorporation on rice yield and N use efficiency in reddish paddy soil are poorly understood. Hence, we hypothesized that (i) RSB may affect the mass decomposition and N release from LCC residue after incorporation to paddy soil, (ii) co-incorporation of RSB and LCC residue will ameliorate soil properties (e.g., inorganic N, microbial population, and biomasses), (iii) their combined application may increase yield and N use efficiency in the rice-based cropping system.
Materials and Methods
Experimental Site, Climate, and Soil
The incubation experiment was conducted at the agroecological park of Jiangxi Agricultural University (28°45′48.3′′ N, 115°50′6.5′′ E). The experimental region has a subtropical monsoon climate, characterized by heavy rain from April to June and seasonal drought from September to December. The average annual temperature is 17.8°C, and the average annual rainfall is 1545.9 mm. The annual sunshine is 1603.4 h. The mean frost-free period lasts for 276 days. The experimental soil is silt-clay derived from Quaternary red soil. The top 0–20 cm soil was collected from a paddy field, air-dried at room temperature, and then passed through a 2-mm sieve prior to laboratory analysis. Initial properties of soil were as follows: pH 5.17 (1:2.5, soil: water), soil organic carbon (SOC) 18.7 g kg–1, total nitrogen (TN) 1.09 g kg–1, available N 86.4 mg kg–1, extractable phosphorus (P) 12.8 mg kg–1, available potassium (K) 107.8 mg kg–1, and contents of clay, silt, and sand were 15.7, 51.4, and 32.9%, respectively.
Leguminous Cover Crop and Biochar
The LCC used in this study was Chinese milk vetch (Astragalus sinicus L.). Fresh LCC shoot was harvested at the full-bloom stage and chopped into about 3–5 cm pieces in length. Roots of the LCC were deliberately not included in the trials as a previous study conducted by Pan et al. (2011) has indicated that N accumulation in LCC root was 6.53 kg N ha–1, which was equivalent to 4.35% of the recommended application rate of N (150 kg ha–1). Meanwhile, we also conducted another pot experiment prior to this experiment and observed that the N accumulation in LCC roots was just 2.50–3.12 kg ha–1, which was equivalent to 1.67–2.08% of the recommended application rate of N at the experimental site (150 kg N ha–1). Hence, it was assumed that the addition of LCC roots would not result in a significant effect under these experimental conditions and was, therefore, not included in the soil. Another justification for not adding roots of the leguminous crop to the soil was that extraction of roots from a soil and then adding it to a crop are quite hectic and would not be practically possible, and was hence not included in the experiment. A subsample of fresh LCC shoot was taken for chemical analysis, which contained 405.2 g C kg–1, 32.6 g N kg–1, 3.45 g P kg–1, and 24.5 g K kg–1. The moisture content was 90.9%, and the C/N ratio was 12.4. The rice (Oryza sativa L., cv. Zhongjiazao 17) seed was provided by the School of Agronomy, Jiangxi Agricultural University, China.
The biochar was made from rice straw which was previously dried at 105°C for 24 h and milled to <2 mm. The milled rice straw was placed into ceramic crucibles and covered with close-fitting lids. Then, the straw in the ceramic crucibles was pyrolyzed under a limited oxygen supply in a muffle furnace (12D-16, Taisite Instrument Co., Ltd., Tianjina, China). The pyrolysis temperature was raised to 450°C at a rate of ∼ 20°C min–1 and then was kept for 3 h (Chun et al., 2004). The RSB was cooled overnight to room temperature and ground to pass through a 0.25 mm sieve. The characteristics of RSB were tested as follows: pH 9.41 (1:100, weight:volume), total organic carbon (TOC) 537.97 g kg–1, TN 6.13 g kg–1, total P (TP) 1.99 g kg–1, total K (TK) 27.15 g kg–1, specific area 242.24 m2 g–1, pore volume 0.14 cm3 g–1, and pore diameter 2.66 nm.
Experimental Design and Management
Experiment 1: Soil Fertility, N Uptake, and Rice Yield
A pot experiment was conducted that included five treatments with four replications, i.e., (i) control (CK, no N fertilizer, RSB, and LCC); (ii) 150 kg N ha–1 (N100); (iii) 80% N applied in conjunction with RSB (N80B), (iv) 80% N applied in conjunction with LCC residue (N80M), and (v) 80% N applied in conjunction with RSB and LCC (N80BM), respectively.
The rice seed was sown in a pot (20 cm in diameter and 25 cm in height) containing 7.5 kg air-dried soil (prepared as described above) that was used for sowing rice seed. The recommended rates of chemical fertilizers to early rice season were as follows: 150 kg N ha–1 {also equivalent to 0.10 g N kg–1 soil–1 [urea, 46.4% N], 0.05 g P2O5kg–1 soil–1 [superphosphate, 12.0% P2O5], and 0.08 g K2O kg–1 soil–1 [potassium chloride (KCl), 60% K2O]}. The N100 treatment received N fertilizer at a rate of 100% recommended N rate, while 80% N treatments received N fertilizer at a rate of 80% recommended N rate. RSB and fresh LCC shoot were applied at a rate of 8.9 g kg–1 soil–1 and 10.0 g kg–1 soil–1, respectively, for the following early rice season. All fertilizers, RSB, and fresh LCC shoot were uniformly mixed with the soil in each pot during the last 10 days of April. Tap water was used to flood the pots thereafter.
Two rice seedlings (25 days old) were transplanted into each plastic pot on 25th April. During the growing period of rice plants, the soil water layer was maintained at 3 cm depth by monitoring every day unless the soil naturally dried 20 days prior to the harvest of rice plants. At harvest maturity, all rice plants were harvested during the middle 10 days of July, and grains were manually separated from the straw of rice plants. Furthermore, no additional fertilizer was applied through the rice season in this study.
Experiment 2: Mass Decomposition and N Release From Leguminous Cover Crop Residue After Incorporation Into Paddy Soil
To study the effects of RSB on the biomass decomposition and N release from LCC shoot residue, a nylon mesh bag method was introduced into experiment 2. This experiment had two treatments (N80M and N80BM), the same as those in experiment 1 with the exception that fresh LCC residue (3–5 cm in length) was enclosed in nylon mesh bags (mesh size 38 μm, bag size 15 cm × 10 cm) and was buried below the soil surface at 10 cm depth in continuously rice-cropped pots (n = 80) before 10 days of rice seedlings transplant. Rice planting and management were the same as described in experiment 1, with four replications per treatment.
Sampling and Chemical Analysis
In experiment 1, soil samples from all pots were collected for the analysis of mineral N (NH4+ and NO3–) content at the maturity stage of rice with a soil corer (∅ 1.5 cm). In each pot, 5 soil cores were randomly collected and mixed thoroughly inside a plastic bucket to form individual bulked pot soil samples. After removing visible roots and stones, the soil samples were divided into two parts. One was sieved <0.149 mm, labeled, and then stored in plastic bags for testing the soil NH4+ and NO3– contents according to the method described previously.
The TOC and TN were measured by TOC/TN analyzer (multi N/C® 3100, Germany). Soil mineral N (i.e., NH4+ and NO3–) was extracted with 2 M KCl at a 5:1 ratio (KCl: soil, v/weight), and NH4+ and NO3– concentrations in the filtrate were analyzed by a continuous flow analyzer (AA3, Germany).
The soil microbial biomass of carbon (SMBC) and soil microbial biomass of N (SMBN) were determined by the fumigation extraction method (Brookes et al., 1985; Vance et al., 1987). In brief, fresh soil (equivalent to 25 g oven-dry weight) was weighed into 250 ml glass bottles. Three unfumigated samples from each treatment were immediately extracted with 100 ml 0.5 M K2SO4 on a rotary shaker at 220 rpm for 30 min, and then filtered through Whatman qualitative filter paper (Chantigny et al., 2006). Other three aliquots of the same samples were fumigated with alcohol-free chloroform for 24 h at 25°C. Excess chloroform was removed by repeated evacuation, and then the samples were extracted and filtered, as described previously. Soil filtrates were stored at −20°C prior to analysis for SMBC and SMBN. Organic carbon in the filtrate was determined with a TOC analyzer (Phoenix-8000, American). Total soluble N in the filtrate was followed by alkaline persulfate oxidation and measured by dual-wavelength ultraviolet spectrophotometry (Norman et al., 1985; Cabrera and Beare, 1993). Aliquots from the un-fumigated filtrates were filtered at <0.45 μm with filter membrane and used for determining soluble organic C and N using the methods described previously. Both NH4+ and NO3– contents of the un-fumigated samples were determined with a continuous flow analyzer (AA3, Germany).
Rice plants in each pot were harvested at the maturity stage and separated into two parts (i.e., straw and grain). Plant samples were weighed after de-enzyming (105°C, 30 min) and oven-drying (70°C, 24 h) to constant weight. All the dried plant samples were ground and sieved through <0.25 mm before laboratory analysis. TN in rice plant samples was determined by sulfuric acid digestion and Kjeldahl distillation. At physiological maturity, grains were separated from the straw.
In experiment 2, the LCC residues in mesh bags were collected at 0, 5, 10, 15, 20, 25, 30, 35, 50, 65, and 91 days after installation. Residues of LCC were carefully washed with tap water, oven-dried at 70°C for 24 h, and then weighed and milled <0.149 mm. Total C and N contents of LCC residue samples were analyzed using an elemental analyzer (Vario Macro Cube, Elementar Inc., Germany).
Calculations and Data Analysis
The percentage of dry matter weight (DMW) N remaining of LCC residue at a given time for each experimental unit was calculated from the following equation:
where Xr is the percentage of DMW or N remaining of LCC residue, Xt is the DMW or N mass at each sampling time t, and X0 is the initial DMW or N mass of LCC residue at 0 days.
To describe the decaying trend of LCC residue and N release, the DMW and N remaining of LCC residue were regressed over time (t) by using the first-order double exponential decay model from the following equation:
where y is the DMW or N remaining of LCC residue at the time t, k1 and k2 are the DMW decay or N release rates, and a and b are the constants.
TN accumulation (TNA) in top rice is the sum of N content of rice shoot.
where Ns is the N content in straw and Ng is the N content in grain rice plants.
Physiological efficiency of applied N fertilizer (PEN) is the ratio of the increased shoot biomass of rice plants to the N amount absorbed from fertilizer:
where Y and Y0 are the shoot biomasses of rice plants with and without N fertilizer, respectively, and U and U0 are the N accumulation in rice plant shoots with and without N fertilizer, respectively.
Values obtained from different treatments were subjected to ANOVA tests. Separation of means was performed on significant ANOVA tests by Tukey’s honestly significant difference using the SAS package (version 9.3; SAS Institute Inc., Cary, NC, United States). Statistical significance was determined at p < 0.05 unless otherwise stated. SigmaPlot 10.0 and MS Excel were used to generate the graphs and tables, respectively.
Results
Mass Decomposition and N Release From Leguminous Cover Crop Residue
More than 40% of the mass was lost and 30% of N released in 15 days after LCC residue incorporation, and thereafter both these factors slowed down (Figures 1, 2). After 91 days, only 34.4 and 37.6% of the mass, and 35.2 and 41.0% of N remained in M and biochar and treatment (BM) pots, respectively.
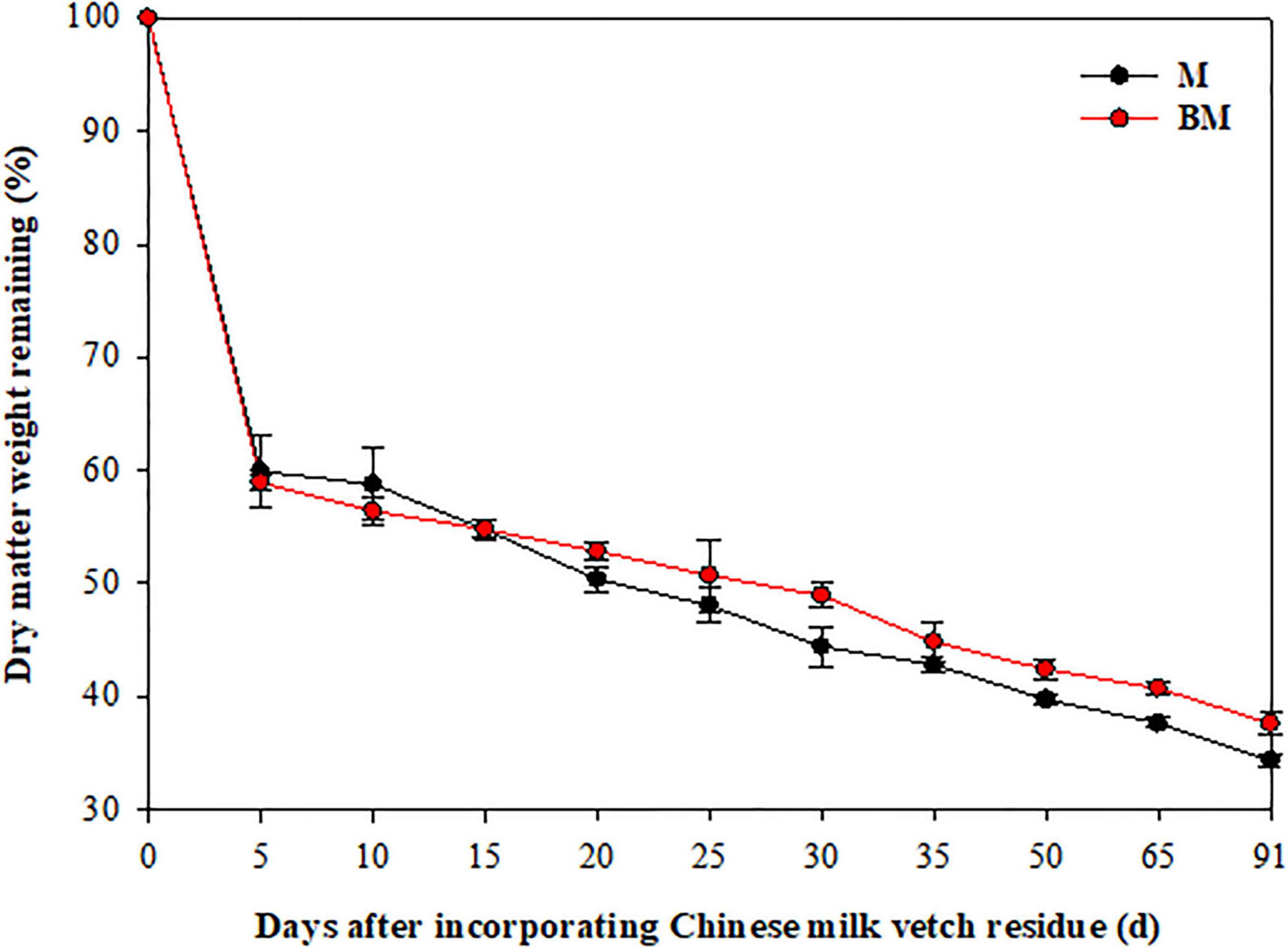
Figure 1. Dry matter decay pattern of leguminous crop after incorporation to reddish paddy soil. Small bars are standard errors.
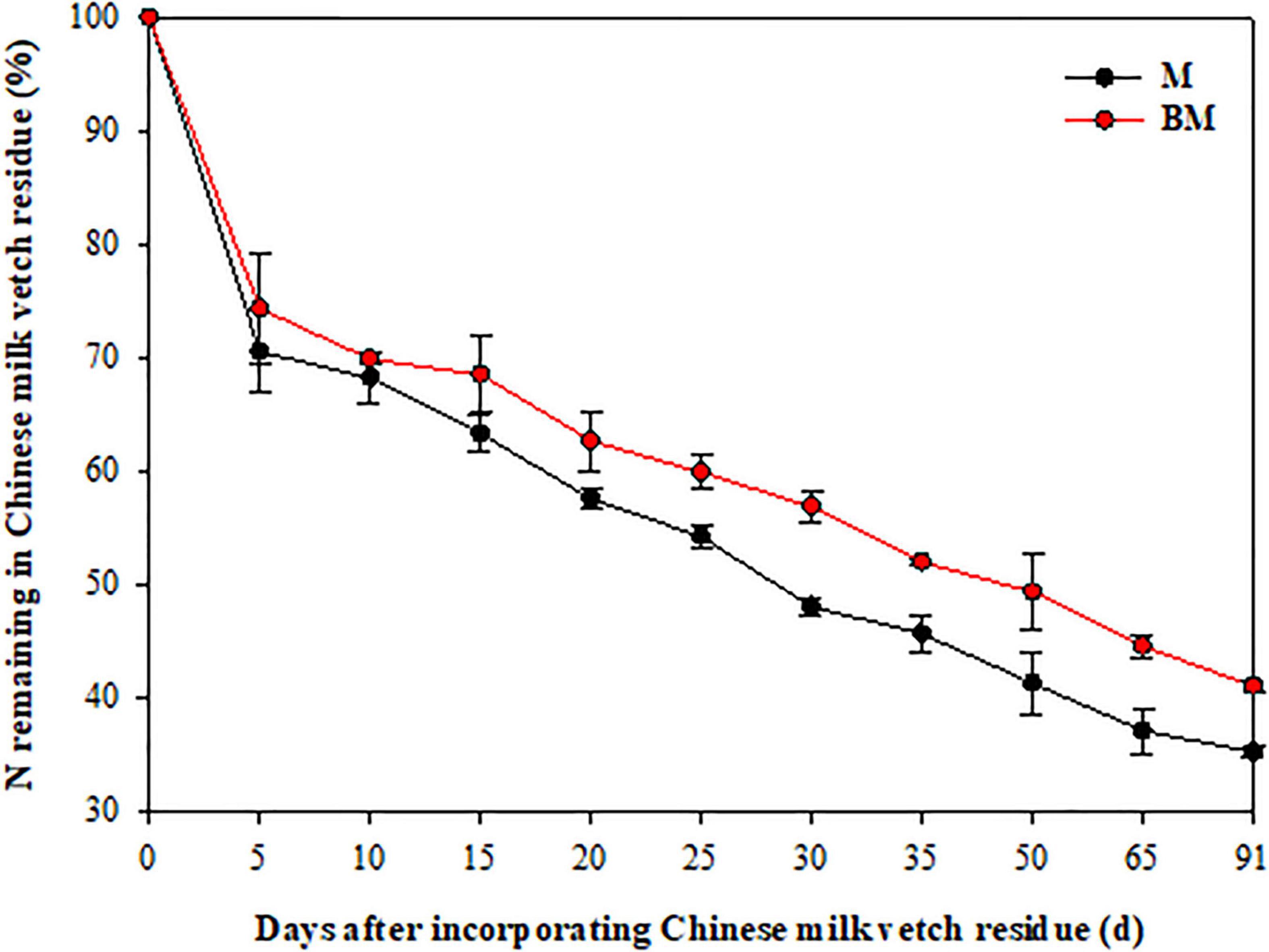
Figure 2. Nitrogen release pattern of leguminous cover crop residue after incorporation to reddish paddy soil. Small bars are standard errors.
As the two-step nature of decay dynamics, the first-order double exponential decay model was used to estimate rate constants (k) that describe the mass loss or N release from LCC residue after incorporation over time (t) (Table 1). The highest rates of mass decay (kD_1 = 0.6126) and N release (kN_1 = 0.3687) were found at relatively rapid decay or release step in BM. However, the highest mass decay rates (kD_2 = 0.0076) and highest N release rates (kN_2 = 0.0101) were found at relatively slower mass degrading and N release steps of LCC residue in case of M alone treatment (Table 1).
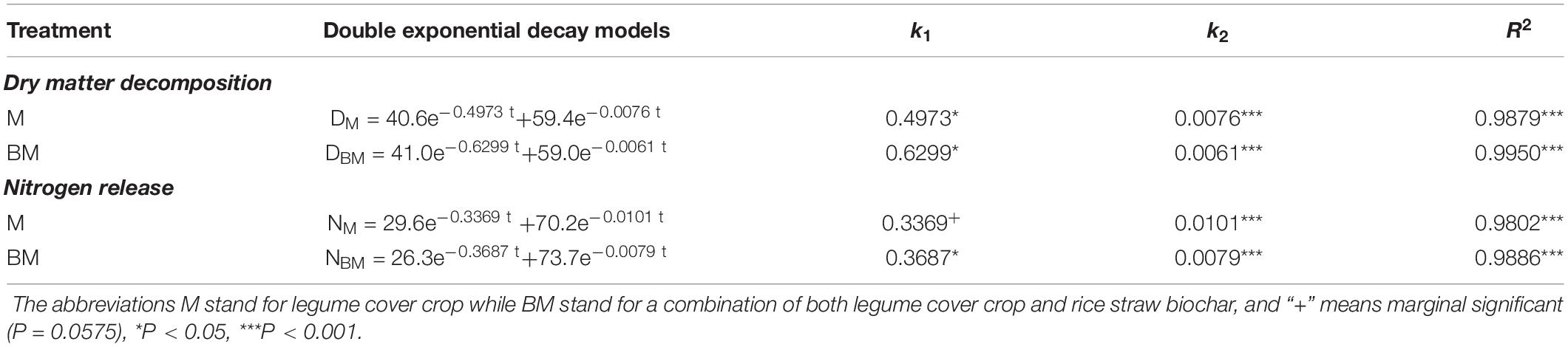
Table 1. The rate constants (k) of mass decomposition and N release for leguminous cover crop residue with or without rice straw biochar estimated by using a double exponential model.
Compared to LCC residue alone, the addition of RSB slightly stimulated the rates of mass decomposition and N release from LCC residue after incorporation into paddy soil, while it notably slowed down the rates of mass decaying and N release from LCC residue for 15 days after incorporation in case of BM treatment at relatively slower stage (Table 1 and Figures 1, 2).
Dry Matter Weight and Yield Components of Rice Plants
Incorporating RSB and/or LCC residue improved yield components and shoot biomass (Table 2). For instance, compared to 100% N, applying 80% N plus combination of RSB and LCC (N80BM) increased the 1,000-grain weight and filled grains per panicle (p > 0.05). Similarly, the number of effective panicles per plant was also markedly increased by 6.36%. Consequently, 7.11% (p < 0.05) increase in grain yield was observed. Almost similar and at par trends were observed for N80B and N80M treatments. However, additional RSB slightly suppressed the yield-increasing effect of LCC residue as green manure on rice plants mainly via decreasing the 1,000-grain weight (by ∼3.81%) and filled grains per panicle (by ∼1.25%).
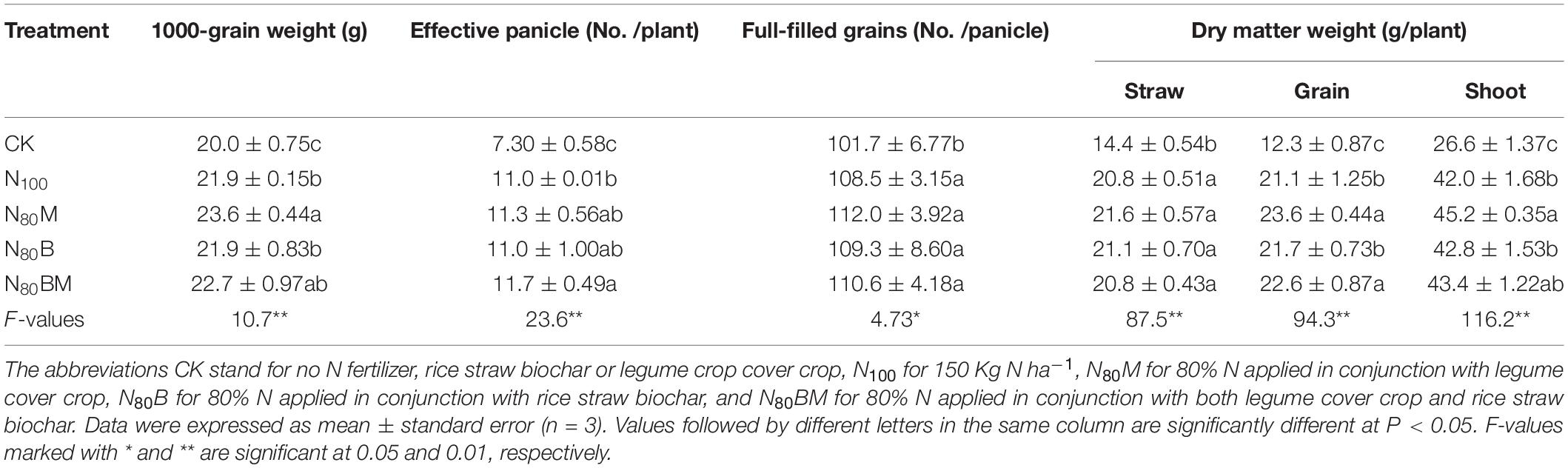
Table 2. Effects of applying rice straw biochar and/or leguminous cover crop residue on dry matter weight and yield components of rice plants.
Accumulation of N in Rice Plant Shoot
Understandably, all the fertilization treatments remarkably improved the N accumulation in rice straw and grain relative to unfertilized treatment (Figure 3). In case of applying 80% N, N accumulation in rice straw and grain did not significantly increase in N80B but applying LCC residue alone or in combination with RSB as N80M or N80BM resulted in markedly (p < 0.05) higher N accumulation in grain (by ∼13.87 and 8.96%), which eventually caused a noticeable increase in the shoot (by ∼10.09 and 10.91%), when compared with N100 treatment, respectively (Figure 3).
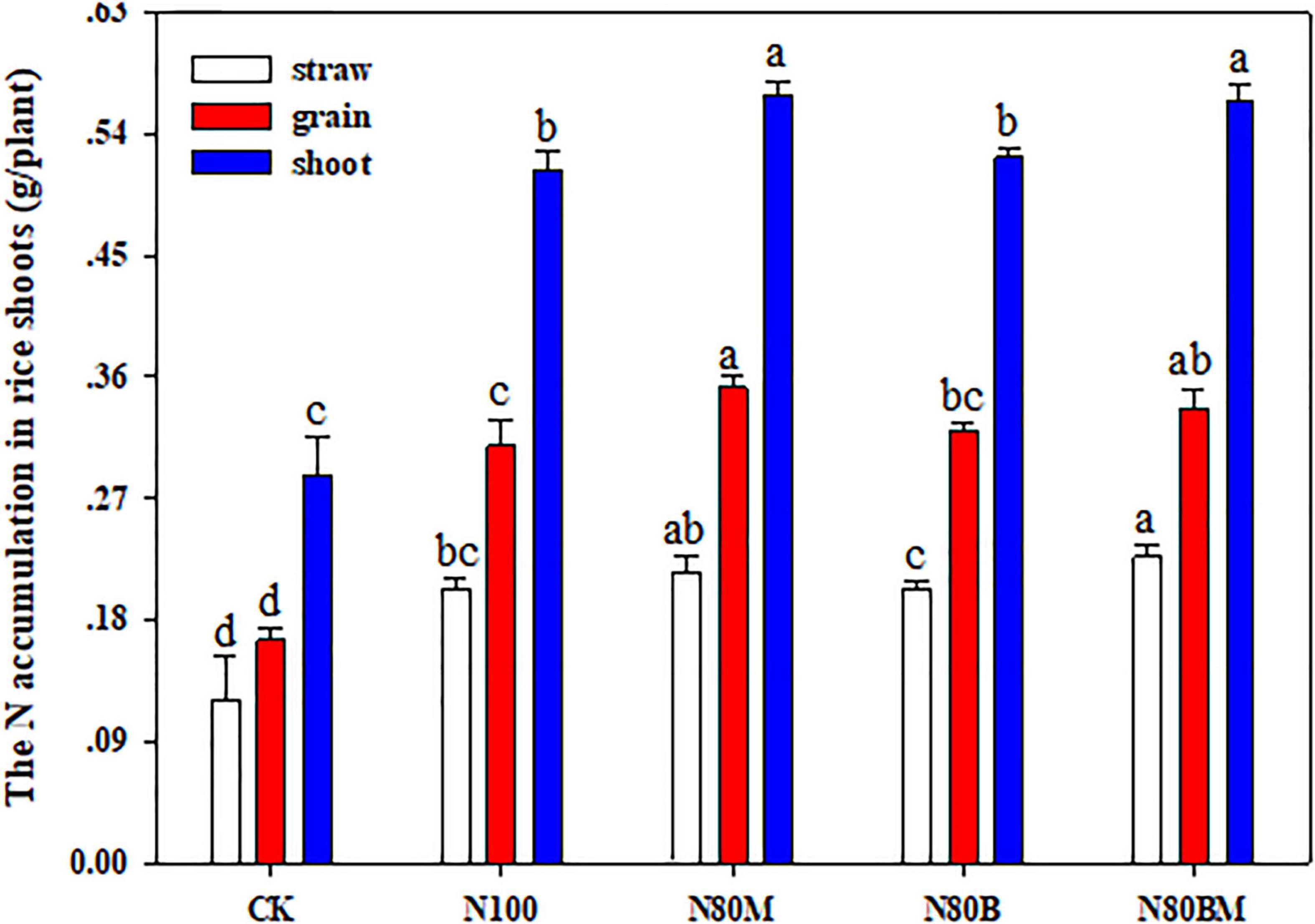
Figure 3. Effects of applying rice straw biochar and leguminous cover crop residue on the N accumulation in rice plant shoot at maturing stage. Small bars are standard errors. Values followed by different letters in the same color are significantly different at P < 0.05.
Physiological N Use Efficiency of Rice Plants
Though PEN did not prominently change in N80M pots, it was remarkably increased in the straw (by ∼28.8 and 6.80%) and grain (by ∼23.7 and 14.5%) and eventually caused a noticeable increase in the shoot of rice (by ∼25.3 and 9.75%) of N80B and N80BM pots when compared with N100, respectively (Table 3).
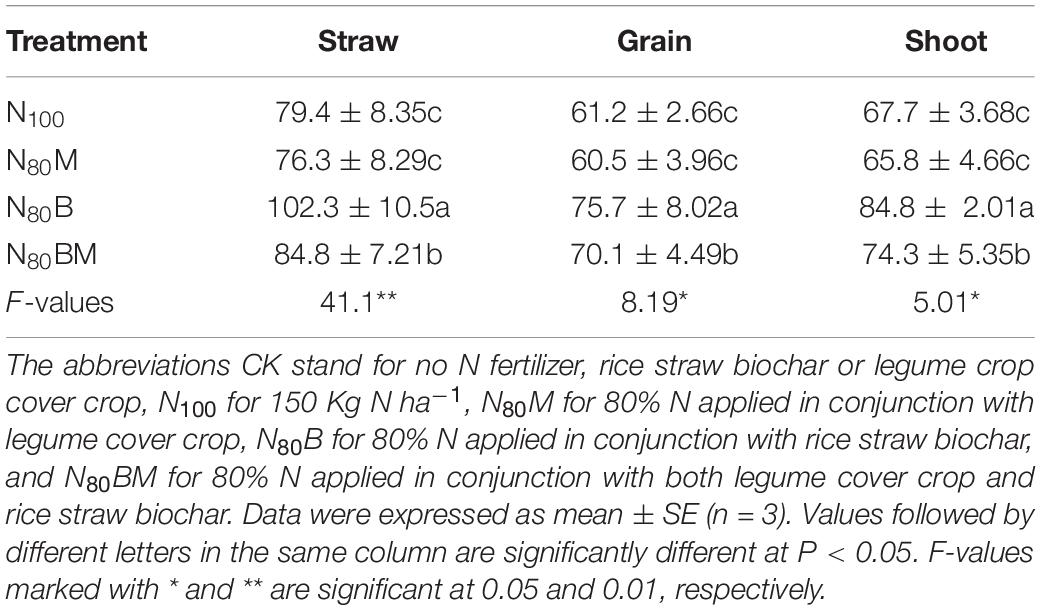
Table 3. Effects of applying rice straw biochar and/or leguminous cover crop residue on physiological N-use efficiency of rice after harvesting plants.
Soil Chemical Properties
The contents of SOM and TN in plow layer of paddy soil after harvesting rice plants clearly showed higher values in N80M, N80B, and N80BM pots, revealing greater soil fertility and N-capacity under these treatments (Table 4). Under 80% N plus additional RSB and/or LCC residue (N80M, N80B, and N80BM treatments), higher SOM of 7.06–29.4% was observed than N100 pots. Similarly, the maximum TN content in soil was observed in N80M and N80BM treatments (1.00 and 0.92 g/kg, respectively) which was remarkably higher by 17.6 and 8.24% than N100, respectively.
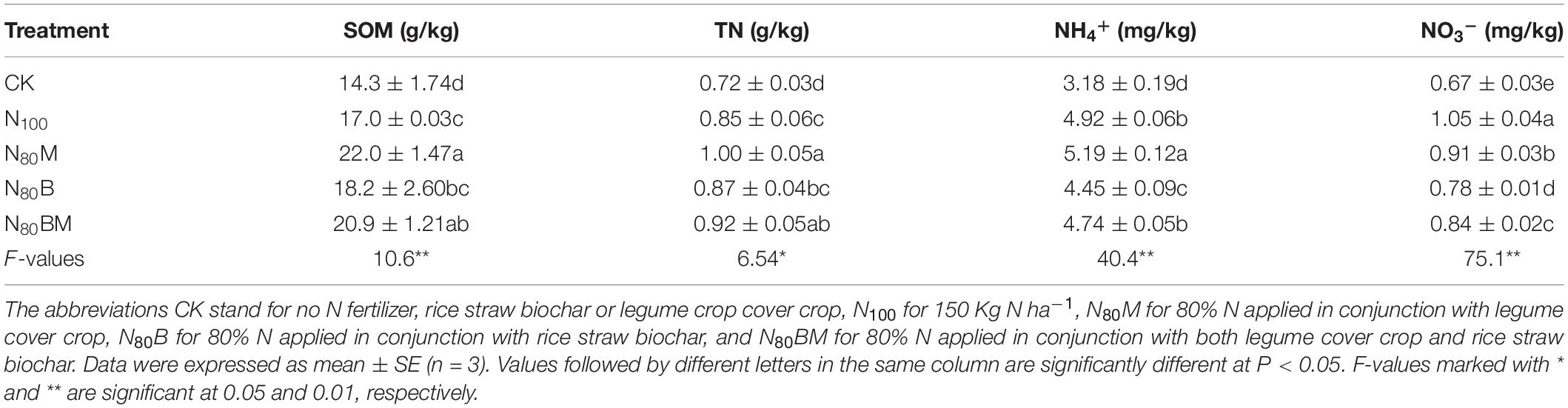
Table 4. Effects of applying rice straw biochar and/or leguminous cover crop residue on the contents of soil organic matter (SOM), total N (TN), NH4+, and NO3– after harvesting rice plants.
Compared to N100, 80% N in conjunction with LCC-only (N80M) significantly increased NH4+ content by 5.49% while decreasing NO3– content by 13.3% (Table 4). Conversely, 80% N plus additional RSB and/or LCC residue (N80B and N80BM) notably decreased NH4+ content by 9.55 and 3.66%, and NO3– content by 25.7 and 20.0% in comparison with N100, respectively (Table 4).
Soil Microbial Population and Biomasses of C and N
Additional RSB and/or LCC residue noticeably increased the SMBC and SMBN as compared to N100 (Figure 4). The obtained data illustrated that the paddy soil showed about 22.9, 12.5, and 16.3% increase in SMBC contents under RSB and/or LCC residue, respectively, when compared to N100. The SMBN contents in N80M, N80B, and N80BM were higher by 39.6, 28.0, and 35.6%, respectively, than N100.
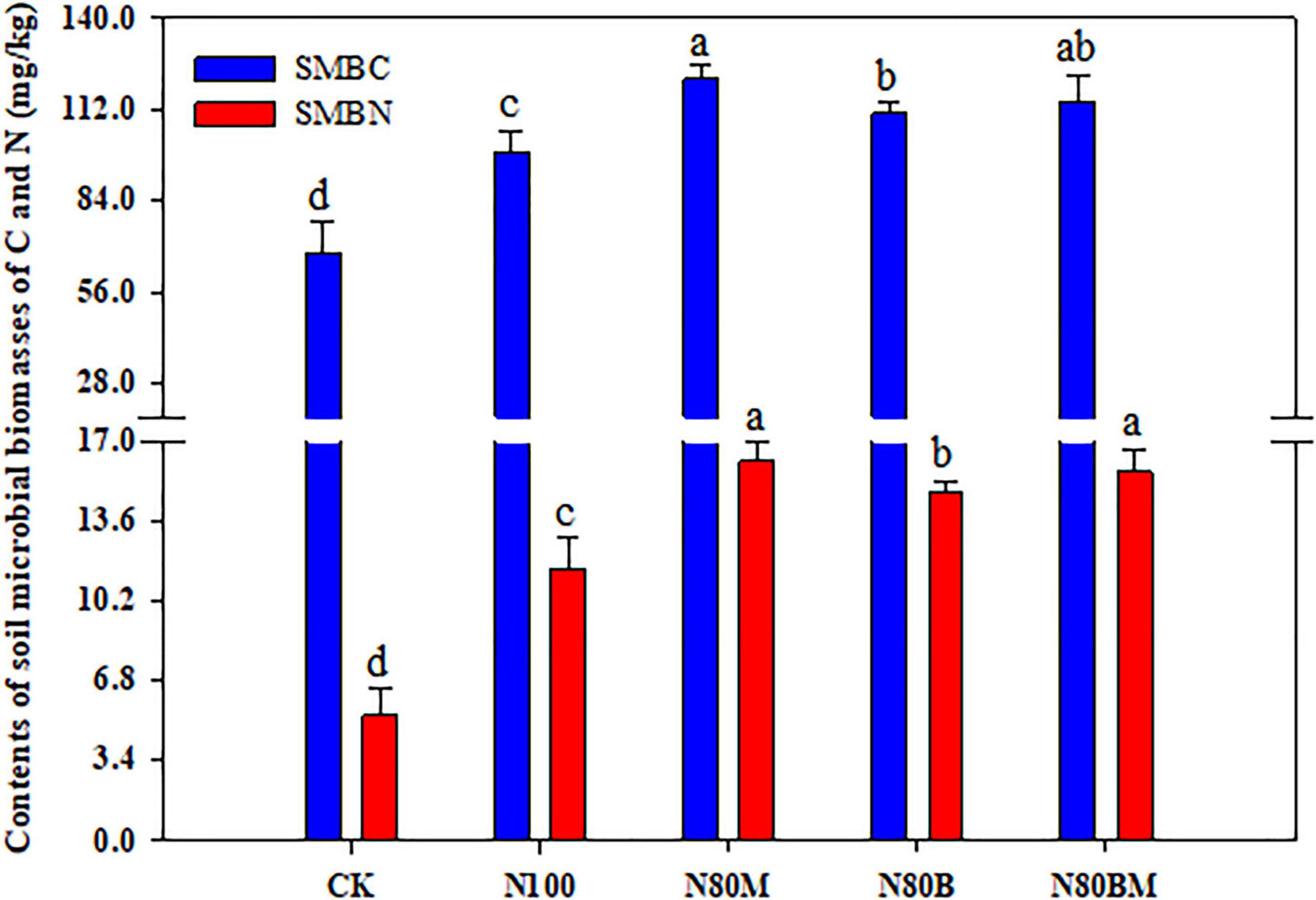
Figure 4. Effects of applying rice straw biochar and leguminous cover crop residue on soil microbial biomasses of C (SMBC) and N (SMBN) after harvesting rice plants. Small bars are standard errors. Values followed by different letters in the same color columns are significantly different at P < 0.05.
Discussion
Mass Decomposition and N Release From Leguminous Cover Crop Residues After Incorporation Into Paddy Soil
Organic residues’ decomposition determines the soil nutrients pool by the way of de-polymerization of fibers and hydrolysis of sugars, which is mainly driven by the heterotrophic soil microbes, for nutrients cycling in agroecosystems (Meisinger and Ricigliano, 2017). A series of studies have simulated organic residues decay by using a nylon mesh bagging method (Yan et al., 2019; Dhakal et al., 2020). Compared to the single exponential decay model (Zhu et al., 2014), it is better to use a double exponential function with two rate constants (k), which separated organic residue into the fast pool (e.g., amino acids) and relatively slow pool (e.g., lignin) (Berg, 1984). The initial rapid phase biomass decomposition and N release mainly occurred in the first 2 weeks after LCC residue incorporation to paddy soil (Table 1 and Figures 1, 2). A logical explanation could be that the non-structural and labile fractions of LCC residue decompose rapidly, which supplied available C and nutrients for soil microorganisms, ultimately leading to increased microbial biomasses and their activities during the rapid phase of decomposition (Zhou et al., 2020). In addition, the availability of organic C plays an important role in influencing the decay kinetics (Walela et al., 2014). The simple fractions of LCC residue are gradually decreased over time, while the recalcitrant fractions are hard to be used by soil microbes that finally led to a relatively slower rate of mass decomposition and N release from LCC residue.
Moreover, the addition of RSB did not remarkably affect the rate constants of biomass decay and N release from LCC residue in the first 2 weeks after incorporation, though it significantly slowed down the rate constants (k2) at the relatively slower stage in paddy soil (Table 1 and Figures 1, 2). These findings are in line with the results obtained by Cheng et al. (2017). It can be thus inferred that RSB with a high C/N ratio (87.8) might have constrained mass decomposition and N release from incorporated LCC residue with a low C/N ratio (12.4) at the slower stage. The high C-rich and porous biochar would cause shifts in soil microbial properties (e.g., size, activity, and structure) that alter microbial C use efficiency, leading to greater partitioning of substrate C into catabolic processes. Thus, microbes will prefer to act on the most energetically advantageous organic matter and stimulate the decomposition of simple fractions at the early stage. Meanwhile, the complex compounds that incur higher energy cost for degradation would be left till the relatively slower stage in soil (Cheng et al., 2017). In addition, both the available C and N in organic amendments and soil help the soil microbes in biomass decaying during the initial rapid phase of decomposition, while the addition of RSB remarkably decreased inorganic N (NH4+ and NO3–) contents in paddy soil after rice harvest (Table 4), which was one of the main factors that might have constrained the microbial activity, and in turn influenced the decomposition and N release from LCC residue at the relatively slower stage (Zhang et al., 2015).
Rice Yield and Its Components, Nitrogen Absorption, and Use Efficiency
Cover crops not only cover fallow soils but also contribute to producing more food without an increase in the use of chemical fertilizer (Xie et al., 2016; Yang L. et al., 2019). This is particularly true for LCC residues that provide N through biological N fixation and increase soil N supply to subsequent crops. In addition, biochar decreases the bulk density, increases electrical conductivity, available phosphorous, porosity, and water-stable aggregation (especially the macro-aggregates), and facilitates the retention of SOC and N nutrient in the soil, ensuring better conditions for plant growth, and thereby, promoting root growth and nutrients absorption of rice plants (Gao et al., 2019; Nan et al., 2020; Zhang et al., 2020; Kimani et al., 2021; Pandey et al., 2021). Application of RSB and/or LCC residue increased dry matter accumulation, N absorption, and PEN of rice shoot when compared with 100% N alone (Tables 2, 3 and Figure 3), which were consistent with the results of Wu et al. (2019) and Zhou et al. (2020). This can be explained in the light of the following four phenomena, i.e., (i) LCC residue as green manure (GM) might increase enzymatic activities (e.g., that of Superoxide Dismutase, Catalase, and Nitrate Reductase) and decrease the Malondialdehyde content in rice root (Xie et al., 2017). Meanwhile, the addition of RSB accelerates ammonia oxidation and increases root activity (Cao et al., 2019). Thus, a combined application of RSB with LCC residue would synergistically promote N uptake and use efficiency; (ii) the yield components of rice crop (i.e., effective panicles, number of full-filled grains per panicle, and 1,000-grain weight) were significantly improved in LCC and/or RSB amended pots (Table 2). Earlier, Huang et al. (2013) reported that spikelet number per panicle and grain weight exhibited positive responses to crop residue retention. Similarly, improvements in leaf area index, photosynthetic rate, and ratio of photosynthetic potential to grain have been found under the application of organic materials in addition to better root growth and development of rice plants (Zhang et al., 2020). These further strengthened the supporting system and provided enough dry matter for yield formation and nutrient absorption by rice plants. Thus, the increase in grain yield under RSB and/or LCC application could be attributed to the more vigorous plant growth at the later stage that eventually led to greater sink capacity and guaranteed the grain filling of rice plants as has also been reported by Takai et al. (2006); (iii) application of RSB and/or LCC improved soil structure such that a better microenvironment near the rhizosphere was ensured resulting in higher density, biomass, and activity of rice root that in turn facilitated N uptake and use efficiency (Zhang et al., 2020); (iv) the combined application of organic amendments with different C/N ratios regulated N release rate that could better meet the N needs of rice plants and promote N absorption and use efficiency (Zhou et al., 2020). However, LCC residue had a larger increasing effect on early rice than RSB (Table 2). It can be ascribed to the lower C/N ratio of LCC residue that probably led to rapid decomposition and N release. Conversely, RSB with a higher C/N ratio notably restricted N release from LCC residue during the relatively slower N release stage (Table 1 and Figure 2).
Soil Chemical Properties
Due to the intrinsic properties (i.e., larger surface area, micropores, and elemental compositions), the addition of biochar potentially alters the physical and chemical properties of the disturbed paddy soil (Chhatarpal et al., 2018). In comparison with 100% FN treatment, 80% N plus RSB with or without LCC residue increased the contents of SOM in soil (Table 4), which is in accordance with other studies (Yang S. H. et al., 2019; Zhang et al., 2020; Zhou et al., 2020). The increase in soil TN content could be linked to additional N from RSB (6.13 g/kg) or LCC residue (32.6 g kg−1) which was subsequently released into the soil (Song et al., 2018). In contrast, with regard to the increase in SOC, biochar could sequester native soil organic matter in its network of pores, thus reducing the degradability and mineralization of native organic matter in soil via microbial disintegration (Dungait et al., 2012). Moreover, biochar is rich in organic carbon that could form aggregates with soil particles that protect SOC from being decomposed (Amoakwah et al., 2020). Finally, biochar particles might also be integrated into the soil matrix (Zhang et al., 2020), resulting in a direct increase in the SOC content.
Furthermore, under 20% FN treatment, sole application of LCC residue remarkably increased NH4+ content while decreasing NO3– content in reddish paddy soil, further confirming the results of our previous study (Xie et al., 2016). Additional RSB with or without LCC decreased NH4+ and NO3– contents when compared with 100% N pots (Table 4), which is in agreement with the findings of Gao et al. (2019) and Martos et al. (2020). The probable mechanisms could be as follows: (i) the reduced N might have led to less available inorganic N (NH4+ and NO3–); (ii) addition of RSB and LCC facilitated N uptake and assimilation by NH4+-preferring rice plants (Figure 3) that further resulted in reducing available N substrates for nitrification and then limiting the nitrification of NH4+ to NO3– (Yang et al., 2015); (iii) addition of RSB could obtain a remarkable increase in the relative abundance of nitrifiers which in turn accelerate ammonia oxidation (Nguyen et al., 2018), and meanwhile, promote NO3– consumption by enhancing root activity and nitrate reductase activity in the roots of rice plants (Fungo et al., 2019; Sun et al., 2019); and (iv) the application of LCC residue as GM might mitigate the reduction in pH or even increase it (Li et al., 2019; Cheng et al., 2021). Conversely, the biochar elevated pH in acidic soil (Kimani et al., 2021), that in turn sharply regulated the forms of NO3– and NH4+ and promoted ammonia volatilization from soil (Hailegnaw et al., 2019; Schofield et al., 2019), that could be captured in micropores or adsorbed to the inner and exterior large oxygen-containing surface of functional groups of biochar particles (Li et al., 2018); (v) the labile C from biochar made from crop residues might induce a relatively N-limited condition and enhance microbial demand for soil N to use additional degradable C (Lehmann et al., 2003), and ultimately decrease soil inorganic N (NH4+ and NO3–). In our study, RSB with or without LCC increased MBC and MBN contents in paddy soil (Figure 4). Interestingly, the combined application of RSB and LCC residue could offset the negative impact of only RSB addition on soil inorganic N to some extent (Table 4). This might have occurred due to the net gain of N that was caused by LCC residue as GM (Xie et al., 2016); and the extra organic N input may be probably preserved by forming a biochar-organo-mineral complex which can further contribute to mineralized N in soil (Zhang et al., 2020; Yang et al., 2021). These results demonstrate that the combination of RSB with LCC residue can be a novel and promising strategy for managing rice straw and N that can effectively mitigate the agro-environmental risks associated with reddish flooded paddy fields.
Microbial Biomass of Carbon and Microbial Biomass of Nitrogen in Paddy Soil
Generally, the MBC and MBN pools in soil are regarded as suitable indicators of soil health and functions according to different fertilization strategies. Our findings indicate that reducing 20% N when coupled with the addition of RSB and/or LCC residue as GM notably increased microbial biomass C and N in paddy soil when compared with 100% N (Figure 4). Applying organic amendments (e.g., biochar and/or crop residues) increases microbial biomasses in acidic soils even under low fertility (Pokharel et al., 2020; Zhou et al., 2020). Moreover, soil pH and SOC are the main factors regulating the activities of soil enzymes. Compared with sole application of chemical fertilizers, applying organic amendments would have more positive influences on microbial biomasses as is evident from the considerable increases of SMBC and SMBN contents, soil pH, C and N dynamics, and the activities of C- and N-cycle-related enzymes that can stimulate soil microbial growth (Luo et al., 2018; Zhou et al., 2020).
Conclusion
Results of the two pot experiments showed that the addition of RSB promoted the rates of mass decomposition and N release from LCC residue after incorporation into paddy soil to some extent during the initial rapid stage of decomposition, and distinctly suppressed their rates during the relatively slower stage. A 20% reduced N application when coupled with RSB and/or LCC residue addition improved rice yield components and increased soil fertility that subsequently led to higher grain and straw yields, and N absorption and use efficiency in rice shoots. However, the addition of RSB with or without LCC residues decreased NH4+ and NO3– contents in the reddish paddy soil. Therefore, it seems logical to conclude that combining RSB with LCC residue can be a novel and promising intervention for improving soil fertility and productivity while decreasing N pollution caused by N losses in rice-based cropping systems. Future studies should further focus on the long-term and field-scale effects of combining RSB and LCC residue and their overall impact on agro-environmental sustainability of the reddish paddy ecosystems of south China. However, field-scale trials are imperative in the near future to confirm and ascertain the long-term benefits of these organic amendments.
Data Availability Statement
The original contributions presented in the study are included in the article/supplementary material, further inquiries can be directed to the corresponding author.
Author Contributions
ZX conceived and performed the experiment and drafted the manuscript. FS participated in the manuscript preparation. CZ provided the facilities. All authors contributed to the article and approved the submitted version.
Funding
This work was supported by the National Natural Science Foundation of China (32060727), National Key R&D Program of China (2017YFD0200808), Natural Science Foundation of Jiangxi Province, China (2020BAB203018), and Science and Technology Project of Department of Education of Jiangxi Province, China (GJJ180170).
Conflict of Interest
The authors declare that the research was conducted in the absence of any commercial or financial relationships that could be construed as a potential conflict of interest.
Publisher’s Note
All claims expressed in this article are solely those of the authors and do not necessarily represent those of their affiliated organizations, or those of the publisher, the editors and the reviewers. Any product that may be evaluated in this article, or claim that may be made by its manufacturer, is not guaranteed or endorsed by the publisher.
References
Agegnehua, G., Srivastavab, A., and Michael, I. B. (2017). The role of biochar and biochar-compost in improving soil quality and crop performance: a review. Appl. Soil Ecol. 119, 156–170. doi: 10.1016/j.apsoil.2017.06.008
Amoakwah, E., Arthur, E., Frimpong, K. A., Parikh, S. J., and Islam, R. (2020). Soil organic carbon storage and quality are impacted by corn cob biochar application on a tropical sandy loam. J. Soil Sediment 20, 1960–1969. doi: 10.1007/s11368-019-02547-5
Berg, B. (1984). Decomposition of root litter and some factors regulating the process: Long-term root litter decomposition in a Scots pine forest. Soil Biol. Biochem. 16, 609–617.
Bouwman, L., Goldewijk, K. K., Van Der Hoek, K. W., Beusen, A. H., Van Vuuren, D. P., Willems, J., et al. (2013). Exploring global changes in nitrogen and phosphorus cycles in agriculture induced by livestock production over the 1900-2050 period. Proc. Natl. Acad. Sci. 110, 20882–20887. doi: 10.1073/pnas.1012878108
Brookes, P. C., Landman, A., Pruden, G., and Jenkinson, D. S. (1985). Chloroform fumigation and the release of soil-nitrogen-a rapid direct extraction method to measure microbial biomass nitrogen in soil. Soil Biol. Biochem. 17, 837–842. doi: 10.1016/0038-0717(85)90144-0
Cabrera, M. L., and Beare, M. H. (1993). Alkaline persulfate oxidation for determining total nitrogen in microbial biomass extracts. Soil Sci. Soc. Am. J. 57, 1007–1012. 5995005700040021x doi: 10.2136/sssaj1993.0361
Cai, S. Y., Pittelkow, C. M., Zhao, X., and Wang, S. Q. (2018). Winter legume-rice rotations can reduce nitrogen pollution and carbon footprint while maintaining net ecosystem economic benefits. J. Clean. Prod. 195, 289–300. doi: 10.1016/j.jclepro.2018.05.115
Cao, H., Ning, L. F., Xun, M., Feng, F., Li, P., Yue, S. Q., et al. (2019). Biochar can increase nitrogen use efficiency of Malus hupehensis by modulating nitrate reduction of soil and root. Appl. Soil Ecol 135, 25–32. 11.002 doi: 10.1016/j.apsoil.2018
Chantigny, M. H., Angers, D. A., Kaiser, K., and Kalbitz, K. (2006). “Extraction and characterization of dissolved organic matter,” in Soil Sampling and Methods of Analysis, eds M. R. Carter and E. G. Gregorich (Canada: Taylor & Francis Group, LLC), 617–635.
Cheng, H. G., Hill, P. W., Bastami, M. S., and Jones, D. L. (2017). Biochar stimulates the decomposition of simple organic matter and suppresses the decomposition of complex organic matter in a sandy loam soil. GCB Bioenergy 9, 1110–1121. doi: 10.1111/gcbb.12402
Cheng, S. H., Zhuang, J. Y., Fan, Y. Y., Du, J. Y., and Cao, L. Y. (2007). Progress in research and development on hybrid rice: a super-domesticate in China. Ann. Bot. 100, 959–966. doi: 10.1093/aob/mcm121
Cheng, Y., Zhang, H., Chen, Z., Wang, J., Cai, Z., Sun, N., et al. (2021). Contrasting effects of different pH-raising materials on N2O emissions in acidic upland soils. Eur. J. Soil. Sci. 72, 432–445. doi: 10.1111/ejss.12964
Chhatarpal, S., Shashank, T., Kumar, G. V., and Shankar, S. J. (2018). The effect of rice husk biochar on soil nutrient status, microbial biomass and paddy productivity of nutrient poor agriculture soils. Catena 171, 485–493. doi: 10.1016/j.catena.2018.07.042
Chun, Y., Sheng, G. Y., Chiou, C. T., and Xing, B. S. (2004). Compositions and sorptive properties of crop residue-derived chars. Environ. Sci. Technol. 38, 4649–4655. doi: 10.1021/es035034w
Dhakal, M., Singh, G., Cook, R. L., and Sievers, T. (2020). Modeling hairy vetch and cereal rye cover crop decomposition and nitrogen release. Agronomy 10:701. 10050701 doi: 10.3390/agronomy
Ding, W. C., Xu, X. P., He, P., Ullah, S., Zhang, J. J., Cui, Z. L., et al. (2018). Improving yield and nitrogen use efficiency through alternative fertilization options for rice in China: a meta-analysis. Field Crop Res. 227, 11–18. doi: 10.1016/j.fcr.2018.08.001
Dungait, J. A. J., Hopkins, D. W., Gregory, A. S., and Whitmore, A. P. (2012). Soil organic matter turnover is governed by accessibility not recalcitrance. Glob. Chang. Biol. 18, 1781–1796. doi: 10.1111/j.1365-2486.2012.02665.x
Fungo, B., Chen, Z., Butterbach-Bahl, K., Lehmannn, J., Saiz, G., Braojos, V., et al. (2019). Nitrogen turnover and N2O/N2 ratio of three contrasting tropical soils amended with biochar. Geoderma 348, 12–20. doi: 10.1016/j.geoderma.2019.04.007
Gao, S., DeLuca, T. H., and Cleveland, C. C. (2019). Biochar additions alter phosphorus and nitrogen availability in agricultural ecosystems: a meta-analysis. Sci. Total Environ. 654, 463–472. doi: 10.1016/j.scitotenv.2018.11.124
Hailegnaw, N. S., Mercl, F., Pračke, K., Száková, J., and Tlustoš, P. (2019). High temperature-produced biochar can be efficient in nitrate loss prevention and carbon sequestration. Geoderma 338, 48–55. doi: 10.1016/j.geoderma.2018.11.006
He, H. B., Li, W. X., Zhang, Y. W., Cheng, J. K., Jia, X. Y., Li, S., et al. (2020). Effects of Italian ryegrass residues as green manure on soil properties and bacterial communities under an Italian ryegrass (Lolium multiflorum L.)-rice (Oryza sativa L.) rotation. Soil Till. Res. 196:104487. doi: 10.1016/j.still.2019.104487
Huang, S., Zeng, Y. J., Wu, J. F., Shi, Q. H., and Pan, X. H. (2013). Effect of crop residue retention on rice yield in China: a meta-analysis. Field Crop Res. 154, 188–194. doi: 10.1016/j.fcr.2013.08.013
Jiang, Y., Qian, H. Y., Huang, S., Zhang, X. Y., Wang, L., Zhang, L., et al. (2019). Acclimation of methane emissions from rice paddy fields to straw addition. Sci. Adv. 5:eaau9038. doi: 10.1126/sciadv.aau9038
Jiang, Y. F., Rao, L., Sun, K., Han, Y., and Guo, X. (2018). Spatio-temporal distribution of soil nitrogen in Poyang lake ecological economic zone (South-China). Sci. Total Environ. 626, 235–243. doi: 10.1016/j.scitotenv.2018.01.087
Kimani, S. M., Bimantara, P. O., Kautsar, V., Tawaraya, K., and Cheng, W. G. (2021). Poultry litter biochar application in combination with chemical fertilizer and Azolla green manure improves rice grain yield and nitrogen use efficiency in paddy soil. Biochar 3, 591–602. doi: 10.1007/s42773-021-00116-z
Lehmann, J. da Silva Jr., J.P., Steiner, C., Nehls, T., Zech, W., and Glaser, B. (2003). Nutrient availability and leaching in an archaeological Anthrosol and a Ferralsol of the Central Amazon basin: fertilizer, manure and charcoal amendments. Plant Soil 249, 343–357. doi: 10.1023/A:1022833116184
Lehmann, J., and Joseph, S. (2009). “Biochar for Environmental management: an introduction,” in Biochar for Environmental Management: Science and Technology, eds J. Lehmann and S. Joseph (London: Earthscan), 1–12.
Li, S. M., Barreto, V., Li, R. W., Chen, G., and Hsieh, Y. P. (2018). Nitrogen retention of biochar derived from different feedstocks at variable pyrolysis temperatures. J. Anal. Appl. Pyrol. 133, 136–146. doi: 10.1016/j.jaap.2018.04.010
Li, T., Gao, J. S., Bai, L. Y., Wang, Y. N., Huang, J., Kumar, M., et al. (2019). Influence of green manure and rice straw management on soil organic carbon, enzyme activities, and rice yield in red paddy soil. Soil Till. Res. 195:104428. doi: 10.1016/j.still.2019.104428
Liu, J., Jiang, B. S., Shen, J. L., Zhu, X., Yi, W. Y., Li, Y., et al. (2021). Contrasting effects of straw and straw-derived biochar applications on soil carbon accumulation and nitrogen use efficiency in double-rice cropping systems. Agr. Ecosyst. Environ. 311:107286. doi: 10.1016/j.agee
Luo, G. W., Li, L., Friman, V. P., Guo, J. J., Guo, S. W., Shen, Q. R., et al. (2018). Organic amendments increase crop yields by improving microbe-mediated soil functioning of agroecosystems: a meta-analysis. Soil Biol. Biochem. 124, 105–115.
Mandal, S., Donner, E., Smith, E., Sarkar, B., and Lombi, E. (2019). Biochar with near-neutral pH reduces ammonia volatilization and improves plant growth in a soil-plant system: A closed chamber experiment. Sci. Total Environ. 697:134114. doi: 10.1016/j.scitotenv.2019.134114
Martos, S., Mattana, S., Ribas, A., Albanell, E., and Domene, X. (2020). Biochar application as a win-win strategy to mitigate soil nitrate pollution without compromising crop yields: a case study in a Mediterranean calcareous soil. J. Soil Sediment. 20, 220–233. doi: 10.1007/s11368-019-02400-9
Meisinger, J. J., and Ricigliano, K. A. (2017). Nitrate leaching from winter cereal cover crops using undisturbed soil-column lysimetersle. J. Environ. Qual. 46, 576–584. 09.0372 doi: 10.2134/jeq2016
Nan, Q., Wang, C., Wang, H., Yi, Q. Q., Liang, B. Q., Xu, J., et al. (2020). Biochar drives microbially-mediated rice production by increasing soil carbon. J. Hazard. Mater. 387:121680. doi: 10.1016/j.jhazmat.2019.121680
Nguyen, T. T. N., Wallace, H. M., Xu, C. Y., Zwieten, L. V., Weng, Z. H., Xu, Z. H., et al. (2018). The effects of short term, long term and reapplication of biochar on soil bacteria. Sci. Total Environ. 636, 142–151. doi: 10.1016/j.scitotenv.2018.04.278
Norman, R. J., Edberg, J. C., and Stucki, J. W. (1985). Determination of nitrate in soil extracts by dual-wavelength ultraviolet spectrophotometry. Soil Sci. Soc. Am. J 49, 1182–1185. doi: 10.2136/sssaj1985.03615995004900050022x
Pan, F. X., Li, X. K., Lu, J. W., Lu, J. M., Liu, W., Wei, Y. X., et al. (2011). Effect of seeding rate on growth and nutrient accumulation of Chinese milk vetch. Chin. J. Eco-Agric 19, 574–578. doi: 10.3724/SP.J.1011.2011.00574
Pandey, B. K., Huang, G. Q., Bhosale, R., Hartman, S., Sturrock, C. J., Jose, L., et al. (2021). Plant roots sense soil compaction through restricted ethylene diffusion. Science 371, 276–280. doi: 10.1126/science.abf3013
Poeplau, C., Helena, A. H., and Myrbeck, Å, and Kätterer, T. (2015). Effect of perennial ryegrass cover crop on soil organic carbon stocks in southern Sweden. Geoderma. Reg. 4, 126–133. geodrs.2015.01.004 doi: 10.1016/j
Pokharel, P., Ma, Z. L., and Chang, S. X. (2020). Biochar increases soil microbial biomass with changes in extra-and intracellular enzyme activities: a global meta-analysis. Biochar 2, 65–79. doi: 10.1007/s42773-020-00039-1
Schofield, H. K., Pettitt, T. R., Tappin, A. D., Rollinson, G. K., and Fitzsimons, M. F. (2019). Biochar incorporation increased nitrogen and carbon retention in a waste-derived soil. Sci. Total Environ. 690, 1228–1236. doi: 10.1016/j.scitotenv.2019.07.116
Song, D. L., Tang, J. W., Xi, X. Y., Zhang, S. Q., Liang, G. Q., Zhou, W., et al. (2018). Responses of soil nutrients and microbial activities to additions of maize straw biochar and chemical fertilization in a calcareous soil. Eur. J. Soil Biol. 84, 1–10. doi: 10.1016/j.ejsobi.2017.11.003
Sun, X., Zhong, T., Zhang, L., Zhang, K. S., and Wu, W. X. (2019). Reducing ammonia volatilization from paddy field with rice straw derived biochar. Sci. Total Environ. 660, 512–518. doi: 10.1016/j.scitotenv.2018.12.450
Takai, T., Matsuura, S., Nishio, T., Ohsumi, A., Shiraiwa, T., and Horie, T. (2006). Rice yield potential is closely related to crop growth rate during late reproductive period. Field Crop Res. 96, 328–335. doi: 10.1016/j.fcr.2005.08.001
Tang, D., and Cheng, Z. (2019). From basic research to molecular breeding-Chinese scientists play a central role in boosting world rice production. Genom. Proteom. Bioinf. 16, 389–392. doi: 10.1016/j.gpb.2018.12.002
Vance, E. D., Brookes, P. C., and Jenkinson, D. S. (1987). An extraction method for measuring soil microbial biomass-C. Soil Biol. Biochem. 19, 703–707. doi: 10.1016/0038-0717(87)90052-6
Walela, C., Daniel, H., Wilson, B., Lockwood, P., Cowie, A., and Harden, S. (2014). The initial lignin: nitrogen ratio of litter from above and below ground sources strongly and negatively influenced decay rates of slowly decomposing litter carbon pools. Soil Biol. Biochem. 77, 268–275. doi: 10.1016/j.soilbio.2014.06.013
Wu, D., Feng, Y. F., Xue, L. H., Liu, M. Q., Yang, B., Hu, F., et al. (2019). Biochar combined with vermicompost increases crop production while reducing ammonia and nitrous oxide emissions from a paddy soil. Pedosphere 29, 82–94. doi: 10.1016/S1002-0160(18)60050-5
Xie, Z. J., He, Y. Q., Tu, S. X., Xu, C. X., Liu, G. R., Wang, H. M., et al. (2017). Chinese milk vetch improves plant growth, development and 15N recovery in the rice-based rotation system of south China. Sci. Rep. UK. 7:3577. doi: 10.1038/s41598-017-03919-y
Xie, Z. J., Tu, S. X., Shah, F., Xu, C. X., Chen, J. R., Han, D., et al. (2016). Substitution of fertilizer-N by green manure improves the sustainability of yield in double-rice cropping system in south China. Field Crop Res. 188, 142–149. doi: 10.1016/j.fcr.2016.01.006
Yan, C., Yan, S. S., Jia, T. Y., Dong, S. K., Ma, C. M., and Gong, Z. P. (2019). Decomposition characteristics of rice straw returned to the soil in northeast China. Nutr. Cycl. Agroecosys. 114, 211–224. doi: 10.1007/s10705-019-09999-8
Yang, F., Cao, X. D., Gao, B., Zhao, L., and Li, F. Y. (2015). Short-term effects of rice straw biochar on sorption, emission, and transformation of soil NH4+-N. Environ. Sci. Pollut. Res. 22, 9184–9192. doi: 10.1007/s11356-014-4067-1
Yang, F., Xu, Z. B., Huang, Y. D., Tsang, D. C. W., Ok, Y. S., Zhao, L., et al. (2021). Stabilization of dissolvable biochar by soil minerals: release reduction and organo-mineral complexes formation. J. Hazard. Mat. 412:125213. doi: 10.1016/j.jhazmat.2021.125213
Yang, L., Zhou, X., Liao, Y. L., Lu, Y. H., Nie, J., and Cao, W. D. (2019). Co-incorporation of Rice Straw and green manure benefits rice yield and nutrient uptake. Crop Sci. 59, 749–759. doi: 10.2135/cropsci2018.07.0427
Yang, S. H., Sun, X., Ding, J., Jiang, Z. W., and Xu, J. Z. (2019). Effects of biochar addition on the NEE and soil organic carbon content of paddy fields under water-saving irrigation. Environ. Sci. Pollut. R. 26, 8303–8311. doi: 10.1007/s11356-019-04326-8
Zhang, H., Voroney, R. P., and Price, G. W. (2015). Effects of temperature and processing conditions on biochar chemical properties and their influence on soil C and N transformations. Soil Biol. Biochem. 83, 19–28. doi: 10.1016/j.soilbio.2015.01.006
Zhang, Q. Q., Song, Y. F., Wu, Z., Yan, X. Y., Gunina, A., Kuzyakov, Y., et al. (2020). Effects of six-year biochar amendment on soil aggregation, crop growth, and nitrogen and phosphorus use efficiencies in a rice-wheat rotation. J. Clean. Prod. 242:118435. doi: 10.1016/j.jclepro.2019.118435
Zhou, G. P., Cao, W. D., Bai, J. S., Xu, C. C., Zeng, N. H., Gao, S. J., et al. (2019). Non-additive responses of soil C and N to rice straw and hairy vetch (Vicia villosa Roth L.) mixtures in a paddy soil. Plant Soil 436, 229–244. doi: 10.1007/s11104-018-03926-6
Zhou, G. P., Gao, S. J., Lu, Y. H., Liao, Y. L., Nie, J., and Cao, W. D. (2020). Co-incorporation of green manure and rice straw improves rice production, soil chemical, biochemical and microbiological properties in a typical paddy field in southern China. Soil Till. Res. 197:104499. doi: 10.1016/j.still.2019.104499
Keywords: rice-straw biochar, leguminous cover crop, soil microbial biomass, rice grain yield, combining effects
Citation: Xie Z, Shah F and Zhou C (2022) Combining Rice Straw Biochar With Leguminous Cover Crop as Green Manure and Mineral Fertilizer Enhances Soil Microbial Biomass and Rice Yield in South China. Front. Plant Sci. 13:778738. doi: 10.3389/fpls.2022.778738
Received: 17 September 2021; Accepted: 03 March 2022;
Published: 25 April 2022.
Edited by:
Marta Wilton Vasconcelos, Catholic University of Portugal, PortugalCopyright © 2022 Xie, Shah and Zhou. This is an open-access article distributed under the terms of the Creative Commons Attribution License (CC BY). The use, distribution or reproduction in other forums is permitted, provided the original author(s) and the copyright owner(s) are credited and that the original publication in this journal is cited, in accordance with accepted academic practice. No use, distribution or reproduction is permitted which does not comply with these terms.
*Correspondence: Zhijian Xie, zjxie@jxau.edu.cn; hoblecat@126.com