The Association Between Vascular Inflammation and Depressive Disorder. Causality, Biomarkers and Targeted Treatment
Abstract
: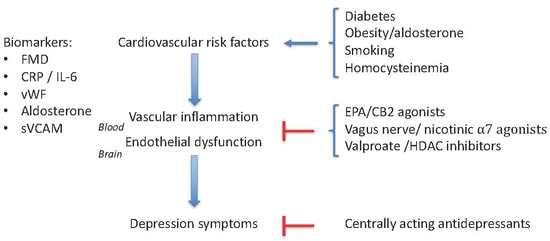
Graphical Abstract
1. Introduction
2. Causes of Vascular Inflammation
2.1. Comorbidity Between Diabetes and Depression
2.2. Comorbidity Between Female Obesity and Depression
2.3. Aldosterone as a Risk Factor for Depression
2.4. Old Age as a Risk Factor for Depression
2.5. Homocysteine and Vascular Inflammation
2.6. The Endothelium of the Blood-Brain Barrier
3. Biomarkers for Vascular Inflammation
3.1. Flow-Mediated Dilation
3.2. Circulating Biomarkers
4. Treatments
4.1. The Fish-Oil Component Eicosapentaenoic Acid (EPA)
4.2. CB2-Receptor Agonists
4.3. Vagus Nerve Stimulation
4.4. Histone Deacetylase inhibition
5. Discussion
Funding
Acknowledgments
Conflicts of Interest
List of Abbreviations
References
- Littrell, J.L. Taking the perspective that a depressive state reflects inflammation: Implications for the use of antidepressants. Front. Psychol. 2012, 3, 297. [Google Scholar] [CrossRef] [Green Version]
- Benros, M.E.; Waltoft, B.L.; Nordentoft, M.; Ostergaard, S.D.; Eaton, W.W.; Krogh, J.; Mortensen, P.B. Autoimmune diseases and severe infections as risk factors for mood disorders: A nationwide study. JAMA Psychiatry 2013, 70, 812–820. [Google Scholar] [CrossRef] [PubMed] [Green Version]
- Berk, M.; Williams, L.J.; Jacka, F.N.; O’Neil, A.; Pasco, J.A.; Moylan, S.; Allen, N.B.; Stuart, A.L.; Hayley, A.C.; Byrne, M.L.; et al. So depression is an inflammatory disease, but where does the inflammation come from? BMC Med. 2013, 11, 200. [Google Scholar] [CrossRef] [PubMed] [Green Version]
- Lotrich, F.E. Inflammatory cytokine-associated depression. Brain. Res. 2015, 1617, 113–125. [Google Scholar] [CrossRef] [PubMed] [Green Version]
- Zalli, A.; Jovanova, O.; Hoogendijk, W.J.; Tiemeier, H.; Carvalho, L.A. Low-grade inflammation predicts persistence of depressive symptoms. Psychopharmacology (Berl.) 2016, 233, 1669–1678. [Google Scholar] [CrossRef] [Green Version]
- Vogelzangs, N.; De Jonge, P.; Smit, J.H.; Bahn, S.; Penninx, B.W. Cytokine production capacity in depression and anxiety. Transl. Psychiatry 2016, 6, e825. [Google Scholar] [CrossRef]
- Young, J.J.; Bruno, D.; Pomara, N. A review of the relationship between proinflammatory cytokines and major depressive disorder. J. Affect. Disord. 2014, 169, 15–20. [Google Scholar] [CrossRef]
- Hodes, G.E.; Menard, C.; Russo, S.J. Integrating interleukin-6 into depression diagnosis and treatment. Neurobiol. Stress 2016, 4, 15–22. [Google Scholar] [CrossRef] [Green Version]
- Kohler, C.A.; Freitas, T.H.; Stubbs, B.; Maes, M.; Solmi, M.; Veronese, N.; De Andrade, N.Q.; Morris, G.; Fernandes, B.S.; Brunoni, A.R.; et al. Peripheral alterations in cytokine and chemokine levels after antidepressant drug treatment for major depressive disorder: Systematic review and meta-analysis. Mol. Neurobiol. 2018, 55, 4195–4206. [Google Scholar] [CrossRef] [Green Version]
- Boorman, E.; Zajkowska, Z.; Ahmed, R.; Pariante, C.M.; Zunszain, P.A. Crosstalk between endocannabinoid and immune systems: A potential dysregulation in depression? Psychopharmacology (Berl.) 2016, 233, 1591–1604. [Google Scholar] [CrossRef] [Green Version]
- Khandaker, G.M.; Dantzer, R.; Jones, P.B. Immunopsychiatry: Important facts. Psychol. Med. 2017, 47, 2229–2237. [Google Scholar] [CrossRef] [Green Version]
- Holzer, P.; Farzi, A.; Hassan, A.M.; Zenz, G.; Jacan, A.; Reichmann, F. Visceral inflammation and immune activation stress the brain. Front. Immunol. 2017, 8, 1613. [Google Scholar] [CrossRef] [PubMed] [Green Version]
- Baruah, J.; Vasudevan, A. The vessels shaping mental health or illness. Open Neurol. J. 2019, 13, 1–9. [Google Scholar] [CrossRef] [PubMed] [Green Version]
- Tiemeier, H.; Van Dijck, W.; Hofman, A.; Witteman, J.C.; Stijnen, T.; Breteler, M.M. Relationship between atherosclerosis and late-life depression: The rotterdam study. Arch. Gen. Psychiatry 2004, 61, 369–376. [Google Scholar] [CrossRef] [PubMed] [Green Version]
- Chen, S.; Zhang, Q.; Dai, G.; Hu, J.; Zhu, C.; Su, L.; Wu, X. Association of depression with pre-diabetes, undiagnosed diabetes, and previously diagnosed diabetes: A meta-analysis. Endocrine 2016, 53, 35–46. [Google Scholar] [CrossRef]
- Chireh, B.; Li, M.; D’Arcy, C. Diabetes increases the risk of depression: A systematic review, meta-analysis and estimates of population attributable fractions based on prospective studies. Prev. Med. Rep. 2019, 14, 100822. [Google Scholar] [CrossRef]
- Gan, Y.; Gong, Y.; Tong, X.; Sun, H.; Cong, Y.; Dong, X.; Wang, Y.; Xu, X.; Yin, X.; Deng, J.; et al. Depression and the risk of coronary heart disease: A meta-analysis of prospective cohort studies. BMC Psychiatry 2014, 14, 371. [Google Scholar] [CrossRef] [Green Version]
- Carney, R.M.; Freedland, K.E. Depression and coronary heart disease. Nat. Rev. Cardiol. 2017, 14, 145–155. [Google Scholar] [CrossRef]
- Lauzon, C.; Beck, C.A.; Huynh, T.; Dion, D.; Racine, N.; Carignan, S.; Diodati, J.G.; Charbonneau, F.; Dupuis, R.; Pilote, L. Depression and prognosis following hospital admission because of acute myocardial infarction. CMAJ 2003, 168, 547–552. [Google Scholar]
- Gottlieb, S.S.; Khatta, M.; Friedmann, E.; Einbinder, L.; Katzen, S.; Baker, B.; Marshall, J.; Minshall, S.; Robinson, S.; Fisher, M.L.; et al. The influence of age, gender, and race on the prevalence of depression in heart failure patients. J. Am. Coll. Cardiol. 2004, 43, 1542–1549. [Google Scholar] [CrossRef] [Green Version]
- Rutledge, T.; Reis, V.A.; Linke, S.E.; Greenberg, B.H.; Mills, P.J. Depression in heart failure a meta-analytic review of prevalence, intervention effects, and associations with clinical outcomes. J. Am. Coll. Cardiol. 2006, 48, 1527–1537. [Google Scholar] [CrossRef] [PubMed] [Green Version]
- Pasco, J.A.; Williams, L.J.; Jacka, F.N.; Ng, F.; Henry, M.J.; Nicholson, G.C.; Kotowicz, M.A.; Berk, M. Tobacco smoking as a risk factor for major depressive disorder: Population-based study. Br. J. Psychiatry 2008, 193, 322–326. [Google Scholar] [CrossRef] [PubMed]
- Capuron, L.; Lasselin, J.; Castanon, N. Role of adiposity-driven inflammation in depressive morbidity. Neuropsychopharmacology 2017, 42, 115–128. [Google Scholar] [CrossRef] [PubMed] [Green Version]
- Pan, A.; Keum, N.; Okereke, O.I.; Sun, Q.; Kivimaki, M.; Rubin, R.R.; Hu, F.B. Bidirectional association between depression and metabolic syndrome: A systematic review and meta-analysis of epidemiological studies. Diabetes Care 2012, 35, 1171–1180. [Google Scholar] [CrossRef] [PubMed] [Green Version]
- Rajan, T.M.; Menon, V. Psychiatric disorders and obesity: A review of association studies. J. Postgrad. Med. 2017, 63, 182–190. [Google Scholar] [PubMed]
- Nabi, H.; Bochud, M.; Glaus, J.; Lasserre, A.M.; Waeber, G.; Vollenweider, P.; Preisig, M. Association of serum homocysteine with major depressive disorder: Results from a large population-based study. Psychoneuroendocrinology 2013, 38, 2309–2318. [Google Scholar] [CrossRef] [Green Version]
- Van Agtmaal, M.J.M.; Houben, A.; Pouwer, F.; Stehouwer, C.D.A.; Schram, M.T. Association of microvascular dysfunction with late-life depression: A systematic review and meta-analysis. JAMA Psychiatry 2017, 74, 729–739. [Google Scholar] [CrossRef]
- Salo, K.I.; Scharfen, J.; Wilden, I.D.; Schubotz, R.I.; Holling, H. Confining the concept of vascular depression to late-onset depression: A meta-analysis of mri-defined hyperintensity burden in major depressive disorder and bipolar disorder. Front. Psychol. 2019, 10, 1241. [Google Scholar] [CrossRef] [Green Version]
- Paneni, F.; Beckman, J.A.; Creager, M.A.; Cosentino, F. Diabetes and vascular disease: Pathophysiology, clinical consequences, and medical therapy: Part i. Eur. Heart. J. 2013, 34, 2436–2443. [Google Scholar] [CrossRef]
- Schmidt, A.M.; Yan, S.D.; Wautier, J.L.; Stern, D. Activation of receptor for advanced glycation end products: A mechanism for chronic vascular dysfunction in diabetic vasculopathy and atherosclerosis. Circ. Res. 1999, 84, 489–497. [Google Scholar] [CrossRef] [Green Version]
- Vistoli, G.; De Maddis, D.; Cipak, A.; Zarkovic, N.; Carini, M.; Aldini, G. Advanced glycoxidation and lipoxidation end products (ages and ales): An overview of their mechanisms of formation. Free Radic. Res. 2013, 47 (Suppl. 1), 3–27. [Google Scholar] [CrossRef] [PubMed] [Green Version]
- Guo, Z.J.; Niu, H.X.; Hou, F.F.; Zhang, L.; Fu, N.; Nagai, R.; Lu, X.; Chen, B.H.; Shan, Y.X.; Tian, J.W.; et al. Advanced oxidation protein products activate vascular endothelial cells via a rage-mediated signaling pathway. Antioxid. Redox Signal. 2008, 10, 1699–1712. [Google Scholar] [CrossRef] [PubMed]
- Lee, J.Y.; Sohn, K.H.; Rhee, S.H.; Hwang, D. Saturated fatty acids, but not unsaturated fatty acids, induce the expression of cyclooxygenase-2 mediated through toll-like receptor 4. J. Biol. Chem. 2001, 276, 16683–16689. [Google Scholar] [CrossRef] [PubMed] [Green Version]
- Dhananjayan, R.; Koundinya, K.S.; Malati, T.; Kutala, V.K. Endothelial dysfunction in type 2 diabetes mellitus. Indian J. Clin. Biochem. 2016, 31, 372–379. [Google Scholar] [CrossRef] [PubMed] [Green Version]
- Van Harten, B.; De Leeuw, F.E.; Weinstein, H.C.; Scheltens, P.; Biessels, G.J. Brain imaging in patients with diabetes: A systematic review. Diabetes Care 2006, 29, 2539–2548. [Google Scholar] [CrossRef] [Green Version]
- Williams, K.J.; Wu, X. Imbalanced insulin action in chronic over nutrition: Clinical harm, molecular mechanisms, and a way forward. Atherosclerosis 2016, 247, 225–282. [Google Scholar] [CrossRef] [PubMed] [Green Version]
- Onyike, C.U.; Crum, R.M.; Lee, H.B.; Lyketsos, C.G.; Eaton, W.W. Is obesity associated with major depression? Results from the third national health and nutrition examination survey. Am. J. Epidemiol. 2003, 158, 1139–1147. [Google Scholar] [CrossRef]
- De Wit, L.; Luppino, F.; Van Straten, A.; Penninx, B.; Zitman, F.; Cuijpers, P. Depression and obesity: A meta-analysis of community-based studies. Psychiatry Res. 2010, 178, 230–235. [Google Scholar] [CrossRef]
- Li, L.; Gower, B.A.; Shelton, R.C.; Wu, X. Gender-specific relationship between obesity and major depression. Front. Endocrinol. (Lausanne) 2017, 8, 292. [Google Scholar] [CrossRef] [Green Version]
- Xue, B.; Yu, Y.; Zhang, Z.; Guo, F.; Beltz, T.G.; Thunhorst, R.L.; Felder, R.B.; Johnson, A.K. Leptin mediates high-fat diet sensitization of angiotensin ii-elicited hypertension by upregulating the brain renin-angiotensin system and inflammation. Hypertension 2016, 67, 970–976. [Google Scholar] [CrossRef] [Green Version]
- Perez-Cornago, A.; De la Iglesia, R.; Lopez-Legarrea, P.; Abete, I.; Navas-Carretero, S.; Lacunza, C.I.; Lahortiga, F.; Martinez-Gonzalez, M.A.; Martinez, J.A.; Zulet, M.A. A decline in inflammation is associated with less depressive symptoms after a dietary intervention in metabolic syndrome patients: A longitudinal study. Nutr. J. 2014, 13, 36. [Google Scholar] [CrossRef] [PubMed] [Green Version]
- Briones, A.M.; Nguyen Dinh Cat, A.; Callera, G.E.; Yogi, A.; Burger, D.; He, Y.; Correa, J.W.; Gagnon, A.M.; Gomez-Sanchez, C.E.; Gomez-Sanchez, E.P.; et al. Adipocytes produce aldosterone through calcineurin-dependent signaling pathways: Implications in diabetes mellitus-associated obesity and vascular dysfunction. Hypertension 2012, 59, 1069–1078. [Google Scholar] [CrossRef] [PubMed]
- Huby, A.C.; Otvos, L., Jr.; Belin de Chantemele, E.J. Leptin induces hypertension and endothelial dysfunction via aldosterone-dependent mechanisms in obese female mice. Hypertension 2016, 67, 1020–1028. [Google Scholar] [CrossRef] [PubMed] [Green Version]
- Faulkner, J.L.; Belin de Chantemele, E.J. Sex differences in mechanisms of hypertension associated with obesity. Hypertension 2018, 71, 15–21. [Google Scholar] [CrossRef]
- Goodfriend, T.L.; Kelley, D.E.; Goodpaster, B.H.; Winters, S.J. Visceral obesity and insulin resistance are associated with plasma aldosterone levels in women. Obes. Res. 1999, 7, 355–362. [Google Scholar] [CrossRef]
- Murck, H.; Held, K.; Ziegenbein, M.; Kunzel, H.; Koch, K.; Steiger, A. The renin-angiotensin-aldosterone system in patients with depression compared to controls—A sleep endocrine study. BMC Psychiatry 2003, 3, 15. [Google Scholar] [CrossRef] [Green Version]
- Emanuele, E.; Geroldi, D.; Minoretti, P.; Coen, E.; Politi, P. Increased plasma aldosterone in patients with clinical depression. Arch. Med. Res. 2005, 36, 544–548. [Google Scholar] [CrossRef]
- O’Brien, P.M.; Craven, D.; Selby, C.; Symonds, E.M. Treatment of premenstrual syndrome by spironolactone. Br. J. Obstet. Gynaecol. 1979, 86, 142–147. [Google Scholar] [CrossRef]
- Rosenfeld, R.; Livne, D.; Nevo, O.; Dayan, L.; Milloul, V.; Lavi, S.; Jacob, G. Hormonal and volume dysregulation in women with premenstrual syndrome. Hypertension 2008, 51, 1225–1230. [Google Scholar] [CrossRef] [Green Version]
- Wang, M.; Hammarback, S.; Lindhe, B.A.; Backstrom, T. Treatment of premenstrual syndrome by spironolactone: A double-blind, placebo-controlled study. Acta Obstet. Gynecol. Scand. 1995, 74, 803–808. [Google Scholar] [CrossRef]
- Farquharson, C.A.; Struthers, A.D. Aldosterone induces acute endothelial dysfunction in vivo in humans: Evidence for an aldosterone-induced vasculopathy. Clin. Sci. (Lond.) 2002, 103, 425–431. [Google Scholar] [CrossRef] [PubMed] [Green Version]
- Schafer, N.; Lohmann, C.; Winnik, S.; Van Tits, L.J.; Miranda, M.X.; Vergopoulos, A.; Ruschitzka, F.; Nussberger, J.; Berger, S.; Luscher, T.F.; et al. Endothelial mineralocorticoid receptor activation mediates endothelial dysfunction in diet-induced obesity. Eur. Heart J. 2013, 34, 3515–3524. [Google Scholar] [CrossRef] [PubMed] [Green Version]
- Dinh, Q.N.; Young, M.J.; Evans, M.A.; Drummond, G.R.; Sobey, C.G.; Chrissobolis, S. Aldosterone-induced oxidative stress and inflammation in the brain are mediated by the endothelial cell mineralocorticoid receptor. Brain. Res. 2016, 1637, 146–153. [Google Scholar] [CrossRef] [PubMed]
- Hashikabe, Y.; Suzuki, K.; Jojima, T.; Uchida, K.; Hattori, Y. Aldosterone impairs vascular endothelial cell function. J. Cardiovasc. Pharmacol. 2006, 47, 609–613. [Google Scholar] [CrossRef] [PubMed]
- Krug, A.W.; Kopprasch, S.; Ziegler, C.G.; Dippong, S.; Catar, R.A.; Bornstein, S.R.; Morawietz, H.; Gekle, M. Aldosterone rapidly induces leukocyte adhesion to endothelial cells: A new link between aldosterone and arteriosclerosis? Hypertension 2007, 50, e156–e157. [Google Scholar] [CrossRef] [PubMed] [Green Version]
- Caprio, M.; Newfell, B.G.; La Sala, A.; Baur, W.; Fabbri, A.; Rosano, G.; Mendelsohn, M.E.; Jaffe, I.Z. Functional mineralocorticoid receptors in human vascular endothelial cells regulate intercellular adhesion molecule-1 expression and promote leukocyte adhesion. Circ. Res. 2008, 102, 1359–1367. [Google Scholar] [CrossRef] [PubMed] [Green Version]
- Marzolla, V.; Armani, A.; Mammi, C.; Moss, M.E.; Pagliarini, V.; Pontecorvo, L.; Antelmi, A.; Fabbri, A.; Rosano, G.; Jaffe, I.Z.; et al. Essential role of icam-1 in aldosterone-induced atherosclerosis. Int. J. Cardiol. 2017, 232, 233–242. [Google Scholar] [CrossRef] [Green Version]
- Liu, G.; Yin, G.S.; Tang, J.Y.; Ma, D.J.; Ru, J.; Huang, X.H. Endothelial dysfunction in patients with primary aldosteronism: A biomarker of target organ damage. J. Hum. Hypertens 2014, 28, 711–715. [Google Scholar] [CrossRef]
- Faulkner, J.L.; Kennard, S.; Huby, A.C.; Antonova, G.; Lu, Q.; Jaffe, I.Z.; Patel, V.S.; Fulton, D.J.R.; Belin de Chantemele, E.J. Progesterone predisposes females to obesity-associated leptin-mediated endothelial dysfunction via upregulating endothelial mr (mineralocorticoid receptor) expression. Hypertension 2019, 74, 678–686. [Google Scholar] [CrossRef]
- Kawarazaki, W.; Fujita, T. The role of aldosterone in obesity-related hypertension. Am. J. Hypertens 2016, 29, 415–423. [Google Scholar] [CrossRef] [Green Version]
- Goodfriend, T.L.; Ball, D.L.; Egan, B.M.; Campbell, W.B.; Nithipatikom, K. Epoxy-keto derivative of linoleic acid stimulates aldosterone secretion. Hypertension 2004, 43, 358–363. [Google Scholar] [CrossRef] [PubMed] [Green Version]
- Lopez-Garcia, E.; Schulze, M.B.; Manson, J.E.; Meigs, J.B.; Albert, C.M.; Rifai, N.; Willett, W.C.; Hu, F.B. Consumption of (n-3) fatty acids is related to plasma biomarkers of inflammation and endothelial activation in women. J. Nutr. 2004, 134, 1806–1811. [Google Scholar] [CrossRef] [PubMed] [Green Version]
- Lucas, M.; Mirzaei, F.; O’Reilly, E.J.; Pan, A.; Willett, W.C.; Kawachi, I.; Koenen, K.; Ascherio, A. Dietary intake of n-3 and n-6 fatty acids and the risk of clinical depression in women: A 10-y prospective follow-up study. Am. J. Clin. Nutr. 2011, 93, 1337–1343. [Google Scholar] [CrossRef] [PubMed] [Green Version]
- Ramsden, C.E.; Domenichiello, A.F.; Yuan, Z.X.; Sapio, M.R.; Keyes, G.S.; Mishra, S.K.; Gross, J.R.; Majchrzak-Hong, S.; Zamora, D.; Horowitz, M.S.; et al. A systems approach for discovering linoleic acid derivatives that potentially mediate pain and itch. Sci. Signal 2017, 10, eaal5241. [Google Scholar] [CrossRef] [Green Version]
- Van de Kerkhof, P.C. Plasma aldosterone and cortisol levels in psoriasis and atopic dermatitis. Br. J. Dermatol. 1982, 106, 423–427. [Google Scholar] [CrossRef]
- Patel, R.V.; Shelling, M.L.; Prodanovich, S.; Federman, D.G.; Kirsner, R.S. Psoriasis and vascular disease-risk factors and outcomes: A systematic review of the literature. J. Gen. Intern. Med. 2011, 26, 1036–1049. [Google Scholar] [CrossRef] [Green Version]
- Takeshita, J.; Wang, S.; Shin, D.B.; Mehta, N.N.; Kimmel, S.E.; Margolis, D.J.; Troxel, A.B.; Gelfand, J.M. Effect of psoriasis severity on hypertension control: A population-based study in the united kingdom. JAMA Dermatol. 2015, 151, 161–169. [Google Scholar] [CrossRef]
- Esposito, M.; Saraceno, R.; Giunta, A.; Maccarone, M.; Chimenti, S. An italian study on psoriasis and depression. Dermatology 2006, 212, 123–127. [Google Scholar] [CrossRef]
- Hennebelle, M.; Otoki, Y.; Yang, J.; Hammock, B.D.; Levitt, A.J.; Taha, A.Y.; Swardfager, W. Altered soluble epoxide hydrolase-derived oxylipins in patients with seasonal major depression: An exploratory study. Psychiatry Res. 2017, 252, 94–101. [Google Scholar] [CrossRef]
- Alexopoulos, G.S.; Meyers, B.S.; Young, R.C.; Campbell, S.; Silbersweig, D.; Charlson, M. ’Vascular depression’ hypothesis. Arch. Gen. Psychiatry 1997, 54, 915–922. [Google Scholar] [CrossRef]
- Musselman, D.L.; Evans, D.L.; Nemeroff, C.B. The relationship of depression to cardiovascular disease: Epidemiology, biology, and treatment. Arch. Gen. Psychiatry 1998, 55, 580–592. [Google Scholar] [CrossRef] [PubMed]
- Lavretsky, H.; Zheng, L.; Weiner, M.W.; Mungas, D.; Reed, B.; Kramer, J.H.; Jagust, W.; Chui, H.; Mack, W.J. The mri brain correlates of depressed mood, anhedonia, apathy, and anergia in older adults with and without cognitive impairment or dementia. Int. J. Geriatr. Psychiatry 2008, 23, 1040–1050. [Google Scholar] [CrossRef] [PubMed]
- Baldwin, R.C.; O’Brien, J. Vascular basis of late-onset depressive disorder. Br. J. Psychiatry 2002, 180, 157–160. [Google Scholar] [CrossRef] [Green Version]
- Messner, B.; Bernhard, D. Smoking and cardiovascular disease: Mechanisms of endothelial dysfunction and early atherogenesis. Arterioscler. Thromb. Vasc. Biol. 2014, 34, 509–515. [Google Scholar] [CrossRef] [PubMed] [Green Version]
- Esse, R.; Barroso, M.; Tavares de Almeida, I.; Castro, R. The contribution of homocysteine metabolism disruption to endothelial dysfunction: State-of-the-art. Int. J. Mol. Sci. 2019, 20, 867. [Google Scholar] [CrossRef] [PubMed] [Green Version]
- Dimitrova, K.R.; DeGroot, K.; Myers, A.K.; Kim, Y.D. Estrogen and homocysteine. Cardiovasc. Res. 2002, 53, 577–588. [Google Scholar] [CrossRef] [Green Version]
- Erickson, M.A.; Banks, W.A. Neuroimmune axes of the blood-brain barriers and blood-brain interfaces: Bases for physiological regulation, disease states, and pharmacological interventions. Pharmacol. Rev. 2018, 70, 278–314. [Google Scholar] [CrossRef] [PubMed]
- Capuron, L.; Miller, A.H. Immune system to brain signaling: Neuropsychopharmacological implications. Pharmacol. Ther. 2011, 130, 226–238. [Google Scholar] [CrossRef] [Green Version]
- Rajagopalan, S.; Brook, R.; Rubenfire, M.; Pitt, E.; Young, E.; Pitt, B. Abnormal brachial artery flow-mediated vasodilation in young adults with major depression. Am. J. Cardiol. 2001, 88, 196–198. [Google Scholar] [CrossRef]
- Rybakowski, J.K.; Wykretowicz, A.; Heymann-Szlachcinska, A.; Wysocki, H. Impairment of endothelial function in unipolar and bipolar depression. Biol. Psychiatry 2006, 60, 889–891. [Google Scholar] [CrossRef]
- Shi, H.; Feng, G.; Wang, Z.; Zhou, C.; Zhong, G.; Hu, Y.; Wang, G. Relationships between depressive symptoms and endothelial function among outpatients of a general hospital in china. Med. Sci. Monit. 2015, 21, 1812–1819. [Google Scholar] [PubMed] [Green Version]
- Van Dooren, F.E.; Schram, M.T.; Schalkwijk, C.G.; Stehouwer, C.D.; Henry, R.M.; Dagnelie, P.C.; Schaper, N.C.; Van der Kallen, C.J.; Koster, A.; Sep, S.J.; et al. Associations of low grade inflammation and endothelial dysfunction with depression—The maastricht study. Brain. Behav. Immun. 2016, 56, 390–396. [Google Scholar] [CrossRef] [PubMed] [Green Version]
- Gimbrone, M.A., Jr.; Garcia-Cardena, G. Endothelial cell dysfunction and the pathobiology of atherosclerosis. Circ. Res. 2016, 118, 620–636. [Google Scholar] [CrossRef] [PubMed] [Green Version]
- Tomaschitz, A.; Pilz, S.; Ritz, E.; Grammer, T.; Amrein, K.; Merger, S.; Meinitzer, A.; Winkelmann, B.R.; Boehm, B.O.; Marz, W. Relationship between plasma aldosterone concentration and soluble cellular adhesion molecules in patients referred to coronary angiography. Exp. Clin. Endocrinol. Diabetes 2011, 119, 649–655. [Google Scholar] [CrossRef]
- Al-Qaisi, M.; Kharbanda, R.K.; Mittal, T.K.; Donald, A.E. Measurement of endothelial function and its clinical utility for cardiovascular risk. Vasc. Health Risk Manag. 2008, 4, 647–652. [Google Scholar] [CrossRef] [Green Version]
- Onkelinx, S.; Cornelissen, V.; Goetschalckx, K.; Thomaes, T.; Verhamme, P.; Vanhees, L. Reproducibility of different methods to measure the endothelial function. Vasc. Med. 2012, 17, 79–84. [Google Scholar] [CrossRef]
- Cooper, D.C.; Tomfohr, L.M.; Milic, M.S.; Natarajan, L.; Bardwell, W.A.; Ziegler, M.G.; Dimsdale, J.E. Depressed mood and flow-mediated dilation: A systematic review and meta-analysis. Psychosom. Med. 2011, 73, 360–369. [Google Scholar] [CrossRef] [Green Version]
- Chrapko, W.E.; Jurasz, P.; Radomski, M.W.; Lara, N.; Archer, S.L.; Le Melledo, J.M. Decreased platelet nitric oxide synthase activity and plasma nitric oxide metabolites in major depressive disorder. Biol. Psychiatry 2004, 56, 129–134. [Google Scholar] [CrossRef]
- Garcia, R.G.; Zarruk, J.G.; Barrera, C.; Pinzon, A.; Trillos, E.; Arenas, W.D.; Luengas, C.; Tomaz, C.; Lopez-Jaramillo, P. Plasma nitrate levels and flow-mediated vasodilation in untreated major depression. Psychosom. Med. 2011, 73, 344–349. [Google Scholar] [CrossRef]
- Greaney, J.L.; Saunders, E.F.H.; Santhanam, L.; Alexander, L.M. Oxidative stress contributes to microvascular endothelial dysfunction in men and women with major depressive disorder. Circ. Res. 2019, 124, 564–574. [Google Scholar] [CrossRef]
- Ledebur, H.C.; Parks, T.P. Transcriptional regulation of the intercellular adhesion molecule-1 gene by inflammatory cytokines in human endothelial cells. Essential roles of a variant nf-kappa b site and p65 homodimers. J. Biol. Chem. 1995, 270, 933–943. [Google Scholar] [CrossRef] [PubMed] [Green Version]
- Kawanami, D.; Maemura, K.; Takeda, N.; Harada, T.; Nojiri, T.; Saito, T.; Manabe, I.; Imai, Y.; Nagai, R. C-reactive protein induces vcam-1 gene expression through nf-kappab activation in vascular endothelial cells. Atherosclerosis 2006, 185, 39–46. [Google Scholar] [CrossRef] [PubMed]
- Zapolska-Downar, D.; Siennicka, A.; Kaczmarczyk, M.; Kolodziej, B.; Naruszewicz, M. Butyrate inhibits cytokine-induced vcam-1 and icam-1 expression in cultured endothelial cells: The role of nf-kappab and pparalpha. J. Nutr. Biochem. 2004, 15, 220–228. [Google Scholar] [CrossRef]
- Milstone, D.S.; Ilyama, M.; Chen, M.; O’Donnell, P.; Davis, V.M.; Plutzky, J.; Brown, J.D.; Haldar, S.M.; Siu, A.; Lau, A.C.; et al. Differential role of an nf-kappab transcriptional response element in endothelial versus intimal cell vcam-1 expression. Circ. Res. 2015, 117, 166–177. [Google Scholar] [CrossRef] [PubMed]
- Schmal, H.; Czermak, B.J.; Lentsch, A.B.; Bless, N.M.; Beck-Schimmer, B.; Friedl, H.P.; Ward, P.A. Soluble icam-1 activates lung macrophages and enhances lung injury. J. Immunol. 1998, 161, 3685–3693. [Google Scholar]
- Lawson, C.; Wolf, S. Icam-1 signaling in endothelial cells. Pharmacol. Rep. 2009, 61, 22–32. [Google Scholar] [CrossRef]
- Peter, K.; Nawroth, P.; Conradt, C.; Nordt, T.; Weiss, T.; Boehme, M.; Wunsch, A.; Allenberg, J.; Kubler, W.; Bode, C. Circulating vascular cell adhesion molecule-1 correlates with the extent of human atherosclerosis in contrast to circulating intercellular adhesion molecule-1, e-selectin, p-selectin, and thrombomodulin. Arterioscler. Thromb. Vasc. Biol. 1997, 17, 505–512. [Google Scholar] [CrossRef]
- De Caterina, R.; Basta, G.; Lazzerini, G.; Dell’Omo, G.; Petrucci, R.; Morale, M.; Carmassi, F.; Pedrinelli, R. Soluble vascular cell adhesion molecule-1 as a biohumoral correlate of atherosclerosis. Arterioscler. Thromb. Vasc. Biol. 1997, 17, 2646–2654. [Google Scholar] [CrossRef]
- Ridker, P.M. Intercellular adhesion molecule (icam-1) and the risks of developing atherosclerotic disease. Eur. Heart J. 1998, 19, 1119–1121. [Google Scholar]
- Dimopoulos, N.; Piperi, C.; Salonicioti, A.; Mitsonis, C.; Liappas, I.; Lea, R.W.; Kalofoutis, A. Elevation of plasma concentration of adhesion molecules in late-life depression. Int. J. Geriatr. Psychiatry 2006, 21, 965–971. [Google Scholar] [CrossRef]
- Van Sloten, T.T.; Schram, M.T.; Adriaanse, M.C.; Dekker, J.M.; Nijpels, G.; Teerlink, T.; Scheffer, P.G.; Pouwer, F.; Schalkwijk, C.G.; Stehouwer, C.D.; et al. Endothelial dysfunction is associated with a greater depressive symptom score in a general elderly population: The hoorn study. Psychol. Med. 2014, 44, 1403–1416. [Google Scholar] [CrossRef] [PubMed]
- Gragnano, F.; Sperlongano, S.; Golia, E.; Natale, F.; Bianchi, R.; Crisci, M.; Fimiani, F.; Pariggiano, I.; Diana, V.; Carbone, A.; et al. The role of von willebrand factor in vascular inflammation: From pathogenesis to targeted therapy. Mediat. Inflamm. 2017, 2017, 5620314. [Google Scholar] [CrossRef] [PubMed]
- Kovacevic, K.D.; Mayer, F.J.; Jilma, B.; Buchtele, N.; Obermayer, G.; Binder, C.J.; Blann, A.D.; Minar, E.; Schillinger, M.; Hoke, M. Von willebrand factor antigen levels predict major adverse cardiovascular events in patients with carotid stenosis of the icaras study. Atherosclerosis 2019, 290, 31–36. [Google Scholar] [CrossRef] [PubMed] [Green Version]
- Bongers, T.N.; De Maat, M.P.; Van Goor, M.L.; Bhagwanbali, V.; Van Vliet, H.H.; Gomez Garcia, E.B.; Dippel, D.W.; Leebeek, F.W. High von willebrand factor levels increase the risk of first ischemic stroke: Influence of adamts13, inflammation, and genetic variability. Stroke 2006, 37, 2672–2677. [Google Scholar] [CrossRef] [PubMed]
- Wieberdink, R.G.; Van Schie, M.C.; Koudstaal, P.J.; Hofman, A.; Witteman, J.C.; De Maat, M.P.; Leebeek, F.W.; Breteler, M.M. High von willebrand factor levels increase the risk of stroke: The rotterdam study. Stroke 2010, 41, 2151–2156. [Google Scholar] [CrossRef] [Green Version]
- Kraft, P.; Drechsler, C.; Gunreben, I.; Nieswandt, B.; Stoll, G.; Heuschmann, P.U.; Kleinschnitz, C. Von willebrand factor regulation in patients with acute and chronic cerebrovascular disease: A pilot, case-control study. PLoS ONE 2014, 9, e99851. [Google Scholar] [CrossRef]
- Thompson, S.G.; Kienast, J.; Pyke, S.D.; Haverkate, F.; Van de Loo, J.C. Hemostatic factors and the risk of myocardial infarction or sudden death in patients with angina pectoris. European concerted action on thrombosis and disabilities angina pectoris study group. N. Engl. J. Med. 1995, 332, 635–641. [Google Scholar] [CrossRef]
- Spiel, A.O.; Gilbert, J.C.; Jilma, B. Von willebrand factor in cardiovascular disease: Focus on acute coronary syndromes. Circulation 2008, 117, 1449–1459. [Google Scholar] [CrossRef] [Green Version]
- Domenici, E.; Wille, D.R.; Tozzi, F.; Prokopenko, I.; Miller, S.; McKeown, A.; Brittain, C.; Rujescu, D.; Giegling, I.; Turck, C.W.; et al. Plasma protein biomarkers for depression and schizophrenia by multi analyte profiling of case-control collections. PLoS ONE 2010, 5, e9166. [Google Scholar] [CrossRef] [Green Version]
- Bot, M.; Chan, M.K.; Jansen, R.; Lamers, F.; Vogelzangs, N.; Steiner, J.; Leweke, F.M.; Rothermundt, M.; Cooper, J.; Bahn, S.; et al. Serum proteomic profiling of major depressive disorder. Transl. Psychiatry 2015, 5, e599. [Google Scholar] [CrossRef] [Green Version]
- Lopez-Vilchez, I.; Diaz-Ricart, M.; Navarro, V.; Torramade, S.; Zamorano-Leon, J.; Lopez-Farre, A.; Galan, A.M.; Gasto, C.; Escolar, G. Endothelial damage in major depression patients is modulated by ssri treatment, as demonstrated by circulating biomarkers and an in vitro cell model. Transl. Psychiatry 2016, 6, e886. [Google Scholar] [CrossRef] [PubMed] [Green Version]
- Paffen, E.; DeMaat, M.P. C-reactive protein in atherosclerosis: A causal factor? Cardiovasc. Res. 2006, 71, 30–39. [Google Scholar] [CrossRef] [PubMed]
- Singh, S.K.; Suresh, M.V.; Voleti, B.; Agrawal, A. The connection between c-reactive protein and atherosclerosis. Ann. Med. 2008, 40, 110–120. [Google Scholar] [CrossRef] [PubMed] [Green Version]
- Badimon, L.; Pena, E.; Arderiu, G.; Padro, T.; Slevin, M.; Vilahur, G.; Chiva-Blanch, G. C-reactive protein in atherothrombosis and angiogenesis. Front. Immunol. 2018, 9, 430. [Google Scholar] [CrossRef] [PubMed]
- Ridker, P.M.; Rifai, N.; Stampfer, M.J.; Hennekens, C.H. Plasma concentration of interleukin-6 and the risk of future myocardial infarction among apparently healthy men. Circulation 2000, 101, 1767–1772. [Google Scholar] [CrossRef] [PubMed] [Green Version]
- Osimo, E.F.; Baxter, L.J.; Lewis, G.; Jones, P.B.; Khandaker, G.M. Prevalence of low-grade inflammation in depression: A systematic review and meta-analysis of crp levels. Psychol. Med. 2019, 49, 1958–1970. [Google Scholar] [CrossRef] [PubMed]
- Chamberlain, S.R.; Cavanagh, J.; De Boer, P.; Mondelli, V.; Jones, D.N.C.; Drevets, W.C.; Cowen, P.J.; Harrison, N.A.; Pointon, L.; Pariante, C.M.; et al. Treatment-resistant depression and peripheral c-reactive protein. Br. J. Psychiatry 2019, 214, 11–19. [Google Scholar] [CrossRef] [Green Version]
- Felger, J.C.; Haroon, E.; Patel, T.A.; Goldsmith, D.R.; Wommack, E.C.; Woolwine, B.J.; Le, N.A.; Feinberg, R.; Tansey, M.G.; Miller, A.H. What does plasma crp tell us about peripheral and central inflammation in depression? Mol. Psychiatry 2018. [Google Scholar] [CrossRef]
- De Berardis, D.; Campanella, D.; Gambi, F.; La Rovere, R.; Carano, A.; Conti, C.M.; Sivestrini, C.; Serroni, N.; Piersanti, D.; Di Giuseppe, B.; et al. The role of c-reactive protein in mood disorders. Int. J. Immunopathol. Pharmacol. 2006, 19, 721–725. [Google Scholar] [CrossRef] [Green Version]
- De Berardis, D.; Fornaro, M.; Orsolini, L.; Iasevoli, F.; Tomasetti, C.; De Bartolomeis, A.; Serroni, N.; De Lauretis, I.; Girinelli, G.; Mazza, M.; et al. Effect of agomelatine treatment on c-reactive protein levels in patients with major depressive disorder: An exploratory study in “real-world”, everyday clinical practice. CNS Spectr. 2017, 22, 342–347. [Google Scholar] [CrossRef]
- Erzen, B.; Sabovic, M.; Sebestjen, M.; Keber, I.; Poredos, P. Interleukin-6 correlates with endothelial dysfunction in young post-myocardial infarction patients. Cardiology 2007, 107, 111–116. [Google Scholar] [CrossRef] [PubMed]
- Hartman, J.; Frishman, W.H. Inflammation and atherosclerosis: A review of the role of interleukin-6 in the development of atherosclerosis and the potential for targeted drug therapy. Cardiol. Rev. 2014, 22, 147–151. [Google Scholar] [CrossRef] [PubMed]
- Howren, M.B.; Lamkin, D.M.; Suls, J. Associations of depression with c-reactive protein, il-1, and il-6: A meta-analysis. Psychosom. Med. 2009, 71, 171–186. [Google Scholar] [CrossRef] [PubMed] [Green Version]
- Haapakoski, R.; Mathieu, J.; Ebmeier, K.P.; Alenius, H.; Kivimaki, M. Cumulative meta-analysis of interleukins 6 and 1beta, tumour necrosis factor alpha and c-reactive protein in patients with major depressive disorder. Brain. Behav. Immun. 2015, 49, 206–215. [Google Scholar] [CrossRef] [Green Version]
- Bruunsgaard, H.; Skinhoj, P.; Pedersen, A.N.; Schroll, M.; Pedersen, B.K. Ageing, tumour necrosis factor-alpha (tnf-alpha) and atherosclerosis. Clin. Exp. Immunol. 2000, 121, 255–260. [Google Scholar] [CrossRef]
- Dowlati, Y.; Herrmann, N.; Swardfager, W.; Liu, H.; Sham, L.; Reim, E.K.; Lanctot, K.L. A meta-analysis of cytokines in major depression. Biol. Psychiatry 2010, 67, 446–457. [Google Scholar] [CrossRef]
- Liu, Y.; Ho, R.C.; Mak, A. Interleukin (il)-6, tumour necrosis factor alpha (tnf-alpha) and soluble interleukin-2 receptors (sil-2r) are elevated in patients with major depressive disorder: A meta-analysis and meta-regression. J. Affect. Disord. 2012, 139, 230–239. [Google Scholar] [CrossRef]
- Lesperance, F.; Frasure-Smith, N.; Theroux, P.; Irwin, M. The association between major depression and levels of soluble intercellular adhesion molecule 1, interleukin-6, and c-reactive protein in patients with recent acute coronary syndromes. Am. J. Psychiatry 2004, 161, 271–277. [Google Scholar] [CrossRef]
- Tchalla, A.E.; Wellenius, G.A.; Sorond, F.A.; Travison, T.G.; Dantoine, T.; Lipsitz, L.A. Elevated circulating vascular cell adhesion molecule-1 (svcam-1) is associated with concurrent depressive symptoms and cerebral white matter hyperintensities in older adults. BMC Geriatr. 2015, 15, 62. [Google Scholar] [CrossRef]
- Muller, N. The role of intercellular adhesion molecule-1 in the pathogenesis of psychiatric disorders. Front. Pharmacol. 2019, 10, 1251. [Google Scholar] [CrossRef]
- Hillaert, M.A.; Lentjes, E.G.; Beygui, F.; Kemperman, H.; Asselbergs, F.W.; Nathoe, H.M.; Agostoni, P.; Voskuil, M.; Ivanes, F.; Jude, B.; et al. Measuring and targeting aldosterone and renin in atherosclerosis-a review of clinical data. Am. Heart. J. 2011, 162, 585–596. [Google Scholar] [CrossRef] [PubMed]
- Zehr, K.R.; Walker, M.K. Omega-3 polyunsaturated fatty acids improve endothelial function in humans at risk for atherosclerosis: A review. Prostaglandins Other Lipid Mediat. 2018, 134, 131–140. [Google Scholar] [CrossRef]
- Calder, P.C. Marine omega-3 fatty acids and inflammatory processes: Effects, mechanisms and clinical relevance. Biochim. Biophys. Acta 2015, 1851, 469–484. [Google Scholar] [CrossRef] [PubMed]
- Schunck, W.H.; Konkel, A.; Fischer, R.; Weylandt, K.H. Therapeutic potential of omega-3 fatty acid-derived epoxyeicosanoids in cardiovascular and inflammatory diseases. Pharmacol. Ther. 2018, 183, 177–204. [Google Scholar] [CrossRef] [PubMed]
- Arterburn, L.M.; Hall, E.B.; Oken, H. Distribution, interconversion, and dose response of n-3 fatty acids in humans. Am. J. Clin. Nutr. 2006, 83, 1467S–1476S. [Google Scholar] [CrossRef] [PubMed]
- Bazinet, R.P.; Laye, S. Polyunsaturated fatty acids and their metabolites in brain function and disease. Nat. Rev. Neurosci. 2014, 15, 771–785. [Google Scholar] [CrossRef] [PubMed]
- Dyall, S.C. Long-chain omega-3 fatty acids and the brain: A review of the independent and shared effects of epa, dpa and dha. Front. Aging Neurosci. 2015, 7, 52. [Google Scholar] [CrossRef] [Green Version]
- Chen, C.T.; Domenichiello, A.F.; Trepanier, M.O.; Liu, Z.; Masoodi, M.; Bazinet, R.P. The low levels of eicosapentaenoic acid in rat brain phospholipids are maintained via multiple redundant mechanisms. J. Lipid Res. 2013, 54, 2410–2422. [Google Scholar] [CrossRef] [Green Version]
- Mozaffarian, D.; Lemaitre, R.N.; King, I.B.; Song, X.; Spiegelman, D.; Sacks, F.M.; Rimm, E.B.; Siscovick, D.S. Circulating long-chain omega-3 fatty acids and incidence of congestive heart failure in older adults: The cardiovascular health study: A cohort study. Ann. Intern. Med. 2011, 155, 160–170. [Google Scholar] [CrossRef]
- Steffen, B.T.; Steffen, L.M.; Tracy, R.; Siscovick, D.; Hanson, N.Q.; Nettleton, J.; Tsai, M.Y. Obesity modifies the association between plasma phospholipid polyunsaturated fatty acids and markers of inflammation: The multi-ethnic study of atherosclerosis. Int. J. Obes. (Lond.) 2012, 36, 797–804. [Google Scholar] [CrossRef] [Green Version]
- Yang, Y.; Lu, N.; Chen, D.; Meng, L.; Zheng, Y.; Hui, R. Effects of n-3 pufa supplementation on plasma soluble adhesion molecules: A meta-analysis of randomized controlled trials. Am. J. Clin. Nutr. 2012, 95, 972–980. [Google Scholar] [CrossRef] [PubMed] [Green Version]
- Fleming, J.A.; Kris-Etherton, P.M. The evidence for alpha-linolenic acid and cardiovascular disease benefits: Comparisons with eicosapentaenoic acid and docosahexaenoic acid. Adv. Nutr. 2014, 5, 863S–876S. [Google Scholar] [CrossRef] [PubMed] [Green Version]
- Martins, J.G. Epa but not dha appears to be responsible for the efficacy of omega-3 long chain polyunsaturated fatty acid supplementation in depression: Evidence from a meta-analysis of randomized controlled trials. J. Am. Coll. Nutr. 2009, 28, 525–542. [Google Scholar] [CrossRef] [PubMed]
- Sublette, M.E.; Ellis, S.P.; Geant, A.L.; Mann, J.J. Meta-analysis of the effects of eicosapentaenoic acid (epa) in clinical trials in depression. J. Clin. Psychiatry 2011, 72, 1577–1584. [Google Scholar] [CrossRef] [PubMed] [Green Version]
- Grosso, G.; Pajak, A.; Marventano, S.; Castellano, S.; Galvano, F.; Bucolo, C.; Drago, F.; Caraci, F. Role of omega-3 fatty acids in the treatment of depressive disorders: A comprehensive meta-analysis of randomized clinical trials. PLoS ONE 2014, 9, e96905. [Google Scholar] [CrossRef] [PubMed] [Green Version]
- Mocking, R.J.; Harmsen, I.; Assies, J.; Koeter, M.W.; Ruhe, H.G.; Schene, A.H. Meta-analysis and meta-regression of omega-3 polyunsaturated fatty acid supplementation for major depressive disorder. Transl. Psychiatry 2016, 6, e756. [Google Scholar] [CrossRef]
- Marangell, L.B.; Martinez, J.M.; Zboyan, H.A.; Kertz, B.; Kim, H.F.; Puryear, L.J. A double-blind, placebo-controlled study of the omega-3 fatty acid docosahexaenoic acid in the treatment of major depression. Am. J. Psychiatry 2003, 160, 996–998. [Google Scholar] [CrossRef]
- Yang, B.; Lin, L.; Bazinet, R.P.; Chien, Y.C.; Chang, J.P.; Satyanarayanan, S.K.; Su, H.; Su, K.P. Clinical efficacy and biological regulations of omega-3 pufa-derived endocannabinoids in major depressive disorder. Psychother. Psychosom. 2019, 88, 215–224. [Google Scholar] [CrossRef]
- Artmann, A.; Petersen, G.; Hellgren, L.I.; Boberg, J.; Skonberg, C.; Nellemann, C.; Hansen, S.H.; Hansen, H.S. Influence of dietary fatty acids on endocannabinoid and n-acylethanolamine levels in rat brain, liver and small intestine. Biochim. Biophys. Acta 2008, 1781, 200–212. [Google Scholar] [CrossRef]
- Steffens, S.; Pacher, P. The activated endocannabinoid system in atherosclerosis: Driving force or protective mechanism? Curr. Drug Targets 2015, 16, 334–341. [Google Scholar] [CrossRef]
- Hind, W.H.; Tufarelli, C.; Neophytou, M.; Anderson, S.I.; England, T.J.; O’Sullivan, S.E. Endocannabinoids modulate human blood-brain barrier permeability in vitro. Br. J. Pharmacol. 2015, 172, 3015–3027. [Google Scholar] [CrossRef] [PubMed] [Green Version]
- Benyo, Z.; Ruisanchez, E.; Leszl-Ishiguro, M.; Sandor, P.; Pacher, P. Endocannabinoids in cerebrovascular regulation. Am. J. Physiol. Heart. Circ. Physiol. 2016, 310, H785–H801. [Google Scholar] [CrossRef] [PubMed] [Green Version]
- Chiurchiu, V.; Battistini, L.; Maccarrone, M. Endocannabinoid signalling in innate and adaptive immunity. Immunology 2015, 144, 352–364. [Google Scholar] [CrossRef] [PubMed]
- Chiurchiu, V.; Leuti, A.; Maccarrone, M. Bioactive lipids and chronic inflammation: Managing the fire within. Front. Immunol. 2018, 9, 38. [Google Scholar] [CrossRef] [Green Version]
- Smaga, I.; Bystrowska, B.; Gawlinski, D.; Przegalinski, E.; Filip, M. The endocannabinoid/endovanilloid system and depression. Curr. Neuropharmacol. 2014, 12, 462–474. [Google Scholar] [CrossRef] [Green Version]
- Balvers, M.G.; Verhoeckx, K.C.; Plastina, P.; Wortelboer, H.M.; Meijerink, J.; Witkamp, R.F. Docosahexaenoic acid and eicosapentaenoic acid are converted by 3t3-l1 adipocytes to n-acyl ethanolamines with anti-inflammatory properties. Biochim. Biophys. Acta 2010, 1801, 1107–1114. [Google Scholar] [CrossRef]
- Meijerink, J.; Balvers, M.; Witkamp, R. N-acyl amines of docosahexaenoic acid and other n-3 polyunsatured fatty acids—From fishy endocannabinoids to potential leads. Br. J. Pharmacol. 2013, 169, 772–783. [Google Scholar] [CrossRef] [Green Version]
- Rouzer, C.A.; Marnett, L.J. Endocannabinoid oxygenation by cyclooxygenases, lipoxygenases, and cytochromes p450: Cross-talk between the eicosanoid and endocannabinoid signaling pathways. Chem. Rev. 2011, 111, 5899–5921. [Google Scholar] [CrossRef]
- Fleming, I. The pharmacology of the cytochrome p450 epoxygenase/soluble epoxide hydrolase axis in the vasculature and cardiovascular disease. Pharmacol. Rev. 2014, 66, 1106–1140. [Google Scholar] [CrossRef] [Green Version]
- McDougle, D.R.; Watson, J.E.; Abdeen, A.A.; Adili, R.; Caputo, M.P.; Krapf, J.E.; Johnson, R.W.; Kilian, K.A.; Holinstat, M.; Das, A. Anti-inflammatory omega-3 endocannabinoid epoxides. Proc. Natl. Acad. Sci. USA 2017, 114, E6034–E6043. [Google Scholar] [CrossRef] [Green Version]
- Jung, F.; Schulz, C.; Blaschke, F.; Muller, D.N.; Mrowietz, C.; Franke, R.P.; Lendlein, A.; Schunck, W.H. Effect of cytochrome p450-dependent epoxyeicosanoids on ristocetin-induced thrombocyte aggregation. Clin. Hemorheol. Microcirc. 2012, 52, 403–416. [Google Scholar] [CrossRef] [PubMed] [Green Version]
- Rajesh, M.; Mukhopadhyay, P.; Batkai, S.; Hasko, G.; Liaudet, L.; Huffman, J.W.; Csiszar, A.; Ungvari, Z.; Mackie, K.; Chatterjee, S.; et al. Cb2-receptor stimulation attenuates tnf-alpha-induced human endothelial cell activation, transendothelial migration of monocytes, and monocyte-endothelial adhesion. Am. J. Physiol. Heart. Circ. Physiol. 2007, 293, H2210–H2218. [Google Scholar] [CrossRef] [PubMed] [Green Version]
- Zhao, Y.; Yuan, Z.; Liu, Y.; Xue, J.; Tian, Y.; Liu, W.; Zhang, W.; Shen, Y.; Xu, W.; Liang, X.; et al. Activation of cannabinoid cb2 receptor ameliorates atherosclerosis associated with suppression of adhesion molecules. J. Cardiovasc. Pharmacol. 2010, 55, 292–298. [Google Scholar] [CrossRef]
- Ramirez, S.H.; Hasko, J.; Skuba, A.; Fan, S.; Dykstra, H.; McCormick, R.; Reichenbach, N.; Krizbai, I.; Mahadevan, A.; Zhang, M.; et al. Activation of cannabinoid receptor 2 attenuates leukocyte-endothelial cell interactions and blood-brain barrier dysfunction under inflammatory conditions. J. Neurosci. 2012, 32, 4004–4016. [Google Scholar] [CrossRef] [PubMed]
- Persidsky, Y.; Fan, S.; Dykstra, H.; Reichenbach, N.L.; Rom, S.; Ramirez, S.H. Activation of cannabinoid type two receptors (cb2) diminish inflammatory responses in macrophages and brain endothelium. J. Neuroimmune Pharmacol. 2015, 10, 302–308. [Google Scholar] [CrossRef] [PubMed] [Green Version]
- Rush, A.J.; Sackeim, H.A.; Marangell, L.B.; George, M.S.; Brannan, S.K.; Davis, S.M.; Lavori, P.; Howland, R.; Kling, M.A.; Rittberg, B.; et al. Effects of 12 months of vagus nerve stimulation in treatment-resistant depression: A naturalistic study. Biol. Psychiatry 2005, 58, 355–363. [Google Scholar] [CrossRef] [Green Version]
- Bajbouj, M.; Merkl, A.; Schlaepfer, T.E.; Frick, C.; Zobel, A.; Maier, W.; O’Keane, V.; Corcoran, C.; Adolfsson, R.; Trimble, M.; et al. Two-year outcome of vagus nerve stimulation in treatment-resistant depression. J. Clin. Psychopharmacol. 2010, 30, 273–281. [Google Scholar] [CrossRef] [Green Version]
- Kraus, C.; Kadriu, B.; Lanzenberger, R.; Zarate, C.A., Jr.; Kasper, S. Prognosis and improved outcomes in major depression: A review. Transl. Psychiatry 2019, 9, 127. [Google Scholar] [CrossRef] [Green Version]
- Wang, H.; Yu, M.; Ochani, M.; Amella, C.A.; Tanovic, M.; Susarla, S.; Li, J.H.; Wang, H.; Yang, H.; Ulloa, L.; et al. Nicotinic acetylcholine receptor alpha7 subunit is an essential regulator of inflammation. Nature 2003, 421, 384–388. [Google Scholar] [CrossRef]
- Tracey, K.J. Reflex control of immunity. Nat. Rev. Immunol. 2009, 9, 418–428. [Google Scholar] [CrossRef] [Green Version]
- Howland, R.H. Vagus nerve stimulation. Curr. Behav. Neurosci. Rep. 2014, 1, 64–73. [Google Scholar] [CrossRef] [PubMed] [Green Version]
- Saeed, R.W.; Varma, S.; Peng-Nemeroff, T.; Sherry, B.; Balakhaneh, D.; Huston, J.; Tracey, K.J.; Al-Abed, Y.; Metz, C.N. Cholinergic stimulation blocks endothelial cell activation and leukocyte recruitment during inflammation. J. Exp. Med. 2005, 201, 1113–1123. [Google Scholar] [CrossRef] [Green Version]
- Cooke, J.P.; Ghebremariam, Y.T. Endothelial nicotinic acetylcholine receptors and angiogenesis. Trends Cardiovasc. Med. 2008, 18, 247–253. [Google Scholar] [CrossRef] [PubMed] [Green Version]
- De Jonge, W.J.; Van der Zanden, E.P.; The, F.O.; Bijlsma, M.F.; Van Westerloo, D.J.; Bennink, R.J.; Berthoud, H.R.; Uematsu, S.; Akira, S.; Van den Wijngaard, R.M.; et al. Stimulation of the vagus nerve attenuates macrophage activation by activating the jak2-stat3 signaling pathway. Nat. Immunol. 2005, 6, 844–851. [Google Scholar] [CrossRef] [PubMed]
- Hashimoto, T.; Ichiki, T.; Watanabe, A.; Hurt-Camejo, E.; Michaelsson, E.; Ikeda, J.; Inoue, E.; Matsuura, H.; Tokunou, T.; Kitamoto, S.; et al. Stimulation of alpha7 nicotinic acetylcholine receptor by ar-r17779 suppresses atherosclerosis and aortic aneurysm formation in apolipoprotein e-deficient mice. Vascul. Pharmacol. 2014, 61, 49–55. [Google Scholar] [CrossRef] [PubMed]
- Marrero, M.B.; Lucas, R.; Salet, C.; Hauser, T.A.; Mazurov, A.; Lippiello, P.M.; Bencherif, M. An alpha7 nicotinic acetylcholine receptor-selective agonist reduces weight gain and metabolic changes in a mouse model of diabetes. J. Pharmacol. Exp. Ther. 2010, 332, 173–180. [Google Scholar] [CrossRef] [PubMed] [Green Version]
- Hasan, M.K.; Friedman, T.C.; Sims, C.; Lee, D.L.; Espinoza-Derout, J.; Ume, A.; Chalfant, V.; Lee, M.L.; Sinha-Hikim, I.; Lutfy, K.; et al. Alpha7-nicotinic acetylcholine receptor agonist ameliorates nicotine plus high-fat diet-induced hepatic steatosis in male mice by inhibiting oxidative stress and stimulating ampk signaling. Endocrinology 2018, 159, 931–944. [Google Scholar] [CrossRef]
- Mazloom, R.; Eftekhari, G.; Rahimi-Balaei, M.; Khori, V.; Hajizadeh, S.; Dehpour, A.R.; Mani, A.R. Correction: The role of alpha7 nicotinic acetylcholine receptor in modulation of heart rate dynamics in endotoxemic rats. PLoS ONE 2015, 10, e0127826. [Google Scholar] [CrossRef] [Green Version]
- Tabas, I. Macrophage death and defective inflammation resolution in atherosclerosis. Nat. Rev. Immunol. 2010, 10, 36–46. [Google Scholar] [CrossRef]
- Aoyama, M.; Kotani, J.; Usami, M. Butyrate and propionate induced activated or non-activated neutrophil apoptosis via hdac inhibitor activity but without activating gpr-41/gpr-43 pathways. Nutrition 2010, 26, 653–661. [Google Scholar] [CrossRef]
- Montero-Melendez, T.; Dalli, J.; Perretti, M. Gene expression signature-based approach identifies a pro-resolving mechanism of action for histone deacetylase inhibitors. Cell Death Differ. 2013, 20, 567–575. [Google Scholar] [CrossRef] [PubMed] [Green Version]
- Soliman, G.A. Dietary fiber, atherosclerosis, and cardiovascular disease. Nutrients 2019, 11, 1155. [Google Scholar] [CrossRef] [PubMed] [Green Version]
- Bowes, A.J.; Khan, M.I.; Shi, Y.; Robertson, L.; Werstuck, G.H. Valproate attenuates accelerated atherosclerosis in hyperglycemic apoe-deficient mice: Evidence in support of a role for endoplasmic reticulum stress and glycogen synthase kinase-3 in lesion development and hepatic steatosis. Am. J. Pathol. 2009, 174, 330–342. [Google Scholar] [CrossRef] [Green Version]
- Huang, A.; Young, T.L.; Dang, V.T.; Shi, Y.; McAlpine, C.S.; Werstuck, G.H. 4-phenylbutyrate and valproate treatment attenuates the progression of atherosclerosis and stabilizes existing plaques. Atherosclerosis 2017, 266, 103–112. [Google Scholar] [CrossRef] [PubMed]
- Kim, H.J.; Rowe, M.; Ren, M.; Hong, J.S.; Chen, P.S.; Chuang, D.M. Histone deacetylase inhibitors exhibit anti-inflammatory and neuroprotective effects in a rat permanent ischemic model of stroke: Multiple mechanisms of action. J. Pharmacol. Exp. Ther. 2007, 321, 892–901. [Google Scholar] [CrossRef]
- Leus, N.G.; Zwinderman, M.R.; Dekker, F.J. Histone deacetylase 3 (hdac 3) as emerging drug target in nf-kappab-mediated inflammation. Curr. Opin. Chem. Biol. 2016, 33, 160–168. [Google Scholar] [CrossRef] [Green Version]
- Olesen, J.B.; Abildstrom, S.Z.; Erdal, J.; Gislason, G.H.; Weeke, P.; Andersson, C.; Torp-Pedersen, C.; Hansen, P.R. Effects of epilepsy and selected antiepileptic drugs on risk of myocardial infarction, stroke, and death in patients with or without previous stroke: A nationwide cohort study. Pharmacoepidemiol. Drug Saf. 2011, 20, 964–971. [Google Scholar] [CrossRef]
- Brookes, R.L.; Crichton, S.; Wolfe, C.D.A.; Yi, Q.; Li, L.; Hankey, G.J.; Rothwell, P.M.; Markus, H.S. Sodium valproate, a histone deacetylase inhibitor, is associated with reduced stroke risk after previous ischemic stroke or transient ischemic attack. Stroke 2018, 49, 54–61. [Google Scholar] [CrossRef]
- Olesen, J.B.; Hansen, P.R.; Abildstrom, S.Z.; Andersson, C.; Weeke, P.; Schmiegelow, M.; Erdal, J.; Torp-Pedersen, C.; Gislason, G.H. Valproate attenuates the risk of myocardial infarction in patients with epilepsy: A nationwide cohort study. Pharmacoepidemiol. Drug Saf. 2011, 20, 146–153. [Google Scholar] [CrossRef]
- Davis, L.L.; Kabel, D.; Patel, D.; Choate, A.D.; Foslien-Nash, C.; Gurguis, G.N.; Kramer, G.L.; Petty, F. Valproate as an antidepressant in major depressive disorder. Psychopharmacol. Bull. 1996, 32, 647–652. [Google Scholar]
- Bowden, C.L. Valproate. Bipolar. Disord. 2003, 5, 189–202. [Google Scholar] [CrossRef] [PubMed]
- Smith, L.A.; Cornelius, V.R.; Azorin, J.M.; Perugi, G.; Vieta, E.; Young, A.H.; Bowden, C.L. Valproate for the treatment of acute bipolar depression: Systematic review and meta-analysis. J. Affect. Disord. 2010, 122, 1–9. [Google Scholar] [CrossRef] [PubMed]
- Raison, C.L.; Miller, A.H. Is depression an inflammatory disorder? Curr. Psychiatry Rep. 2011, 13, 467–475. [Google Scholar] [CrossRef] [PubMed] [Green Version]
- Krishnadas, R.; Cavanagh, J. Depression: An inflammatory illness? J. Neurol. Neurosurg. Psychiatry 2012, 83, 495–502. [Google Scholar] [CrossRef] [Green Version]
- Lanquillon, S.; Krieg, J.C.; Bening-Abu-Shach, U.; Vedder, H. Cytokine production and treatment response in major depressive disorder. Neuropsychopharmacology 2000, 22, 370–379. [Google Scholar] [CrossRef]
- O’Brien, S.M.; Scully, P.; Fitzgerald, P.; Scott, L.V.; Dinan, T.G. Plasma cytokine profiles in depressed patients who fail to respond to selective serotonin reuptake inhibitor therapy. J. Psychiatr. Res. 2007, 41, 326–331. [Google Scholar] [CrossRef]
- Tulner, D.M.; Smith, O.R.; Schins, A.; De Jonge, P.; Quere, M.; Delanghe, J.R.; Crijns, H.J.; Den Boer, J.A.; Korf, J.; Honig, A. Antidepressive effect of mirtazapine in post-myocardial infarction depression is associated with soluble tnf-r1 increase: Data from the mind-it. Neuropsychobiology 2011, 63, 169–176. [Google Scholar] [CrossRef] [Green Version]
- Kopschina Feltes, P.; Doorduin, J.; Klein, H.C.; Juarez-Orozco, L.E.; Dierckx, R.A.; Moriguchi-Jeckel, C.M.; De Vries, E.F. Anti-inflammatory treatment for major depressive disorder: Implications for patients with an elevated immune profile and non-responders to standard antidepressant therapy. J. Psychopharmacol. 2017, 31, 1149–1165. [Google Scholar] [CrossRef] [Green Version]
- Rapaport, M.H.; Nierenberg, A.A.; Schettler, P.J.; Kinkead, B.; Cardoos, A.; Walker, R.; Mischoulon, D. Inflammation as a predictive biomarker for response to omega-3 fatty acids in major depressive disorder: A proof-of-concept study. Mol. Psychiatry 2016, 21, 71–79. [Google Scholar] [CrossRef]
Factor | Vascular Inflammation | Major Depressive Disorder |
---|---|---|
CRP | [112,113,114,115] | [116,117,118,119,120] |
IL6 | [115,121,122] | [123,124] |
TNFα | [125] | [126,127] |
sICAM1 | [96,99] | [82,100,101,128,129,130] |
sVCAM1 | [97,98] | [82,100,101,129,130] |
vWF | [103,104,105,106] | [82,109,110,111] |
Aldosterone | [43,58,59,131] | [46,47,49] |
Homocysteine | [75] | [26] |
© 2020 by the author. Licensee MDPI, Basel, Switzerland. This article is an open access article distributed under the terms and conditions of the Creative Commons Attribution (CC BY) license (http://creativecommons.org/licenses/by/4.0/).
Share and Cite
Kalkman, H.O. The Association Between Vascular Inflammation and Depressive Disorder. Causality, Biomarkers and Targeted Treatment. Pharmaceuticals 2020, 13, 92. https://0-doi-org.brum.beds.ac.uk/10.3390/ph13050092
Kalkman HO. The Association Between Vascular Inflammation and Depressive Disorder. Causality, Biomarkers and Targeted Treatment. Pharmaceuticals. 2020; 13(5):92. https://0-doi-org.brum.beds.ac.uk/10.3390/ph13050092
Chicago/Turabian StyleKalkman, Hans O. 2020. "The Association Between Vascular Inflammation and Depressive Disorder. Causality, Biomarkers and Targeted Treatment" Pharmaceuticals 13, no. 5: 92. https://0-doi-org.brum.beds.ac.uk/10.3390/ph13050092